DOI:
10.1039/C6RA18965B
(Paper)
RSC Adv., 2016,
6, 95865-95878
Removal of antimonite (Sb(III)) and antimonate (Sb(V)) using zerovalent iron decorated functionalized carbon nanotubes
Received
26th July 2016
, Accepted 29th September 2016
First published on 30th September 2016
Abstract
Carbon nanotubes (CNT) were synthesized by floating catalytic chemical vapor deposition method using ferrocene and benzene as hydrocarbon source. The prepared CNTs were oxidized with nitric acid (CNT-OX) followed by coating with nano zerovalent iron (ZVI) to yield nano ZVI decorated CNTs (CNT-Fe(0)). The prepared adsorbents were characterized by various techniques. Using spectral techniques like infra red, Raman and X-ray photoelectron spectroscopy, the mechanism of adsorption between the adsorbent and adsorbate has been postulated. Using CNT-Fe(0) at pH 5, the maximum adsorption capacity of Sb(III) and Sb(V) was found to be 250 mg g−1. The high sorption capacity could be attributed to the high surface area of the prepared CNTs and the reactive nature of the nano zerovalent iron particles on CNTs. A pseudo second order kinetics was followed by CNT-Fe(0) with both Sb(III) and Sb(V) with a rate constant of 0.0015 and 0.0011 mg g−1 min−1 respectively. The interference effect of various ions on the adsorption of Sb(III)/Sb(V) are as follows: PO43− > SiO32− > ASO43− > SO42−, NO3− > Cl−, humic acid. The recyclability of the sorbent was demonstrated for 5 cycles using 0.05 M HCl as desorbent. The versatility of the adsorbent was demonstrated by the removal capacity of both Sb(III) and Sb(V) at parts per billion levels from nuclear decontamination formulation (NAC) and tap water matrix as well.
1. Introduction
Antimony (Sb) is widely used in various industries around the world.1 In the environment antimony exists as both Sb(III) and Sb(V) species. It is used in various industrial products such as alloys for ammunition, flame retardants, semiconductors, and catalysts for plastics synthesis.2 Antimony is also used as a hardening agent (2–5% w/w) in the alloy of bullets. Hence it can leach into the soil of shooting ranges from these bullets.3 In oxic systems antimony primarily exists as Sb(V) and in anoxic systems it exists as Sb(III). Due to biological activity and kinetic effects significant amounts of Sb(III) and Sb(V) have been found in oxic and anoxic systems, respectively.1 Further, its use as a structural material in nuclear reactors has also been reported.4 Specifically in the coolant pumps of nuclear reactors, antimony impregnated graphite seals are found to be used. It is reported that, due to routine wear and tear, antimony gets released into the coolant.4 The released antimony gets activated by radiation to active species of antimony (122Sb, 124Sb).5 Though cation exchange columns were effective in removing various other nuclides like cesium, cobalt, it was inefficient in removing antimony as it was present in neutral Sb(III) state.6 A process involving oxidation of Sb(III) to Sb(V) species and its subsequent removal anion exchange resins were also sorted but the removal was not satisfactory due to the competition from hydroxyl ions which were present in excess compared to the low concentration of antimony. Recently, Nishad et al. (2014)4 reported the removal of antimony from decontamination medium, however the removal of Sb(V) was found to be only 32.81%. Thus, there is a need for an adsorbent to remove both species of antimony at low levels in nuclear reactors. Apart from its use in nuclear reactors, antimony and its compounds are classified as priority pollutants by the EU and USEPA and maximum contaminant level in the environment is set at 6.0 μg l−1.7 Though various methods including chemical precipitation, coagulation/flocculation, ion exchange resins, membrane technologies have been used, adsorption is the most resorted technique owing to its cost effectiveness, simplicity in the design and applicability to low levels of antimony as well. Several kinds of adsorbents including graphene,8 montmorillonite, bentonite9 hydrated ferric oxides,10 kaolinite,11 manganite,12 goethite,13 Fe–Zr bimetal oxides,14 Fe–Mn bimetal oxides15 MWCNT,16 ZrO2 doped CNTs17 etc., have been reported. It is to be noted that except a very few adsorbents like goethite, montmorillonite, ZrO2 doped CNTs, all the other adsorbents reported were evaluated for the removal of either Sb(III) or Sb(V) species.
It is well known that Fe(0) has been found useful as an efficient adsorbent for the removal of various contaminants in the environment.18–20 Some of the contaminants include Cr(VI),21,22 uranium,23 selenium,24 etc. However, the challenges that are faced using Fe(0) nano particles are lack of stability, agglomeration, difficulty in recycling due to their small size and reducing specificity in aqueous medium.25,26 To overcome the afore mentioned challenges, embedding of nanoparticles in high surface area materials would lead to environmental solutions.
Thus in this paper, carbon nano tubes were prepared indigenously by floating catalytic chemical vapor deposition method and zerovalent iron was doped on CNTs to obtain CNT-Fe(0). Further addition of EDTA during the preparation of Fe(0) helped in the prevention of agglomeration of Fe(0) nano particles.27 The prepared adsorbent was characterized by both physical and chemical spectroscopic techniques and evaluated for the removal of both Sb(III) and Sb(V). The conditions required for the maximum removal of both the species has been optimized and its applicability has been demonstrated in the Sb(III)/Sb(V) spiked tap water and Sb(III)/Sb(V) spiked nuclear decontaminant solutions. Using FTIR, Raman and X-ray photoelectron spectroscopic techniques mechanism of adsorption is also postulated.
2. Materials and methods
2.1 Materials
Analytical grades of ferrous sulphate, sodium borohydride, EDTA, potassium antimony(III) tartrate hemihydrates (K(SbO)C4H4O6−·0.5H2O) and potassium hexahydroxo antimonate (KSb(OH)6), were acquired from Sigma-Aldrich. All other chemicals and reagents were of AR grade and were acquired from either from Himedia or Merck, India. All the reagents and standards were prepared in Milli-Q water. Sb(III) and Sb(V) solutions were prepared using potassium antimony(III) oxide tartrate and KSb(OH)6−, respectively.
2.2 Adsorbent preparation
CNTs were prepared by floating catalytic horizontal CVD (Chemical Vapor Deposition) method using 2% of ferrocene in benzene as hydrocarbon source. The detailed procedure regarding the preparation is described elsewhere.28 Obtained CNT-plain was oxidized by heating with nitric acid for 80 °C 3 h to obtain CNT-OX. For the preparation of CNT-Fe(0), around 0.3 g of CNT-OX was treated with 100 ml of 0.1 M FeSO4, 50 ml of 0.05 M EDTA and mixed in a three necked round bottomed flask by using propeller mixing. Further, drop wise addition of 0.75 M NaBH4 facilitated the formation of black colored nano zerovalent iron. Finally the Fe(0) coated CNTs were washed, filtered and dried at 100 °C for further use.
2.3 Characterization
SEM images of CNT-plain, CNT-OX and CNT-Fe(0) were obtained with a field emission scanning electron microscope (Carl Zeiss NTS GmbH, Oberkochen (Germany) Model: SUPRA 40VP) operated at an accelerating voltage of 10 kV. Confocal Raman Microscope (Witec, Germany) using a striking wavelength of 514 nm was used for Raman spectra. Antimony concentrations were determined by inductively coupled plasma-mass spectrometry (ICP-MS) (Thermo Scientific, XSERIES 2). The X-ray diffraction (XRD) patterns of the CNT-Fe(0) and Sb(III)/Sb(V) loaded adsorbent were recorded using Hecus X-Ray Systems GmbH, Graz (Austria) Model: S3 MICRO. Nicolet 17DSX FT-IR Spectrometer in the attenuated total reflectance (ATR) mode using Ge crystal was used for FT-IR measurements. X-ray photoelectron spectroscopy (XPS) measurements were performed using PHI 5000 Versa Prob II, FEI Inc. spectrometer using non-monochromatic Al Kα radiation (1486.6 eV). Individual peaks were deconvoluted using XPSPEAK41 software with a Gaussian–Lorentzian line shape. Fitting of each spectral region was carried out by subtracting a nonlinear Shirley background.
