DOI:
10.1039/C6RA16960K
(Paper)
RSC Adv., 2016,
6, 80296-80301
Fidelity quantification of mercury(II) ion via circumventing biothiols-induced sequestration in enzymatic amplification system†
Received
1st July 2016
, Accepted 11th August 2016
First published on 12th August 2016
Abstract
Mercury(II) ion (Hg2+) is a hazardous pollutant with distinct toxicological profiles that can cause deleterious effects on human health and the environment even at low concentrations, so an accurate detection method with high sensitivity is of great importance. Compared with traditional techniques requiring sophisticated instrumentation and skilled personnel, Hg2+ sensors based on nucleic acids amplification outperform others due to their efficiency, robustness, and ease of operation. Among these nucleic acids amplification-based strategies, a common problem meriting attention is that biothiols such as dithiothreitol (DTT) extensively exist in enzymatic amplification systems as essential components to guarantee tool enzyme activity. However, due to the high affinity of Hg2+ and S-donor atoms, biothiols tend to sequestrate Hg2+ and are probably present to affect the accuracy of detection. Herein, a fidelity quantification of Hg2+ based on nucleic acids amplification is developed via a redox reaction or DNA architecture probe. Upon circumventing a biothiols-induced sequestration in enzymatic amplification system, the proposed strategy exhibits excellent accuracy and sensitivity with a detection limit down to 300 pM.
Introduction
Hg2+ is a hazardous pollutant with deleterious effects on human health and the environment even at low concentrations.1,2 So an accurate method with high sensitivity is imperative for its monitoring. Recently, thymine (T)–Hg2+–T coordination is extremely fascinating in the development of Hg2+ sensors due to its strong interaction, which is even more stable than the Watson–Crick adenine (A)–T pair.3,4 Serving as an emerging signal amplification tool, nucleic acids amplification rapidly accumulates sequences of interest and dramatically enhances analytical sensitivity,5–12 Ascribed to its efficiency, robustness, and ease of operation, various nucleic acids amplification-based strategies have been proposed for Hg2+ detection.13–20
Among the methods above, a common problem meriting attention is that biothiols such as DTT and bovine serum albumin extensively exist in enzymatic amplification systems as essential components to guarantee tool enzyme activity.21–24 But in the meantime, biothiols are also utilized to sequestrate Hg2+ through disassembling the T–Hg2+–T bridge due to the high affinity of Hg2+ and S-donor atoms,25–27 thus resulting in a serious interference to the quantification of Hg2+. Thus, the development of highly sensitive and accurate biosensors of Hg2+ based on nucleic acids amplification is still urgently needed.
Experimental section
Reagents and materials
Mercury perchlorate trihydrate (Hg(ClO4)2·3H2O) was purchased from J&K Chemical Ltd. (Beijing, China). NaAc, KAc, CaCl2, NiSO4, Mg(Ac)2, MnSO4, CuCl2, Pb(NO3)2, ZnCl2, CdCl2, FeCl3, AlCl3, Tris(hydroxymethyl)aminomethane (Tris), 3-(N-morpholino)-propanesulfonic acid (MOPS) and other chemicals were ordered from Sinopharm Chemical Reagent Co., Ltd. (Shanghai, China). Klenow Fragment (exo−), Nt.BbvCI and their corresponding buffer were obtained from New England Biolabs Ltd. (Beijing, China). All solutions were prepared and diluted with Milli-Q water (resistance > 18.2 MΩ). All the oligonucleotides were synthesized by Sangon Biotechnology Co., Ltd. (Shanghai, China), and the sequences are as follows:
Primer 1: 5′-TAGAGGTT-3′;
Primer 2: 5′-TTGAGGTA-3′;
Complementary primer: 5′-TAGAGGTA-3′;
Three-way junction (3-WJ) probe 1:
5′-AGAGGTAGTAGGTGATAGTCGG-3′;
3-WJ probe 2: 5′-CCGTCTTTCTCCCCATAT-3′;
3-WJ template: 5′-TGAGGCTAGAGCGAGCTGAGGC
GGATATGGAATACTACCTCTAAA-3′;
Linear template: 5′-CAGTGAGGCTAGAGCGAGCTGA
GGCGGATATGGAATACTACCTCTAAA-3′;
Molecular beacon (MB): 5′-FAM-CCACGAGTGTGCT
GAGGCTAGAGCGTGG-DABCYL-3′;
The bold letters represent the recognition sequence of Nt.BbvCI, and the 5′ end and 3′ end of the MB are labeled with fluorescein (FAM) and quencher (DABCYL), respectively.