2.4 Batch adsorption studies
Batch adsorption studies were carried out for CNT-plain, CNT-OX and CNT-Fe(0). For pH studies, experiments were conducted by varying the initial pH of the solution from 2 to 9 and equilibrating for 4 h using 50 ppm each of Sb(III)/Sb(V) at a dose rate 2.5 g l−1 in a total aqueous volume of 20 ml. After equilibration, the solutions were filtered and the concentration of antimony was analyzed using ICP MS instrument. All the measurements were done in triplicate and the average of these determinations were taken into account. Isotherm studies were carried out by maintaining the adsorbent dose at 2.5 g l−1 for CNT-plain, CNT-OX and Fe(0) and the concentration of Sb(III)/Sb(V) was varied from 10 to 150 ppm at pH 5 and equilibrations were carried out for 4 h at 110 RPM. In the case of CNT-Fe(0) alone, the adsorbent dose was maintained at 0.50 g l−1 and the concentration was varied from 10 to 300 ppm of Sb(III)/Sb(V) in a final aqueous volume of 20 ml. For the kinetic experiments, the solutions containing 50 ppm of Sb(III)/Sb(V) at pH 5 was equilibrated with the adsorbent dose of 2.5 g l−1. The samples were withdrawn at regular intervals of time and analyzed for antimony sorption. Recyclability of CNT-Fe(0) was evaluated for both Sb(III)/Sb(V) for 5 cycles using 0.05 M HCl as desorbent. Applicability of the prepared sorbent was evaluated for the spiked tap waters and spiked nuclear decontamination solutions which are detailed later.
3. Results and discussion
3.1 Structural characterization
3.1.1 Scanning electron microscopy. The scanning electron microscopic images of CNT-plain, CNT-OX, CNT-Fe(0) and CNT-Fe(0)–Sb(III) are shown in Fig. 1(a)–(d) respectively. It is evident from the image that upon oxidation with nitric acid there was no appreciable change in surface morphology. Doping of zerovalent iron on the surface of CNTs was evident from Fig. 1(c). Further EDAX plot of Sb(III) loaded CNT-Fe(0) revealed the presence of antimony and Fe in the range of 2.69 and 3.62% respectively.
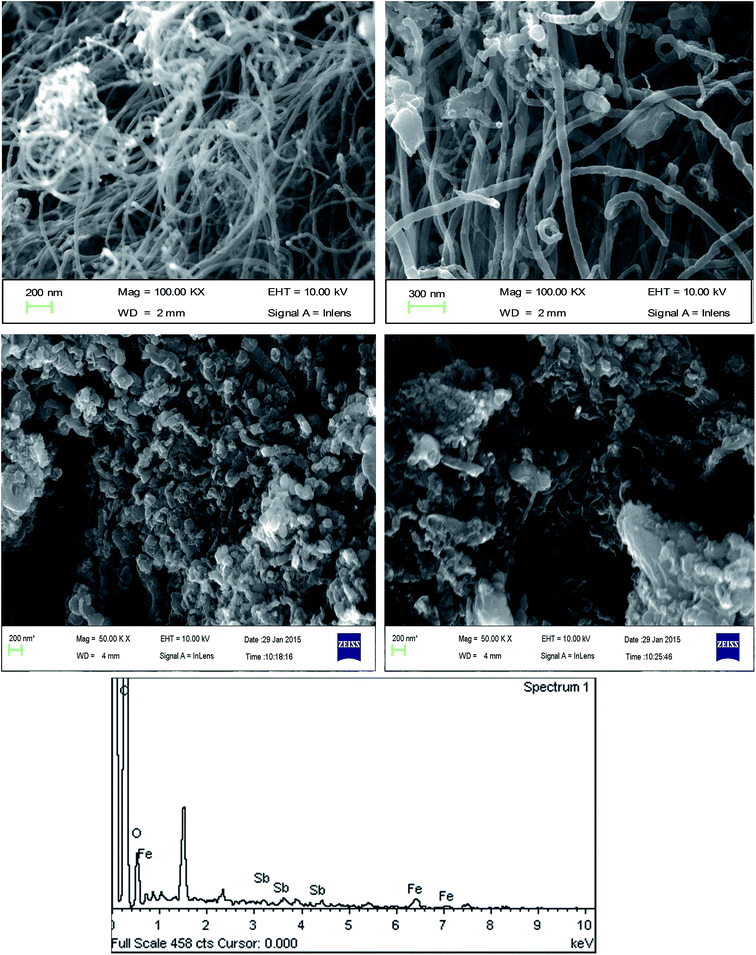 |
| Fig. 1 SEM images of (a) plain CNT (b) CNT-OX (c)CNT-ZVI (d) Sb(III) loaded CNT-ZVI (e) EDAX plot of Sb(III) loaded CNT-ZVI. | |
3.1.2 Surface area measurements. BET measurements revealed the surface area of plain CNTs prepared to be 76 m2 g−1. Upon oxidation with nitric acid removal of catalyst iron from the pores and opening of pores takes place and further unties the entwined CNTs29,30 which resulted in higher specific surface area (SSA). The SSA of CNT-OX was found to be 125 m2 g−1. A similar observation of increased surface area upon oxidation has been reported elsewhere.31,32 Further doping of CNT-OX with Fe(0) marginally increased the surface area to 132 m2 g−1. In our early studies on the applicability of Fe(0) coated MWCNTs towards arsenic removal the SSA increased from 70.2 to 78.78 m2 g−1 upon coating with Fe(0).27
3.1.3 XRD studies. The XRD spectra of CNT-Fe(0) and Sb(III) and Sb(V) doped CNT-Fe(0) are shown in Fig. 2. In all the three spectra a sharp peak around 26.4 and 44.3 could be attributed to 002 reflection plane of graphitic carbon of CNTs33 and αFe(110) phase of Fe(0)34 respectively. Further, in Sb(III) and Sb(V) loaded CNTs, diffraction peaks at 30.3(220), 35.6(311), 43.5(400) and 51.2(511) correspond to γFe2O3 or Fe3O4 phase (matched with JCPDS card no. (79-0417)). The result obtained is similar to the previous study reported by Zhao et al. (2014)26 where the authors reported the formation of magnetite after the reaction of PVA stabilized Fe(0) with Sb(III)/Sb(V). Thus the corroded Fe oxides aids the adsorption of both Sb(III) and Sb(V).
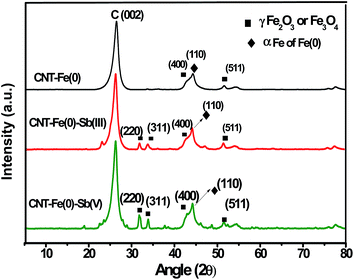 |
| Fig. 2 XRD Spectra of CNT-Fe(0) and Sb(III)/Sb(V) loaded CNT-Fe(0). | |
3.2 Effect of initial pH on adsorption
The effect of initial pH on the adsorption of Sb(III)/Sb(V) by CNT-Fe(0) is shown in Fig. 3(a). Efforts were not made to maintain the pH and a slight increase in pH was observed after equilibration with Sb(III)/Sb(V) (Fig. 3(b)). Fe(0) present in the surface of the adsorbent generates Fenton's reagent undergoing aerial oxidation and hydrated ferric oxides are formed on the surface25 as shown below eqn (1) |
Fe0 + O2 → H2O2/O2, H2O2, OH and Fe(II)
| (1) |
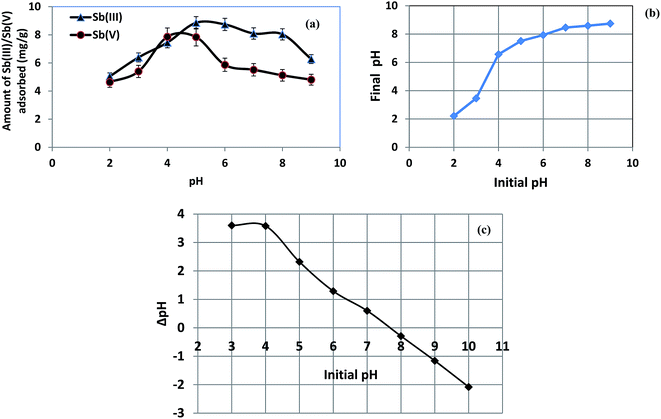 |
| Fig. 3 (a) Effect of initial pH on the adsorption behavior of Sb(III)/Sb(V) onto CNT-Fe(0); (b) changes of pH in the adsorption of Sb(III)/Sb(V) onto CNT-Fe(0) (c) zeropoint charge determination of CNT-Fe(0). | |
Thus the slight increase in pH could be attributed to the released hydroxide ions in the solution.
In the case of antimonite the sorption increases from pH 2 and remains maximum in the pH range 5 to 8 and at pH values greater than 8, decreased sorption was observed. For antimonate, it is evident that increased adsorption was observed from pH 2 and reaches a maximum in the range of pH 5 to 6 and subsequent increase in pH resulted in decreased adsorption. The result obtained in our study is similar to the one reported by Zhao et al.26 The ΔpH vs. initial pH plot revealed the zeropoint charge of CNT-Fe(0) to be 7.5 (Fig. 3(b)). Thus at pH values less than 7.5 the surface of the adsorbent is protonated. It is well known that zerovalent iron is very reactive and undergoes oxidation to form Fe2+/Fe3+ on the surface of Fe0. Further in the presence of water formed Fe3+ hydrolyses to form FeOOH+ under acidic conditions. Antimonate exists as Sb(OH)6− or SbO43− in the pH range of 4–10.1 It is evident from the graph that antimonate adsorption is maximum between pH 3 and 5 and subsequent increase in pH resulted in decreased adsorption. The electrostatic attraction between protonated ZVI surface which exists as FeOOH+ and antimonate anion results in the mass transfer of the antimonate anion from the solution to the surface then further reduction and complexation/precipitation occurs.26,35 Hence in the pH range above 5 due to the decreased amount of protonated surface charge decreased uptake of Sb(V) occurs compared to Sb(III). Thus there exists an electrostatic attraction between the adsorbent and adsorbate followed by the inner sphere complexation between Sb(V) and FeOOH similar to that of arsenic.36 Antimonite exists as neutral Sb(OH)3 in the pH range of 3–11.1 Thus similar to arsenite, there exists a complexation reaction between FeOOH and antimonite.31,36 Since surface of the adsorbent remains negative at high pH values decreased adsorption of both Sb(III) and Sb(V) were observed. Further, the inferior adsorption of both forms of antimony at higher pH could also be attributed to the passivation effect of Fe(0) loaded on CNT.
3.3 Adsorption isotherms
Antimonite and antimonate adsorption isotherms on CNT-plain, CNT-OX and CNT-Fe(0) at pH 4 are shown in Fig. 4. It is evident that CNT-Fe(0) was capable of removing both species of antimony and exhibited a very high adsorption capacity compared to plain and oxidized CNTs. Isotherm parameters were modeled by linearized Langmuir and Freundlich isotherm models and the data obtained are presented in Table 1. Correlation coefficients >0.96 were observed for Sb(III) and Sb(V) with CNT-Fe(0) systems. A comparison of adsorption capacities of other adsorbents for Sb(III) and Sb(V) are shown in Table 2. Due to the presence of Fe(0) on the surface of CNTs, CNT-Fe(0) exhibited higher capacity than plain and oxidized CNTs. Higher capacity of CNT-OX over plain CNTs could be attributed to the increased pores and surface area and the presence of hydroxyl and carbonyl groups which are introduced during oxidation procedure. These groups help in complexing the Sb(III)/Sb(V) species. It is evident from the table that CNT-Fe(0) exhibited very high sorption capacity compared to plain CNTs (0.360 mg g−1 for Sb(III)),16 PVA stabilized Fe0 (6.99 mg g−1 for Sb(III); 1.65 mg g−1 Sb(V)),26 ZrO2 loaded CNT (70.83 mg g−1 Sb(III) and 57.7 mg g−1 Sb(V)).17 Thus the prepared adsorbent is capable of removing both the species of antimony with high adsorption capacity compared to other adsorbents reported in the literature. Further equilibrium experiments were also performed with as prepared zerovalent iron and the capacity obtained was higher than plain CNT and CNT-OX and lesser than CNT-Fe(0). Nano zerovalent iron exhibits high van der Waal interaction, high Haymaker constant, high surface energy and high magnetic properties.37 Because of these reasons nano Fe(0) agglomerate in aqueous solutions. However, if it is anchored in CNTs the agglomeration is prevented and it is effectively dispersed on the surface of CNTs. Thus the adsorption capacity of CNT-Fe(0) towards Sb(III)/Sb(V) is comparatively high than plain Fe(0) owing to the surface electrical properties and functional groups. Thereby, Sb(III)/Sb(V) ions can be well enriched on the particle surfaces, and can more easily react with nano Fe(0). In other words, CNT facilitated the mass transfer of Sb(III)/Sb(V) from solution to iron surface, which contributes to the acceleration of surface reaction for Sb(III)/Sb(V) with iron.38 Further experiments were also carried out to study the amount of iron leached from the adsorbent after equilibration. After equilibration with the adsorbent for 4 h the amount of iron leached in the solution was found to be less than 25 ppm. Thus the adsorbent is suitable for recycling studies.
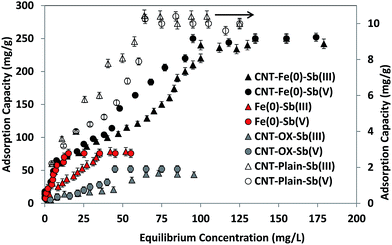 |
| Fig. 4 Equilibrium adsorption isotherms of Sb(III)/Sb(V) on CNT- Plain, CNT-OX and CNT-Fe(0). | |
Table 1 Langmuir and Freundlich model constants
Adsorbent |
Antimony species |
Langmuir model |
Freundlich model |
qmax (mg g−1) |
b (ml mg−1) |
R2 |
1/n |
Kf |
R2 |
CNT-plain |
Sb(III) |
10.86 |
0.0527 |
0.935 |
0.571 |
0.879 |
0.965 |
Sb(V) |
10.98 |
0.0309 |
0.842 |
0.503 |
0.926 |
0.894 |
CNT-OX |
Sb(III) |
47.61 |
0.0344 |
0.963 |
0.671 |
2.123 |
0.931 |
Sb(V) |
52.63 |
0.0300 |
0.930 |
0.867 |
1.435 |
0.903 |
Fe(0) |
Sb(III) |
83.33 |
6.8960 |
0.990 |
0.682 |
6.011 |
0.993 |
Sb(V) |
76.92 |
0.2766 |
0.981 |
0.615 |
14.32 |
0.975 |
CNT-Fe(0) |
Sb(III) |
250.0 |
0.0274 |
0.968 |
0.613 |
11.67 |
0.952 |
Sb(V) |
250.0 |
0.0400 |
0.981 |
0.576 |
15.03 |
0.967 |
Table 2 Comparison of maximum adsorption capacities (Langmuir isotherm) of various adsorbents for Sb(III)/Sb(V)
Adsorbent |
Maximum adsorption capacity (mg g−1) |
Concentration range, dose rate, equilibration time, operational pH |
Ref. |
Graphene |
8.056 – Sb(III) |
1–10 mg l−1, 0.4 g l−1, 4 h, 11 |
8 |
MWCNT |
0.360 – Sb(III) |
0–4 mg l−1, 0.2 g l−1, 0.5 h, 7 |
16 |
ZrO2-MWCNT |
70.83 – Sb(III), 57.17 – Sb(V) |
10–500 mg l−1, 1.0 g l−1, 12 h, 7 |
17 |
Graphene oxide–FeO |
158.6 – Sb(V) |
0–60 mg l−1, 0.3 g l−1, 24 h, 7 |
39 |
Fe(0)-PVA |
6.99 – Sb(III), 1.65 – Sb(V) |
0–20 mg l−1, 2 g l−1, 5 h, 7 |
26 |
Fe(0)-zeolite |
135.18 – Sb(III) |
20–220 mg l−1, 2 g l−1, 0.5 h, 10 |
35 |
Mesoporous activated alumina |
118.3 – Sb(V) |
2–37 mg l−1, 0.3 g l−1, 24 h, 5 |
53 |
CNT-plain |
10.31 – Sb(III), 10.91 – Sb(V) |
10–150 mg l−1, 2.5 g l−1, 4 h, 5 |
Present work |
CNT-OX |
45.5 – Sb(III), 50.0 – Sb(V) |
10–150 mg l−1, 2.5 g l−1, 4 h, 5 |
Present work |
Fe(0) |
83.33 – Sb(III), 76.92 – Sb(V) |
10–150 mg l−1,2.5 g l−1, 4 h, 5 |
Present work |
CNT-Fe(0) |
250.0 – Sb(III), 250.0 – Sb(V) |
10–300 mg l−1, 0.5 g l−1, 4 h, 5 |
Present work |
3.4 Adsorption kinetics
For the design of POU/POE units it is critical to evaluate the adsorbents for kinetic parameters. It is evident from preliminary investigations that sorption rate is rapid for both Sb(III) and Sb(V) with CNT-Fe(0) and around 50% of the adsorption was observed in the first 60 min and adsorption reached equilibrium after 4 h (Fig. 5(a)). The time required to attain equilibrium using CNT-Fe(0) is much more rapid compared to other recently reported nanocomposites such as Fe(0)-PVA (48 h)26 and graphene oxide/Schwertmannite nanocomposites (GOSCH) (24 h).39,40 The obtained kinetic data were modeled by pseudo first order model41 Ho's pseudo-second order model42 and Web Morris intraparticle diffusion model (Fig. 5(b))43 represented by eqn (2)–(4) respectively. |
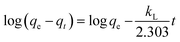 | (2) |
|
 | (3) |
|
 | (4) |
where kL is the Lagergren rate constant of adsorption (min−1);
the pseudo-second-order rate constant of adsorption (g mg−1 min−1); kint the intraparticle rate constant (g mg−1 min−0.5) qe and qt are the amounts of Sb(III)/Sb(V) adsorbed (mg g−1) at equilibrium and at time t, respectively. It is evident from Fig. 5(a), that rate of adsorption of both Sb(III) and Sb(V) followed pseudo second order model which implies that adsorption of both species of antimony is through chemisorptions involving sharing or exchange of electrons between the analyte and CNT-Fe(0). The model parameters are evaluated and detailed in Table 3. To further look into the diffusion process between sorbent and sorbate, the obtained kinetic data was modeled through Web–Morris intraparticle diffusion model given in eqn (4). Thus a plot of amount of Sb(III)/Sb(V) adsorbed over CNT-Fe(0) vs. √t (Fig. 5(b)) yielded a straight line with an intercept. This indicates that in addition to diffusion mechanism other boundary layer mechanisms could also be involved in the adsorption of Sb(III)/Sb(V) onto CNT-Fe(0).