Apparatus
The melting curves analyses were performed with LightCycler 96 (Roche Applied Science, Mannheim, Germany). Syngene G:BOX Imaging System (Syngene System, Cambridge, UK) was used to image gel electrophoresis. Fluorescence measurements were carried out on FluoroMax-4 fluorescence spectrometer (Horiba Jobin Yvon, Edison, NJ). Excitation and emission wavelengths were set at 494 nm and 520 nm with the slit widths of 5 nm, respectively. The emission spectra were monitored from 510 nm to 600 nm in steps of 1 nm.
Analysis of samples
The concentration of stock Hg2+ is 10 mM, which was dissolved in 0.5% HNO3, and further diluted to desired concentration with 10 mM MOPS solution (pH = 7.0) containing 0.1 M NaNO3.
Gel electrophoresis and melting curve analysis. The extension reaction was carried out in Tris–Ac buffer (10 mM Tris, 75 mM KAc, 10 mM Mg(Ac)2, pH = 7.9) containing 100 nM primer (primer 1 or primer 2), 100 nM template (3-WJ template), 0.5 units Klenow Fragment, 0.2 × SYBR Green I and 40 μM dNTPs. After addition of Hg2+, the solutions were incubated at 37 °C for 5 min. Then products of the extension reaction were analyzed by agarose gel electrophoresis and melting curve.
Fluorescence measurement. The detection was carried out in a Tris–Ac buffer (10 mM Tris, 75 mM KAc, 10 mM Mg(Ac)2, pH = 7.9) containing 20 nM primer (3-WJ probe 1 & 2 or Primer 1), 20 nM 3-WJ template (or Linear template), 150 nM MB, 0.5 units Klenow Fragment, 4 units Nt.BbvCI and 40 μM dNTPs. After addition of various concentrations of Hg2+, the solutions were incubated at 37 °C for 1 h. Then, fluorescence emission spectra were recorded as described above.
Results and discussion
To circumvent the biothiols-induced sequestration of Hg2+ in polymerase reaction, several studies make compromises through reducing the concentration of DTT in reaction buffers on the basis of commercially available reaction solutions.16,19,28,29 Nevertheless, in stock buffers, tool enzyme is always accompanied by DTT which is unable to be changed or removed. As shown in Fig. 1A, the Hg2+ binding site is denoted as green in primer. Theoretically, induced by the T–Hg2+–T coordination (circled by dash line), primer should offer a complementary 3′ terminal to initiate polymerization. Whereas indeed, due to the sequestration by DTT introduced from polymerase, the signal response is almost the same with blank control. In view of biothiols' reducibility and based on the reported methods, we tried to scavenge DTT by oxidation, which is a more effective approach to avoid the interferences of biothiol in both reaction buffer and tool enzymes' stock buffers at the same time. Hydrogen peroxide (H2O2) was preferentially taken into account for its non-interference in standard polymerase reaction (with complementary primer) at the selected concentration (Fig. S1, ESI†) and the reduction product is also compatible (mechanism of the redox reaction was shown in Fig. S2, ESI†).30,31 As expected, Fig. 1B presents an observable signal response both in gel electrophoresis and melting curve analysis after treatment with H2O2, indicating the interference of DTT could be effectively eliminated. To confirm the roles of H2O2 and DTT in this system, we further added extra DTT into the polymerase reaction recovered by H2O2 and obtained an undetectable signal. Then H2O2 with higher concentration was brought in to consume excess DTT, resulting in a signal restoration again (Fig. 1C). As a kind of common oxidant, the H2O2 involved approach is universal and can be directly applied to various kinds of amplification strategies. Though this proposed strategy achieves a desired result, the involvement of readily degradable H2O2 is likely to lead reproducibility problems with the assay. What's more, excess H2O2 causes side-effects and even inhibits the signal response (Fig. S3, ESI†).