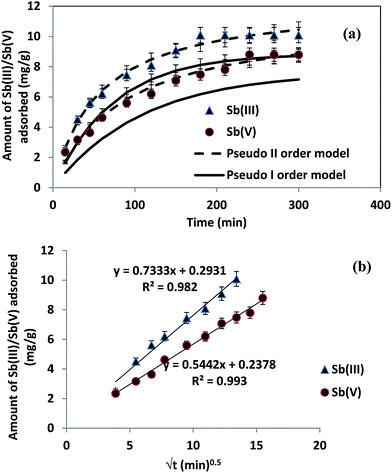 |
| Fig. 5 (a) Kinetics of sorption and (b) Web Morris model of CNT-Fe(0) and Sb(III)/Sb(V) systems. | |
Table 3 Kinetic parameters of CNT-Fe(0) with Sb(III) and Sb(V) systems
Arsenic species |
Pseudo first order |
Pseudo second order |
Web Morris model |
KL (min−1) |
R2 |
 (mg g−1 min−1) |
R2 |
kint |
R2 |
Sb(III) |
0.0138 |
0.982 |
0.0015 |
0.994 |
0.733 |
0.982 |
Sb(V) |
0.0192 |
0.996 |
0.0011 |
0.984 |
0.544 |
0.993 |
3.5 Effect of other ions on the adsorption behavior of Sb(III)/Sb(V) onto CNT-Fe(0)
Effect of commonly occurring ions like sulfate, nitrate, phosphate, chloride, silicate, arsenate and humic acid on the sorption behavior of Sb(III)/Sb(V) by CNT-Fe(0) was explored (Fig. 6). In both the species of Sb(III) and Sb(V) among all the ions tested, sulfate, arsenate, phosphate and silicate exhibited negative interference. The interference effect of these ions is as follows: PO43− > SiO32− > ASO43− > SO42−, NO3− > Cl−, humic acid. The effect was more pronounced in phosphate, silicate and arsenate which could be attributed to the chemical similarity with antimony oxyanions. However, the concentration levels of these ions tested in the present study is much higher than the actual concentration levels present the ground water. Thus, the prepared adsorbent could be used to remove Sb(III)/Sb(V) from ground water as well.
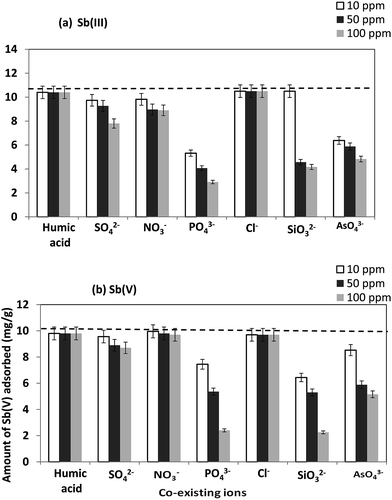 |
| Fig. 6 Effect of co-existing ions of the adsorption behavior of (a) Sb(III) and (b) Sb(V) on CNT-Fe(0); dotted line indicates the amount of Sb(III)/Sb(V) adsorbed without interfering ions. | |
3.6 Adsorption mechanism using XPS, FTIR and Raman studies
XPS studies were used as tools to study the interaction of antimony with the adsorbent at molecular levels. Fig. 7 depicts the survey scan spectrum of CNT-Fe(0), Sb(III) loaded CNT-Fe(0), Sb(V) loaded CNT-Fe(0). It is evident that O 1s, C 1s, Fe 2p3/2 and Sb 3d are identified. Thus the adsorption of both species of antimony onto CNT-Fe(0) is validated. The deconvoluted C 1s spectrum of CNT-ZVI (Fig. 8(a)) yielded three peaks at binding energies 282.9, 283.3 and 283.9 eV corresponding to C
C, C–O and C
O bonds respectively. It is evident from the figure (Fig. 8(b) and (c)) that after Sb(III) and Sb(V) adsorption the binding energies of the three peaks of carbon shifted to higher binding energies. This proves that after antimony adsorption the chemical environment around carbon is changed. This result is quite contrary to that obtained for Zr decorated CNTs used for the removal of Sb(III) and Sb(V), where there appeared to be no change in the carbon peak positions.17
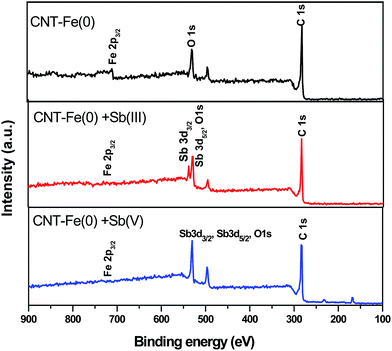 |
| Fig. 7 Survey spectra of CNT-Fe(0), Sb(III)/Sb(V) loaded CNT-Fe(0). | |
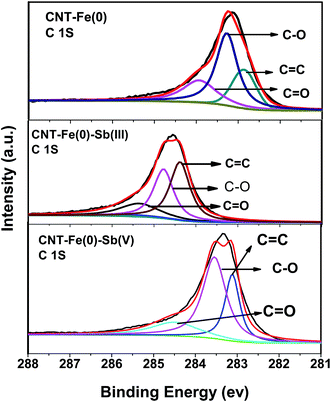 |
| Fig. 8 XPS spectra of C 1s of CNT-Fe(0) before and after Sb(III)/Sb(V) adsorption. | |
The Fe 2p spectra of CNT-Fe(0) (Fig. 9) revealed the photoelectron peaks at 723.6 and 710.1 eV corresponding to Fe 2p1/2 and Fe 2p3/2 respectively which confirms the presence of Fe-oxide particles29 on the surface. Additionally photoelectron peaks corresponding to Fe0 were observed at 705.9 and 717.69 eV.37 The Fe 2p binding energy showed that Fe is in the states of Fe(II) or Fe(III). It very difficult to resolve the oxidation states of Fe by XPS technique due to background and overlap of satellite peaks. After loading with Sb(III) and Sb(V) the changes observed in Fe 2p spectra is shown in Table 4. From the spectra the following points are evident
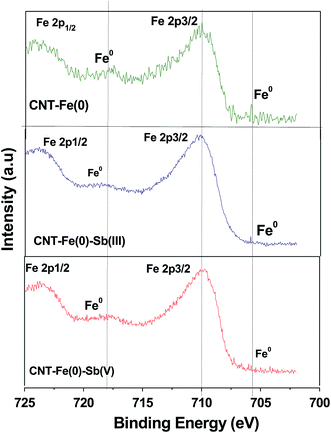 |
| Fig. 9 XPS spectra of Fe 2p of CNT-Fe(0) before and after Sb(III)/Sb(V) adsorption. | |
Table 4 Molecular level binding energies (eV) of Fe 2p in various systems
Adsorbent |
Fe–O |
Fe 2p3/2 |
Fe 2p1/2 |
CNT-Fe(0) |
710.10 |
723.60 |
CNT-Fe(0)–Sb(III) |
710.38 |
723.80 |
CNT-Fe(0)–Sb(V) |
709.42 |
723.50 |
(1) Fe0 is observed even after the reaction of Sb(III) and Sb(V) with the adsorbent.