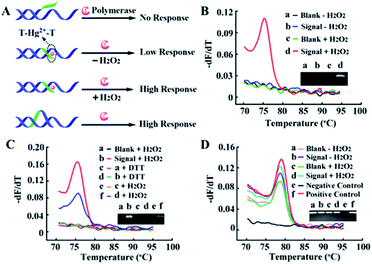 |
| Fig. 1 (A) Illustration of the approaches to circumvent biothiols-induced sequestration of Hg2+ in polymerase reaction. (B) Gel electrophoresis and melting curve analysis of polymerase reaction using duplex probe with Hg2+ binding site at the 3′ terminal of primer in the presence of Hg2+ before (b) and after (d) the addition of H2O2 (0.01%), with corresponding blank controls without Hg2+ (a, c), respectively. (C) The effect of H2O2 and DTT on the polymerase reaction: the signal response of Hg2+ (b) disappears with the addition of DTT (10 μM, d) and can be recovered again after treatment with higher concentration H2O2 (0.02%, f), with corresponding blank controls without Hg2+ (a, c, e), respectively. (D) Gel electrophoresis and melting curve analysis of polymerase reaction using duplex probe with 6 bp complementary region from Hg2+ binding site to the 3′ terminal of primer in the presence of Hg2+ before (b) and after (d) the addition of H2O2 (0.01%), with corresponding blank controls without Hg2+ (a, c), respectively. Negative (e) and positive (f) control represent the polymerase reaction without and with complementary primer. | |
Inspired by the distinct gap between polymerase's exceptional catalytic velocity (at rates near 1000 nucleotides per minute per molecule of enzyme)32 and biothiols' gradual competition process,33–35 we propose another alternative strategy herein. A rational designed DNA architecture probe first initiates extension upon Hg2+ binding, and immediately forms a stable polymerizable structure which is insusceptible to the subsequent Hg2+ release, providing another avenue to avoid the interference of biothiols. To exert a maximum advantage of catalytic rate, we reserved a perfectly complementary region with a length of 6 bp to match the minimal primer length required for polymerase,36 so as to spatially separate the Hg2+ binding domain and polymerase recognition domain. A primer-template duplex probe is not a candidate due to its unwanted background, even though it shows a significant signal response in the presence of DTT (Fig. 1D).
Compared with a duplex probe, a DNA machine assembled by three strands offers an excellent discrimination of even single-base mismatched sequences,37 and thus holds great potential to reduce the system's background amplification. Based on our previous studies about DNA architecture probes and their thermodynamics properties,38,39 we constructed a Hg2+-triggered 3-WJ DNA machine which can circumvent biothiols-induced sequestration in an enzymatic amplification system without introducing any extra interference. As illustrated in Fig. 2A, 3-WJ probes and a 3-WJ template are involved in this proposed sensing strategy. In the absence of Hg2+, no stable 3-WJ DNA machine is generated to initiate the subsequent amplification. Once the Hg2+-triggered 3-WJ DNA machine forms (Hg2+ binding site is circled by a dashed line and highlighted in pink), polymerase immediately starts extension along the 3-WJ template and produces a duplex region containing a sequence-specific nicking site (highlighted in orange) for the nicking endonuclease Nt.BbvCI to initiate a new extension cycle. Ascribed to the tactful design, numerous DNA products are continuously produced upon repeated scission and replication, even if the 3-WJ structure may have been broken up by biothiols. These products can anneal with the MB (hybridization regions are highlighted in blue) and lead to a significant fluorescence signal. On the basis of linear amplification, we introduced a recognition sequence of Nt.BbvCI within the MB, by which the next stage amplification can be coupled and generate more DNA products to open MB (hybridization regions are highlighted in magenta). Eventually, two stage amplification modules are integrated into a quadratic amplification system without adding any extra reaction components or processes. Then we compared the fluorescence responses of different amplification modes in the presence of 20 nM Hg2+. In the linear process, extra 3 bases are added at the 5′ terminal of the quadratic template to block the next stage amplification. As presented in Fig. 2B, the quadratic mode exhibits a signal enhancement of 11.4 fold, whereas only a 4.6-fold signal increase is achieved by the linear mode. To confirm the feasibility of the 3-WJ DNA machine to circumvent the biothiols-induced sequestration of Hg2+, a series of experiments was performed for comparing the signal responses in different systems without the treatment of H2O2. As shown in Fig. 2C, the 3-WJ DNA machine induces an excellent fluorescence signal enhancement, yet no detectable signal is obtained using the duplex probe with a Hg2+ binding site at the 3′ terminal of the primer.
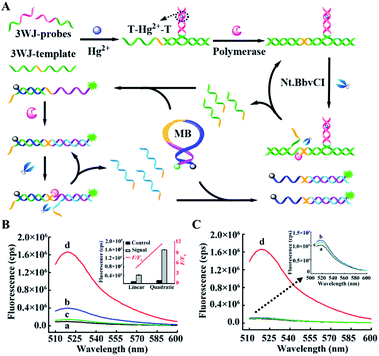 |
| Fig. 2 (A) Illustration of Hg2+ detection based on 3-WJ DNA machine. (B) The fluorescence spectra of the linear (b) and quadratic (d) amplification in the presence of 20 nM Hg2+, with corresponding backgrounds control without Hg2+ (a, c), respectively. Inset: fluorescence responses of linear and quadratic amplification. F0 and F represent the fluorescence intensity in the absence and presence of Hg2+, respectively. (C) Confirmation the feasibility of 3-WJ DNA machine to circumvent the biothiols-induced sequestration of Hg2+. The fluorescence spectra of the duplex probe-mediated (with Hg2+ binding site at the 3′ terminal of primer, b) and 3-WJ DNA machine-mediated (d) quadratic amplification in the presence of 20 nM Hg2+, with corresponding backgrounds control without Hg2+ (a, c), respectively. Inset is the enlargement for fluorescence spectra of (a), (b) and (c). | |
To obtain the best sensing performance, the ionic strength of buffer solution (Fig. S4, ESI†), concentration of MB (Fig. S5, ESI†), Klenow Fragment (Fig. S6, ESI†), and Nt.BbvCI (Fig. S7, ESI†) were investigated at a fixed Hg2+ concentration of 20 nM. As a result, 75 mM KAc, 10 mM Mg (Ac)2, 150 nM MB, 0.5 units Klenow Fragment, and 4 units Nt.BbvCI were selected for the maximum fluorescence enhancement (F/F0). Apart from the aforementioned parameters, the concentration of 3-WJ probes and 3-WJ template, reaction temperature, and time are also critical factors in this sensing strategy. With the reactant concentration increasing, more successful molecular collisions occur and speed up the rate of reaction. As shown in Fig. 3A, with the concentration of 3-WJ probes varying from 5 to 80 nM, the fluorescence intensity gradually increases and reaches a maximum at 20 nM. Thereafter, fluorescence response exhibits a decline with further increase in the concentration of the 3-WJ probes. It is possible that a certain amount of Hg2+ disperses among the excess probes, thus disturbing the formation of a stable 3-WJ DNA machine. As similarly presented in Fig. 3B, the fluorescence response trends to increase with the concentration of 3-WJ templates. However, high concentration templates offer more available locations to anneal amplification products which will compete with MB and reduce the signal responses, so 20 nM 3-WJ probes and 3-WJ template were chosen for the following experiments. An appropriate reaction temperature is not only closely related with the assembly of 3-WJ DNA machine, but also able to ensure the activity of enzyme. With comparison of the F/F0 ratios at four temperatures, 37 °C was selected as the optimum temperature (Fig. 3C). In addition, the effect of the reaction time was also investigated. As depicted in Fig. 3D, the fluorescence intensity of both signal and background increases gradually with the prolongation of the reaction time. The signal intensity nearly approaches a steady state after incubation for 60 min, while background intensity continues to rise. Thus, 60 min was selected as the optimized reaction time.