(2) Marginal difference in the binding energies Fe 2p1/2 and Fe 2p3/2 of Fe–O bond after Sb(III) and Sb(V) adsorption was observed. The core level shifts in binding energies indicated an electron transfer in the valence band involving Fe–O and Sb. Thus it is evident that Fe–O plays an indispensable role in adsorption of both Sb species.
In a study involving polyvinyl alcohol stabilized zerovalent iron and Sb by Zhao et al.26 authors have reported the disappearance of Fe(0) peak after the reaction with Sb(III) and Sb(V). However, in the present study XPS spectra revealed the presence of Fe(0) even after the reaction with Sb(III)/Sb(V). The presence of Fe(0) in Sb(III)/Sb(V) loaded CNTs is further confirmed by XRD spectra as well (Fig. 2). Since the Fe(0) is coated over CNT bundles it is probable that a certain amount of Fe(0) is present in the tubular structure of CNTs which prevents it from oxidation with Sb(III)/Sb(V) species.
In Fig. 10 two photoelectron peaks at binding energy of 539.0 and 530.3 eV corresponding to Sb 3d3/2 and Sb 3d5/2 were observed.16,21,24 Analysis of Sb spectrum indicated the overlap of O 1s spectrum with the Sb 3d5/2 which is considerable intense peak for Sb. Further, the Sb binding energies of Sb 3d3/2 in Sb(III) oxide and Sb(V) oxide were reported to be 539.7 and 542.0 eV respectively.44 From the XPS spectra (Fig. 10), it was observed that Sb(III) and Sb(V) bound CNT-Fe(0), the Sb 3d3/2 spectra shifted to 539.0 and 536.0 eV respectively. This confirms that the adsorbed antimony species is chemically distinct from the respective oxides and precipitation of these oxides has not occurred. From the Sb spectrum it is not easy to decipher the oxidation state as Sb(III) or Sb(V) as both Sb(III) and Sb(V) oxides have similar binding energies.44 It is reported that pure Sb metal exhibits 3d3/2 and 3d5/2 at 527.8 and 536.9 eV respectively.45 Thus after adsorption of both Sb(III)/Sb(V) with CNT-Fe(0), absence of these peaks at these binding energy values indicates the absence of Sb(0). The proportion of Oads/Olatt increased from 31.0% to 36.9% and 48.6% after the adsorption of Sb(III) and Sb(V) respectively. The higher percentage in Sb(V) compared to Sb(III) after adsorption could be attributed to the fact that Sb(V) is bonded with five –OH groups, while Sb(III) is bonded with three –OH groups.16 The details of the XPS fitting results and the respective binding energies of various bonds are shown in Table 5.
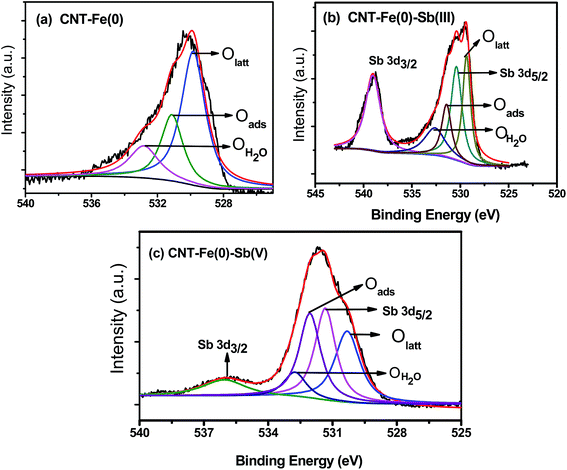 |
| Fig. 10 XPS Spectra of O 1s and Sb 3d of CNT-Fe(0) before and after Sb(III)/Sb(V) adsorption. | |
Table 5 XPS Fitting results of O 1s and Sb 3d core level spectra
Adsorbent |
Composition (%) |
Binding energies (eV) |
Oads/Olatt |
Olatt |
Oads |
OH2O |
Sb 3d5/2 |
Sb 3d3/2 |
CNT-Fe(0) |
31.0 |
529.8 |
531.1 |
532.8 |
— |
— |
CNT-Fe(0)–Sb(III) |
36.9 |
529.3 |
531.4 |
532.6 |
530.3 |
539.0 |
CNT-Fe(0)–Sb(V) |
48.6 |
530.3 |
532.1 |
532.7 |
531.3 |
536.0 |
The FTIR spectra of CNT-Fe(0), and CNT-Fe(0) after adsorption of antimonite and antimonate are shown in Fig. 11. The details of the FTIR bands are shown in Table 6.
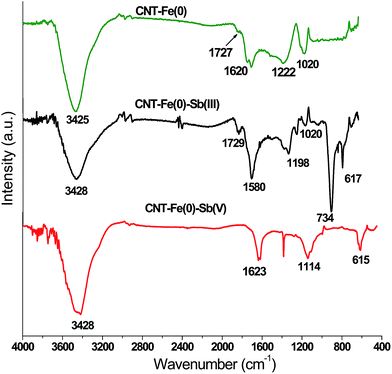 |
| Fig. 11 FTIR spectra of CNT-Fe(0) and Sb(III)/Sb(V) loaded CNT-Fe(0). | |
Table 6 FTIR spectral frequencies of CNT-Fe(0) and Sb(III)/Sb(V) loaded CNT-Fe(0)
Absorption bands |
Stretching frequencies (cm−1) |
CNT-Fe(0) |
CNT-Fe(0)–Sb(III) |
CNT-Fe(0)–Sb(V) |
–OH str |
3425 |
3428 |
3428 |
Surface adsorbed water/aromatic C–C str |
1620 |
1580 |
1623 |
Carbonyl group (C–O str) |
1727 |
1729 |
— |
C–O–H str |
1020 |
1020 |
— |
Aliphatic C–C str |
1222 |
1198 |
1114 |
Sb–O–Sb str of antimony trioxide |
— |
734 |
— |
Sb–O str |
— |
617 |
615 |
The broad FTIR peaks around 3425 cm−1 could be assigned to –OH stretching frequency. Further the –OH frequency of surface adsorbed water molecules along with C–C aromatic stretch is observed at 1620, 1580 and 1623 cm−1 for CNT-Fe(0), CNT-Fe(0)–Sb(III) and CNT-Fe(0)–Sb(V) respectively.39,46 In both CNT-Fe(0) and Sb(III) loaded CNT-Fe(0), the carbonyl stretching and C–O–H stretching were observed around 1725 and 1020 cm−1 respectively. The Fe–O vibrations were not observed in both plain and loaded CNT-Fe(0) owing to its low intensity in FTIR spectroscopy. A similar observation was reported by other researchers as well.46 In Sb(III) loaded adsorbent a sharp strong band at 734 cm−1 corresponding to the stretching vibration of Sb–O–Sb was observed. This band is similar to the Sb–O–Sb vibrations present in antimony trioxide (Sb2O3).47 Further in both Sb(III) and Sb(V) loaded FTIR spectra the vibration due to Sb–O was found at 617 and 615 cm−1 respectively.
The characteristic D and G band were observed in the Raman spectrum of CNT-Fe(0) and Sb(III)/Sb(V) loaded adsorbent at 1358 and 1572 cm−1 respectively (Fig. 12). The ratio of intensities of D and G band varied from 1.17 to 1.29 indicating the defects on the surface of the adsorbent.22 The weak bands observed around 400–600 cm−1 in CNT-Fe(0) could be assigned to oxidized iron particles on the surface of the sorbent. In antimonite adsorbed sorbent the Raman spectra exhibited a band around 623 cm−1, which could be attributed to the Sb–O stretch.39
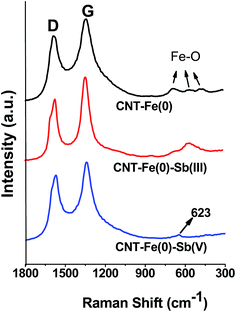 |
| Fig. 12 Raman Spectra of CNT-Fe(0) and Sb(III)/Sb(V) loaded CNT-Fe(0). | |
From the above discussions it is evident that Sb is chemically bonded with Fe in the adsorbent (XPS, FTIR and Raman). Though the presence of Fe(0) and absence of Sb(0) after Sb(III)/Sb(V) loading onto CNT-Fe(0) is evident, the changes in the oxidation states of both Sb and Fe after adsorption is not clear. From the spectral analysis and the reported literature, the following reaction mechanism is proposed.