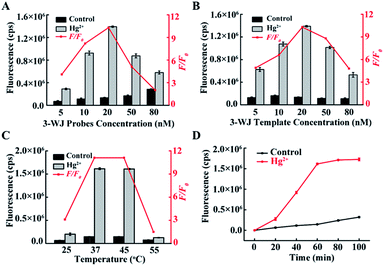 |
| Fig. 3 Optimization of experimental conditions. The effect of the 3-WJ probes concentration (A), 3-WJ template concentration (B), and reaction temperature (C) on the fluorescence responses of proposed strategy. F0 and F represent the fluorescence intensity in the absence and presence of 20 nM Hg2+, respectively. (D) The time-dependent fluorescence response with 20 nM Hg2+ over background fluorescence. The error bars indicate the standard deviations of three independent experiments. | |
Under the optimized detection conditions, various concentrations of Hg2+ (0–40 nM) were introduced into the system to examine whether the proposed strategy could be used for quantification. With increase of the Hg2+ concentration, the fluorescence spectrum appears as an obvious enhancement accordingly (Fig. 4A). The relationship between the fluorescence intensities at 520 nm and the concentrations of Hg2+ is shown in Fig. 4B. As depicted in Fig. 4C, a good linear relationship between the fluorescence intensity and the Hg2+ concentration is obtained in the range from 300 pM to 20 nM, with a regression equation of Y = 0.7368X + 1.4087 and a correlation coefficient of 0.9933, where Y is the fluorescence intensity (×105 cps) and X is the Hg2+ concentration, indicating that the fluorescence sensing strategy is effective for the detection of Hg2+. The limit of detection (taken to be 3 times the standard deviation in the blank solution) is 300 pM, which is lower than the maximum level of Hg2+ in drinking water permitted by the U.S. Environmental Protection Agency (10 nM). To the best of our knowledge, this detection limit of the proposed approach is much lower than those of most existing colorimetric (50–60 nM)40,41 and fluorescent (0.8–2.5 nM)42,43 methods, and is even comparable to some electrochemical (0.2 nM)44 and electrochemiluminescent (2.3 nM)45 sensors.
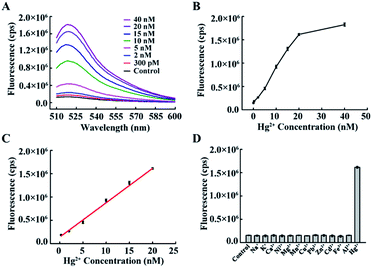 |
| Fig. 4 (A) Fluorescence spectra response to different concentrations of Hg2+. (B) Changes in fluorescence intensity at 520 nm upon the addition of various concentrations of Hg2+. (C) The linear correlation between the fluorescence intensity and the concentration of Hg2+ in the range of 300 pM to 20 nM. (D) Selectivity of the proposed strategy for Hg2+ detection over other common interference metal ions. The concentration of Hg2+ is 20 nM, whereas the concentrations of other metal ions are each 2 μM. The error bars indicate the standard deviations of three independent experiments. | |
The selectivity of the proposed sensing system for Hg2+ was evaluated by investigating the responses of the assay to other environmentally relevant metal ions, including Na+, K+, Ca2+, Ni2+, Mg2+, Mn2+, Cu2+, Pb2+, Zn2+, Cd2+, Fe3+, and Al3+. As shown in Fig. 4D, Hg2+ exhibits a remarkable fluorescence signal response and other interfering metal ions have only negligible effects even at a 100-fold concentration more than Hg2+ (2 μM vs. 20 nM), demonstrating an excellent selectivity of the proposed method over other metal ions.
To validate the capability of the proposed strategy for practical applications, we applied this assay to detect Hg2+ ions in water samples from the Xi'an Chan River and Xi'an Ba River. The collected water was filtered by using a 0.22 μm membrane to remove insoluble substances, and obtained an undetectable signal response of Hg2+, which was confirmed by Atomic Fluorescence Spectrometry (AFS). Then the water samples were spiked with Hg2+ at different concentrations of 5 nM and 10 nM. As listed in Table 1, satisfactory values between 95.6% and 104.6% were obtained for the recovery experiments. Moreover, the artificially contaminated water samples were further certified by AFS, the results of which are in accordance with the proposed method, confirming the accuracy and fidelity of the developed strategy for the quantification of Hg2+ and indicating its great potential for real environmental samples analysis.
Table 1 Results for the determination of Hg2+ in river water samples
Sample |
Hg2+ added (nM) |
Measured (nM) |
Recoverya (%) |
AFS (nM) |
Recoveryb (%) |
The recovery obtained by this proposed method. The recovery obtained by AFS. Mean of three determinations. Standard deviation. |
Xi'an |
5 |
5.23 ± 0.38d |
104.6 |
5.48c ± 0.45d |
109.0 |
Chan river |
10 |
10.35c ± 0.15d |
103.5 |
9.93c ± 0.51d |
99.3 |
Xi'an |
5 |
4.78c ± 0.28d |
95.6 |
5.38c ± 0.34d |
107.6 |
Ba river |
10 |
10.15c ± 0.43d |
101.5 |
10.33c ± 0.31d |
103.3 |
Conclusions
In this work, the sequestration of Hg2+ induced by biothiols in an enzymatic system was discussed. By using a redox reaction or DNA architecture probe, we successfully developed a strategy to circumvent interferences and obtained a fidelity quantification of Hg2+. Through a well-designed MB, we introduced a homogeneous and quadratic amplification strategy and achieved an excellent sensitivity with a detection limit of 300 pM. In addition, the proposed sensing system displayed an extreme specificity towards Hg2+, even in the presence of 100-fold excess of other interfering metal ions due to the specific formation of T–Hg2+–T coordination. Furthermore, the method's accuracy and reliability for the detection of Hg2+ in complex water samples was verified by AFS, demonstrating the sensing strategy has enormous potential in real environmental samples.