It is well known that nano Fe(0) exhibits a core shell structure with Fe(0) as the core surrounded by oxidized iron oxide/hydroxide shell (Fig. 2 XRD spectra).48,49 The reactions of Fe(0) is represented as follows:
|
Fe0 + O2 + 2H+ → Fe2+ + H2O2
| (5) |
|
Fe0 + H2O2 + 2H+ → Fe2+ + 2H2O
| (6) |
|
Fe2+ + H2O2 → Fe3+ + OH− + OH
| (7) |
Thus nano Fe(0) exhibit ligand like properties in water. At pH around 4–5 Fe(0) is surrounded by positively charged iron hydroxide layer and in this pH Sb(V) exists as Sb(OH)6− or SbO43−. Thus there exists an electrostatic attraction and mass transport of Sb(V) species from the solution to the sorbent surface takes place. Further it is well known that nano Fe(0) can serve as an efficient electron donor (Fe0 → Fe2+ + 2e−, Eored = −0.44 V). Thus after mass transfer it is plausible that fraction of Sb(V) is reduced to Sb(III) and both Sb(III)/Sb(V) forms mono or bidentate complex with iron.36
|
Sb(OH)6− + 3H+ + 2e− → Sb(OH)3 + 3H2O, Ered = + 0.76 V
| (8) |
From XPS studies we did not observe the formation of Sb(0) in both Sb(III) and Sb(V). Thus it could be concluded that reduction of Sb(III) and Sb(V) to Sb(0) is not taking place in the present situation. The binding energies of Sb(III) or Sb(V) loaded 3d Sb spectrum was different from the pure Sb2O3 or Sb2O5 (Table 6) which further proves the complexation of Sb species with iron. In the case of Sb(III), initially surface adsorption of Sb(III) species takes place followed by complexation with Fe(II) or Fe(III) to form mixed hydroxides.
3.7 Recyclability studies
To evaluate the recyclability of the prepared CNT-Fe(0) adsorption desorption cycles were repeated for 5 cycles using 0.05 M HCl as desorbent. The results obtained for both Sb(III) and Sb(V) is shown in Table 7. It is evident from the table that after 5 cycles only 20% reduction in capacity was observed in both Sb(III) and Sb(V) which makes it an efficient material for treatment units. Amount of Sb(III)/Sb(V) desorbed in each cycle range from 65–75%. Thus decreased adsorption capacity in subsequent cycles could be attributed to the adsorbed Sb(III)/Sb(V) from previous cycles which were not desorbed. Further to study the change in the surface of CNT-Fe(0) during recycling studies, XPS and XRD studies were conducted on Sb(III)/Sb(V) loaded CNT-Fe(0) after 3rd and 5th cycles. From XPS studies (Fig. 13) it is evident that Fe(0) is observed even after 3rd and 5th cycle in both Sb(III) and Sb(V) loaded adsorbent. In X-ray diffraction studies (Fig. 14) apart from Fe(0) and iron oxides, formation of lepidochrocite (γFeO(OH)) was also evident. The peaks at 27.1(120), 38.1(111), 52.9(151) confirmed the formation of lepidochrocite (PDF #01-0136).50 The peaks arising due to the formation of lepidochrocite is stronger in 5th cycle compared to 3rd cycle as aging of the adsorbent causes crystallization of FeOOH. The formation of lepidochrocite could be attributed to the oxidation products of Fe(0) and iron oxides as detailed in equations below:33 |
4Fe0 + 3O2 + 2H2O → 4γFeOOH
| (9) |
|
4Fe3O4 + O2 + 6H2O → 2γFeOOH
| (10) |
|
γFe2O3 + H2O → 2γFeOOH
| (11) |
Table 7 Adsorption Desorption Cycles
Cycles |
Sb(III) |
Sb(V) |
Adsorbed (mg g−1) |
Desorbed (mg g−1) |
% reduction in adsorption |
Adsorbed (mg g−1) |
Desorbed (mg g−1) |
% reduction in adsorption |
1 |
22.36 |
16.92 |
NIL |
21.72 |
12.52 |
NIL |
2 |
20.84 |
15.28 |
6.8 |
20.2 |
11.52 |
7.00 |
3 |
20.16 |
12.96 |
9.84 |
19.32 |
10.48 |
11.05 |
4 |
19.04 |
12.20 |
14.8 |
18.56 |
9.72 |
14.55 |
5 |
18.32 |
11.98 |
18.06 |
17.68 |
8.68 |
18.60 |
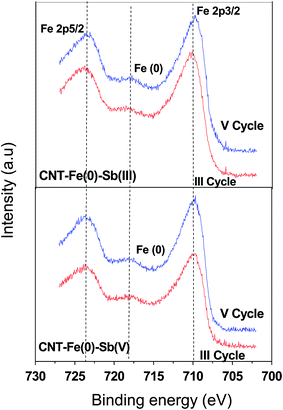 |
| Fig. 13 XPS spectra of Fe 2p in Sb(III) and Sb(V) loaded CNT-Fe(0) after III and V cycles. | |
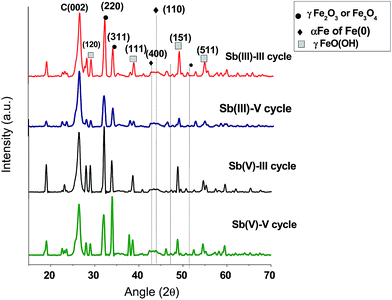 |
| Fig. 14 XRD spectra of Sb(III) and Sb(V) loaded CNT-Fe(0) after III and V cycles. | |
Thus it is evident even after 5 cycles Fe(0) is present on the CNTs and formation of lepidochrocite further helps in the adsorption of Sb(III)/Sb(V). Hence only a marginal decrease in the adsorption is observed after 5 cycles. Even in spite of extensive oxidation, a substantial portion (i.e. the core) of the iron nano particles remain as metallic (zerovalent) (XPS and XRD spectra). Li and Zhang51 conducted XANES studies on six weeks old Fe(0) nano particle and observed that 50 nm of Fe(0) particle had only 5 nm thickness of Fe2O3 thickness. Thus, they postulated that oxidation rate of iron decreases with growth of oxidized shell which protects or conserves the metallic core structure.
3.8 Application studies
3.8.1 Evaluation of the prepared adsorbents towards the removal of Sb(III)/Sb(V) from nuclear decontamination formulation. Adsorption of Sb(III)/Sb(V) from typical nuclear decontamination formulation (NAC)5,52 was evaluated in batch reactors for CNT-plain, CNT-OX and CNT-Fe(0). NAC solution consisted of nitriloacetic acid (1.37 mM or 262 ppm), ascorbic acid and citric acid (300 ppm). The results obtained are shown in Table 8. The results indicated that 97.6 and 92.7% removal of Sb(III) and Sb(V) were observed using 50 mg of CNT-Fe(0) reducing the concentration of Sb(III) and Sb(V) from 500 ppb down to 14 ppb and 36.47 ppb respectively. Using plain CNTs the removal percentage of Sb(III) and Sb(V) was found to be 25.6% and 28.2% respectively. Due to the oxidation with nitric acid and introduction of additional functional groups like carbonyl and hydroxyl groups on the surface of CNTs, CNT-OX exhibited higher capacity than plain CNTs. The removal rate was around 80% for both species of antimony. However, in an earlier study4 using nano TiO2–chitosan the removal capacities were in the order of 81.8 and 36.8% for Sb(III) and Sb(V) respectively for an initial concentration of 100 ppb of Sb(III)/Sb(V) in NAC medium. Hence, the material prepared in this study exhibited excellent removal potential of both species of antimony in NAC medium.
Table 8 Sorption of Sb(III)/Sb(V) from nuclear contamination formulation (NAC) medium
Metal ion solution |
Removal percentage (%) |
Plain CNTs |
CNT-OX |
CNT-Fe(0) |
500 ppb of Sb(III) in NAC medium |
25.6 ± 1.3 |
79.8 ± 3.9 |
97.6 ± 4.1 |
500 ppb of Sb(V) in NAC medium |
28.2 ± 1.6 |
80.4 ± 3.8 |
92.7 ± 3.6 |
3.8.2 Removal of Sb(III)/Sb(V) from tap water using the prepared adsorbents. Compared to deionized water tap water contains various anions, cations, organic matter including humic acid and microbes. Experiments were carried out to evaluate the performance of CNT-plain, CNT-OX and CNT-Fe(0) in the removal of Sb(III) and Sb(V) from IIT Kanpur tap water. The pH, TDS, hardness and TOC of the tap water used was found to be 7.8, 469 mg l−1, 140 mg l−1 and 10.1 mg l−1. To simulate antimony contaminated ground water, IIT Kanpur tap water was spiked with 500 μg l−1 of Sb(III)/Sb(V). Batch studies were carried out with various adsorbent doses of ranging from 0.1 to 0.5 g. From the results (Table 9) it is evident that CNT-Fe(0) is effective in bringing down the concentration of Sb(III)/Sb(V) from 500 μg l−1 to WHO limits of 6 μg l−1.
Table 9 Removal of Sb(III)/Sb(V) from spiked tap water
Metal ion solution |
Adsorbent dose (g) |
Residual concentration (μg l−1) |
Plain CNT |
CNT-OX |
CNT-Fe(0) |
500 ppb of Sb(III) in tap water |
0.1 |
73.00 ± 2.92 |
153.70 ± 4.61 |
94.90 ± 5.60 |
0.2 |
161.00 ± 6.11 |
120.30 ± 3.80 |
44.20 ± 2.21 |
0.3 |
175.00 ± 4.90 |
91.56 ± 2.29 |
18.50 ± 0.91 |
0.4 |
164.80 ± 5.40 |
76.91 ± 2.12 |
5.50 ± 0.25 |
0.5 |
164.00 ± 4.88 |
67.62 ± 1.89 |
4.30 ± 0.19 |
500 ppb of Sb(V) in tap water |
0.1 |
302.80 ± 8.11 |
151.50 ± 4.52 |
57.13 ± 2.85 |
0.2 |
282.70 ± 7.12 |
170.30 ± 4.85 |
40.08 ± 1.95 |
0.3 |
231.10 ± 6.02 |
102.70 ± 4.95 |
15.50 ± 0.81 |
0.4 |
215.50 ± 5.85 |
98.20 ± 3.89 |
6.98 ± 0.28 |
0.5 |
222.60 ± 6.10 |
68.85 ± 1.78 |
4.92 ± 0.22 |
4. Conclusions
Floating catalytic chemical vapor deposition method was used to prepare CNT (CNT-plain) and further functionalization was carried out by oxidation with nitric acid (CNT-OX) and coating with zerovalent iron (CNT-Fe(0)). Using various spectroscopic techniques both physical and chemical characterization was carried out. BET measurements revealed the surface area of plain CNTs, CNT-OX and CNT-Fe(0) to be 76, 125 and 132 m2 g−1 respectively. Bonding of Sb(III)/Sb(V) with surface oxidized iron was revealed by FTIR, XPS and Raman techniques. The adsorption capacities of the prepared adsorbents were in the order CNT-plain < CNT-OX < CNT-Fe(0). A very high adsorption capacity of CNT-Fe(0) (250.0 mg g−1) towards both Sb(III)and Sb(V) could be attributed to variety of factors including high surface area of CNTs, anchored functional groups like hydroxide and carbonyl, and highly reactive Fe(0). The adsorbent could be successfully recycled for 5 cycles without appreciable change in the adsorption behavior for both the species of antimony. The prepared adsorbents removed both species of antimony from tap water and nuclear decontamination formulations.
Acknowledgements
The funding received from Board of Research in Nuclear Sciences, Department of Atomic Energy, Mumbai, India (Ref. No. 2013/36/57-BRNS/2482) to carry out this work is gratefully acknowledged.
References
- M. Filella, N. Belzile and Y. W. Chen, Antimony in the environment: a review focused on natural waters: II. Relevant solution chemistry, Earth-Sci. Rev., 2002, 59, 265–285 CrossRef CAS.
- S. C. Wilson, P. V. Lockwood, P. M. Ashley and M. Tighe, The chemistry and behavior of antimony in the soil environment with comparisons to arsenic: a critical review, Environ. Pollut., 2010, 158, 1169–1181 CrossRef CAS PubMed.
- C. A. Johnson, H. Moench, P. Wersin, P. Kugler and C. Wenger, Solubility of antimony and other elements in samples taken from shooting ranges, J. Environ. Qual., 2005, 34, 248–254 CAS.
- P. A. Nishad, A. Bhaskarapillai and S. Velmurugan, Nano-titania-crosslinked chitosan composite as a superior sorbent for antimony(III) and (V), Carbohydr. Polym., 2014, 108, 169–175 CrossRef CAS PubMed.
- K. H. Neeb, The Radiochemistry of Nuclear Power Plants with Light Water Reactors, Walter de Gruyter & Co., Berlin, New York, 1997 Search PubMed.
- S. Velmurugan, A. L. Rufus, V. S. Sathyaseelan, V. Subramanian, V. K. Mittal and S. V. Narasimhan, Experience with dilute chemical decontamination in Indian pressurized heavy water reactors, Energy Procedia, 2011, 7, 645–649 CrossRef CAS.
- M. Filella, N. Belzile and Y.-W. Chen, Antimony in the environment: a review focused on natural waters: I. Occurrence, Earth-Sci. Rev., 2002, 57, 125–176 CrossRef CAS.
- Y. Leng, W. Guo, S. Su, C. Yi and L. Xing, Removal of antimony(III) from aqueous solution by graphene as an adsorbent, Chem. Eng. J., 2012, 211–212, 406–411 CrossRef CAS.
- J. Xi, M. He and C. Lin, Adsorption of antimony(III) and antimony(V) on bentonite: kinetics, thermodynamics and anion competition, Microchem. J., 2011, 97, 85–91 CrossRef CAS.
- Y. Miao, F. Han, B. Pan, Y. Niu, G. Nie and L. Lv, Antimony(V) removal from water by hydrated ferric oxides supported by calcite sand and polymeric anion exchanger, J. Environ. Sci., 2014, 26, 307–314 CrossRef CAS.
- J. Xi, M. He and C. Lin, Adsorption of antimony(V) on kaolinite as a function of pH, ionic strength and humic acid, Environ. Eng. Sci., 2010, 60, 715–722 CAS.
- V. K. Mittal, S. Bera, S. V. Narasimhan and S. Velmurugan, Adsorption behavior of antimony(III) oxyanions on magnetite surface in aqueous organic acid environment, Appl. Surf. Sci., 2013, 266, 272–279 CrossRef CAS.
- A. K. Leuz, H. Monch and C. A. Johnson, Sorption of Sb(III) and Sb(V) to goethite: influence on Sb(III) oxidation and mobilization, Environ. Sci. Technol., 2006, 40, 7277–7282 CrossRef CAS PubMed.
- X. Li, X. Dou and J. Li, Antimony(V) removal from water by iron–zirconium bimetal oxide: performance and mechanism, J. Environ. Sci., 2012, 24, 1197–1203 CrossRef CAS.
- W. Xu, H. Wang, R. Liu, X. Zhao and J. Qu, The mechanism of antimony(III) removal and its reactions on the surfaces of Fe–Mn binary oxide, J. Colloid Interface Sci., 2011, 363, 320–326 CrossRef CAS PubMed.
- M. A. Salam and R. M. Mohamed, Removal of antimony(III) by multi-walled carbon nanotubes from model solution and environmental samples, Chem. Eng. Res. Des., 2013, 91, 1352–1360 CrossRef CAS.
- J. Luo, X. Luo, J. Crittenden, J. Qu, Y. Bai, Y. Peng and J. Li, Removal of antimonite (Sb(III)) and antimonite (Sb(V)) from aqueous solution using carbon nanofibers that are decorated with zirconium oxide (ZrO2), Environ. Sci. Technol., 2015, 49, 11115–11124 CrossRef CAS PubMed.
- A. D. Henderson and A. H. Demond, Long-term performance of zero-valent iron permeable reactive barriers: a critical review, Environ. Eng. Sci., 2007, 24, 401–423 CrossRef CAS.
- A. B. Cundy, L. Hopkinson and R. L. Whitby, Use of iron-based technologies in contaminated land
and groundwater remediation: a review, Sci. Total Environ., 2008, 400, 42–51 CrossRef CAS PubMed.
- R. A. Crane and T. B. Scott, Nanoscale zero-valent iron: future prospects for an emerging water treatment technology, J. Hazard. Mater., 2012, 211–212, 112–125 CrossRef CAS PubMed.
- X. Lv, J. Xu, G. Jiang and X. Xu, Removal of chromium(VI) from wastewater by nanoscale zero-valent iron particles supported on multiwalled carbon nanotubes, Chemosphere, 2011, 85, 1204–1209 CrossRef CAS PubMed.