Acknowledgements
This research was supported by the Research Project Foundation of Shaanxi Branch of China National Tobacco Corporation (No. 201112029), the National Natural Science Foundation of China (No. 21475102) and “Young Talent Support Plan” of Xi'an Jiaotong University. We would like to thank Engineer Guanghui Qiang at Xi'an Water Group Company Limited (Xi'an, Shaanxi) for supporting the AFS analysis.
Notes and references
- P. M. Bolger and B. Schwetz, N. Engl. J. Med., 2002, 347, 1735–1736 CrossRef PubMed.
- T. W. Clarkson, L. Magos and G. J. Myers, N. Engl. J. Med., 2003, 349, 1731–1737 CrossRef CAS PubMed.
- A. Ono and H. Togashi, Angew. Chem., Int. Ed., 2004, 43, 4300–4302 CrossRef CAS PubMed.
- Y. Miyake, H. Togashi, M. Tashiro, H. Yamaguchi, S. Oda, M. Kudo, Y. Tanaka, Y. Kondo, R. Sawa and T. Fujimoto, J. Am. Chem. Soc., 2006, 128, 2172–2173 CrossRef CAS PubMed.
- Y. Zhao, F. Chen, Q. Li, L. Wang and C. Fan, Chem. Rev., 2015, 115, 12491–12545 CrossRef CAS PubMed.
- Y. Zhao, F. Chen, Q. Zhang, Y. Zhao, X. Zuo and C. Fan, NPG Asia Mater., 2014, 6, e131 CrossRef CAS.
- R. Deng, L. Tang, Q. Tian, Y. Wang, L. Lin and J. Li, Angew. Chem., Int. Ed., 2014, 53, 2389–2393 CrossRef CAS PubMed.
- Z. Ge, M. Lin, P. Wang, H. Pei, J. Yan, J. Shi, Q. Huang, D. He, C. Fan and X. Zuo, Anal. Chem., 2014, 86, 2124–2130 CrossRef CAS PubMed.
- W. Li, Z. Liu, H. Lin, Z. Nie, J. Chen, X. Xu and S. Yao, Anal. Chem., 2010, 82, 1935–1941 CrossRef CAS PubMed.
- X. Zhang, C. Liu, L. Sun, X. Duan and Z. Li, Chem. Sci., 2015, 6, 6213–6218 RSC.
- Y. Zhang, L. Zhang, J. Sun, Y. Liu, X. Ma, S. Cui, L. Ma, J. J. Xi and X. Jiang, Anal. Chem., 2014, 86, 7057–7062 CrossRef CAS PubMed.
- R. Duan, X. Zuo, S. Wang, X. Quan, D. Chen, Z. Chen, L. Jiang, C. Fan and F. Xia, J. Am. Chem. Soc., 2013, 135, 4604–4607 CrossRef CAS PubMed.
- J. Liu, L. Chen, J. Chen, C. Ge, Z. Fang, L. Wang, X. Xing and L. Zeng, Anal. Methods, 2014, 6, 2024–2027 RSC.
- X. Zhou, Q. Su and D. Xing, Anal. Chim. Acta, 2012, 713, 45–49 CrossRef CAS PubMed.
- L. Freage, F. Wang, R. Orbach and I. Willner, Anal. Chem., 2014, 86, 11326–11333 CrossRef CAS PubMed.
- D. Li, A. Wieckowska and I. Willner, Angew. Chem., 2008, 120, 3991–3995 CrossRef.
- H. Jia, Z. Wang, C. Wang, L. Chang and Z. Li, RSC Adv., 2014, 4, 9439–9444 RSC.
- G. Zhu, Y. Li and C.-Y. Zhang, Chem. Commun., 2014, 50, 572–574 RSC.
- X. Zhu, X. Zhou and D. Xing, Biosens. Bioelectron., 2011, 26, 2666–2669 CrossRef CAS PubMed.