- G. Sheng, J. Hu, H. Li, J. Li and Y. Huang, Enhanced sequestration of Cr(VI) by nanoscale zero-valent iron supported on layered double hydroxide by batch and XAFS study, Chemosphere, 2016, 148, 227–232 CrossRef CAS PubMed.
- G. Sheng, P. Yang, Y. Tang, Q. Hu, H. Li, X. Ren, B. Hu, X. Wang and Y. Huang, New insights into the primary roles of diatomite in the enhanced sequestration of UO22+ by zerovalent iron nanoparticles: an advanced approach utilizing XPS and EXAFS, Appl. Catal., B, 2016, 193, 189–197 CrossRef CAS.
- G. Sheng, A. Alsaedi, W. Shammakh, S. Monaquel, J. Sheng, X. Wang, H. Li and Y. Huang, Enhanced sequestration of selenite in water by nanoscale zero valent iron immobilization on carbon nanotubes by a combined batch, XPS and XAFS investigation, Carbon, 2016, 99, 123–130 CrossRef CAS.
- D. Chauhan, J. Dwivedi and N. Sankararamakrishnan, Novel chitosan/PVA/zerovalent iron biopolymeric nanofibers with enhanced arsenic removal applications, Environ. Sci. Pollut. Res., 2014, 21, 9430–9442 CrossRef CAS PubMed.
- X. Zhao, X. Dou, D. Mohan, C. U. Pittman, Y. S. Ok and X. Jin, Antimonate and antimonite adsorption by a polyvinyl alcohol-stabilized granular adsorbent containing nanoscale zero-valent iron, Chem. Eng. J., 2014, 247, 250–257 CrossRef CAS.
- N. Sankararamakrishnan, A. Gupta and S. R. Vidyarthi, Enhanced arsenic removal at neutral pH using functionalized multiwalled carbon nanotubes, J. Environ. Chem. Eng., 2014, 2, 802–810 CrossRef CAS.
- N. Sankararamakrishnan, D. Chauhan and J. Dwivedi, Synthesis of functionalized carbon nanotubes by floating catalytic chemical vapor deposition method and their sorption behavior toward arsenic, Chem. Eng. J., 2016, 284, 599–608 CrossRef CAS.
- I. D. Rosca, F. Watari, M. Uo and T. Akasaka, Oxidation of multiwalled carbon nanotubes by nitric acid, Carbon, 2005, 43, 3124–3131 CrossRef CAS.
- X.-Q. Li, D. WElliott and W.-X. Zhang, Zero-Valent Iron Nanoparticles for Abatement of Environmental Pollutants: Materials and Engineering Aspects, Crit. Rev. Solid State Mater. Sci., 2006, 31, 111–122 CrossRef CAS.
- S. Mishra, J. Dwivedi, A. Kumar and N. Sankararamakrishnan, The synthesis and characterization of tributyl phosphate grafted carbon nanotubes by the floating catalytic chemical vapor deposition method and their sorption behavior towards uranium, New J. Chem., 2016, 40, 1213–1221 RSC.
- A. Gupta, S. R. Vidyarthi and N. Sankararamakrishnan, Enhanced sorption of mercury from compact fluorescent bulbs and contaminated water streams using functionalized multiwalled carbon nanotubes, J. Hazard. Mater., 2014, 274, 132–144 CrossRef CAS PubMed.
- A. Liu, J. Liu, B. Panac and W.-X. Zhang, Formation of lepidocrocite (g-FeOOH) from oxidation of nanoscale zero-valent iron (nZVI) in oxygenated water, RSC Adv., 2014, 4, 57377–57382 RSC.
- J. Gurgul, M. T. Rinke, I. Schellenberg, H. Eckert and R. Pottgen, The antimonide oxides REZnSbO and REMnSbO (RE = Ce, Pr)-An XPS Study, Solid State Sci., 2013, 17, 122–127 CrossRef CAS.
- Z. Zhou, C. Dai, X. Zhou, J. Zhao and Y. Zhang, The Removal of Antimony by Novel NZVI-Zeolite: The Role of Iron Transformation, Water, Air, Soil Pollut., 2015, 226, 76 CrossRef.
- P. Dorjee, D. Amarasiriwardena and B. Xing, Antimony adsorption by zero-valent iron nanoparticles (nZVI): ion chromatography-inductively coupled plasma mass
spectrometry (IC-ICP-MS) study, Microchem. J., 2014, 116, 15–23 CrossRef CAS.
- L. Li, M. Fan, R. C. Brown, J. V. Leeuwen, J. W. Leeuwen, J. Wang, W. Wang, Y. Song and P. Zhang, Synthesis, properties, and environmental applications of nanoscale iron-based materials: a review, Environ. Sci. Technol., 2010, 36, 405–431 CrossRef.
- G. Sheng, A. Alsaedi, W. Shammakh, S. Monaquel, J. Sheng, X. Wang, H. Li and Y. Huang, Enhanced sequestration of selenite in water by nanoscale zero valent iron immobilization on carbon nanotubes by a combined batch, XPSand XAFS investigation, Carbon, 2016, 99, 123–130 CrossRef CAS.
- S. Dong, X. Dou, D. Mohan, C. U. Pittman Jr and J. Luo, Synthesis of graphene oxide/Schwertmannite nanocomposites and their application in Sb(V) adsorption from water, Chem. Eng. J., 2015, 270, 205–214 CrossRef CAS.
- X. Guo, Z. Wu, M. He, X. Meng, X. Jin, N. Qiu and J. Zhang, Adsorption of antimony onto iron oxyhydroxides: adsorption behavior and surface structure, J. Hazard. Mater., 2014, 276, 339–345 CrossRef CAS PubMed.
- S. Lagergren, Zur theorie der sogenannten adsorption gelster stoffe, K. Sven. Vetenskapsakad. Handl., 1898, 24, 1–39 Search PubMed.
- Y.-S. Ho and G. McKay, Pseudo-second order model for sorption processes, Process Biochem., 1999, 34, 451–465 CrossRef CAS.
- W. J. Weber and J. C. Morris, Kinetics of adsorption on carbon from solution, J. Sanit. Eng. Div., Am. Soc. Civ. Eng., 1963, 89, 31–60 Search PubMed.
- R. Izquierdo, E. Sacher and A. Yelon, X-ray photoelectron spectra of antimony oxides, Appl. Surf. Sci., 1989, 40, 175–177 CrossRef CAS.
- C. D. Wagner, J. F. Moulder, L. E. Davis and W. M. Riggs, Handbook of XPS, Perkin Elmer corporation, Eden Prairie, Missesota (USA), 1979 Search PubMed.
- X. Luo, C. Wang, L. Wang, F. Deng, S. Luo, X. Tu and C. Au, Nanocomposites of graphene oxide-hydrated zirconium oxide for simultaneous removal of As(III) and As(V) from water, Chem. Eng. J., 2013, 220, 98–106 CrossRef CAS.
- F. A. Miller and C. H. Wilkins, Infrared spectra and characterization of inorganic ions, Anal. Chem., 1952, 24, 1253–1294 CrossRef CAS.
- X.-Q. Li, D. W. Elliott and W.-X. Zhang, Zero-valent iron nanoparticles for abatement of environmental pollutants: materials and engineering aspects, Crit. Rev. Solid State Mater. Sci., 2006, 31, 111–122 CrossRef CAS.
- W.-X. Zhang, Nanoscale iron particles for environmental remediation: an overview, J. Nanopart. Res., 2003, 5, 232–332 CrossRef.
- G. B. McGarvey, K. B. Burnett and D. G. Owen, Preparation and reactivity of lepidocrocite under simulated feedwater conditions, Ind. Eng. Chem. Res., 1998, 37, 412–419 CrossRef CAS.
- X.-G. Li and W.-X. Zhang, Sequestration of metal cations with zerovalent iron nanoparticles– a study with high resolution X-ray photoelectron spectroscopy (HR-XPS), J. Phys. Chem. C, 2007, 111, 6939–6946 CAS.
- A. L. Rufus, S. Velmurugan, V. S. Sathyaseelan and S. V. Narasimhan, Comparative studies of nitriloacetic acid (NTA) and EDTA as formulation constituents for chemical decontamination of primary coolant systems of nuclear power plants, Prog. Nucl. Energy, 2004, 44, 13–31 CrossRef CAS.
- X. Dou, D. Mohan, X. Zhao and C. U. Pittman Jr, Antimonate removal from water using hierarchical macro-/mesoporous amorphous alumina, Chem. Eng. J., 2015, 264, 617–624 CrossRef CAS.
|
This journal is © The Royal Society of Chemistry 2016 |
Click here to see how this site uses Cookies. View our privacy policy here.