- J. Yin, X. He, X. Jia, K. Wang and F. Xu, Analyst, 2013, 138, 2350–2356 RSC.
- W. Cleland, Biochemistry, 1964, 3, 480–482 CrossRef CAS PubMed.
- S. Shah, A. Sharma and M. N. Gupta, Anal. Biochem., 2006, 351, 207–213 CrossRef CAS PubMed.
- M. Nagai, A. Yoshida and N. Sato, IUBMB Life, 1998, 44, 157–163 CrossRef CAS.
- A. Haas and I. Rose, J. Biol. Chem., 1982, 257, 10329–10337 CAS.
- J. M. Thomas, H.-Z. Yu and D. Sen, J. Am. Chem. Soc., 2012, 134, 13738–13748 CrossRef CAS PubMed.
- L. Wang, T. Yao, S. Shi, Y. Cao and W. Sun, Sci. Rep., 2014, 4, 5320 CAS.
- M. Stobiecka, A. A. Molinero, A. Chałupa and M. Hepel, Anal. Chem., 2012, 84, 4970–4978 CrossRef CAS PubMed.
- H. Urata, E. Yamaguchi, T. Funai, Y. Matsumura and S. I. Wada, Angew. Chem., 2010, 122, 6666–6669 CrossRef.
- T. Funai, J. Nakamura, Y. Miyazaki, R. Kiriu, O. Nakagawa, S. I. Wada, A. Ono and H. Urata, Angew. Chem., Int. Ed., 2014, 53, 6624–6627 CrossRef CAS PubMed.
- W. M. Kaiser, Planta, 1979, 145, 377–382 CrossRef CAS PubMed.
- C. C. Winterbourn and D. Metodiewa, Free Radical Biol. Med., 1999, 27, 322–328 CrossRef CAS PubMed.
- A. Kornberg, Science, 1969, 163, 1410–1418 CAS.
- M. Zhang, H.-N. Le, X.-Q. Jiang and B.-C. Ye, Chem. Commun., 2013, 49, 2133–2135 RSC.
- J. Zhao, C. Chen, L. Zhang, J. Jiang, G. Shen and R. Yu, Analyst, 2013, 138, 1713–1718 RSC.
- H. Xu and M. Hepel, Anal. Chem., 2011, 83, 813–819 CrossRef CAS PubMed.
- G. Zhao and Y. Guan, Acta Biochim. Biophys. Sin., 2010, 42, 722–728 CrossRef CAS PubMed.
- Q. Zhang, F. Chen, F. Xu, Y. Zhao and C. Fan, Anal. Chem., 2014, 86, 8098–8105 CrossRef CAS PubMed.
- Y. Zhao, L. Qi, F. Chen, Y. Zhao and C. Fan, Biosens. Bioelectron., 2013, 41, 764–770 CrossRef CAS PubMed.
- F. Chen, C. Fan and Y. Zhao, Anal. Chem., 2015, 87, 8758–8764 CrossRef CAS PubMed.
- G.-H. Chen, W.-Y. Chen, Y.-C. Yen, C.-W. Wang, H.-T. Chang and C.-F. Chen, Anal. Chem., 2014, 86, 6843–6849 CrossRef CAS PubMed.
- J. Wu, L. Li, D. Zhu, P. He, Y. Fang and G. Cheng, Anal. Chim. Acta, 2011, 694, 115–119 CrossRef CAS PubMed.
- H. Xu, X. Zhu, H. Ye, L. Yu, X. Liu and G. Chen, Chem. Commun., 2011, 47, 12158–12160 RSC.
- J. Ma, Y. Chen, Z. Hou, W. Jiang and L. Wang, Biosens. Bioelectron., 2013, 43, 84–87 CrossRef CAS PubMed.
- F. Xuan, X. Luo and I. M. Hsing, Anal. Chem., 2013, 85, 4586–4593 CrossRef CAS PubMed.
- X. Zhu, L. Chen, Z. Lin, B. Qiu and G. Chen, Chem. Commun., 2010, 46, 3149–3151 RSC.
Footnote |
† Electronic supplementary information (ESI) available: Additional figures. See DOI: 10.1039/c6ra16960k |
|
This journal is © The Royal Society of Chemistry 2016 |
Click here to see how this site uses Cookies. View our privacy policy here.