DOI:
10.1039/C6RA18715C
(Paper)
RSC Adv., 2016,
6, 81838-81846
Design and synthesis of sugar-benzohydrazides: low molecular weight organogelators†
Received
23rd July 2016
, Accepted 22nd August 2016
First published on 23rd August 2016
Abstract
A novel class of methyltriglycol benzohydrazide based N-glycosylamines containing long alkyl chain derivatives were synthesized in good yield and characterized using NMR (1H and 13C) spectral analysis. The gelation test shows that these derivatives form gels with a wide range of polar (1,2-dichlorobenzene, chloroform) and non-polar (1,2-dichloroethane, toluene, benzene) solvents even with lower CGC (1.0%). Morphological studies of these amphiphilic glycosidic gels were studied using FE-SEM, TEM and powder XRD analysis. Studies further show the formation of 3D self-assembled spherical and rod structures which is due to the influence of organic solvents. It is found that hydrogen bonding and van der Waals interactions play an important role in the self-assembled structure.
1. Introduction
Several inorganic and organic molecules and polymers spontaneously self-assemble into various nanostructures, such as, toroids, tubes, helices, disks, rod and fibers.1–4 Smart gels, also known as stimuli-responsive gels, respond to external stimuli by changing their physico-chemical properties. Smart materials based on stimuli-responsive Low-Molecular Weight Organo Gelators (LMWOGs) have potential applications in drug delivery,5,6 photonics,7 sensors and self-assembly.8,9 A wide variety of organogels that can respond to external stimuli, such as light, temperature, sound, mechanical stimuli (sonication-aided gelation), anions, metal ions, redox, proton/pH, and small molecules have been investigated.10 The basic principles, the structural features of gelators, influence of solvents and role of non-covalent interactions, properties of gels, and their characterization have been discussed in the literature.11 Recently low molecular weight organogels have attracted much interest because of their unique features and potential applications for novel organic soft materials.12,13 It is known that most organogelators can self-assemble into nanoscale superstructures, such as fibers, rods, and ribbons through hydrogen bonding, π–π stacking, van der Waals, coordination, and charge-transfer interactions to create three-dimensional networks which lead to the gelation of organic solvents.14 Gels are materials formed mostly by liquid, yet they rheologically behave like solids because of a three-dimensional network that entraps and immobilizes the fluid. In comparison to traditional polymeric gels, viscoelastic materials made of LMOGs show the ultimate level of innovation in this area, by means of a better-defined mode of self-assembly than the former and the reversibility of their Self-Assembled Fibrillar Network (SAFIN).15 LMOG compounds self-organize through supramolecular, noncovalent interactions, to form gels in water (hydrogel), organic solvents (organogel) or ionic liquids that can be sensitive to a variety of physical (temperature, light, etc.) and/or chemical (pH, ions, etc.) property.16 The design and modification of LMOGs are easier than those for polymers. In addition, direct drug loading during the organogel preparation makes it possible for a great quantity of drug loading and precise control of drug concentration.17
Based on the available reports it is clear that benzohydrazides show various biological activities like antimicrobial, antidepressant, analgesic, anti-inflammatory, anti-tumour, anti-HIV, anticancer, anticonvulsant, anti-tubercular, anticancer, antimalarial activities etc.18 Since the carbohydrate molecule are biocompatible, the gel derived from those molecule have wide application in biology and also as functional materials.19,20 Moreover, the abundant availability of saccharides enhance research into the design of novel sugar based gelator molecules. To the best of our knowledge, there is no report on sugar-benzohydrazide based organogelators. Here, we designed several new sugar benzohydrazide derivatives, which form organogels with different morphologies in various organic solvents.21,22 In this work, twelve different novel class of long alkyl chain based hydrazide N-glycosylamines derivatives and its organogels are reported. The change in morphology of gels and the self-assembly pathways were very well studied using SEM, TEM, powder XRD and rheological study.
2. Materials and methods
4-Hydroxy benzoic acid and hydrazine hydrate, were purchased from Merck, India. Four different long alkyl bromides and triethylene glycol monomethyl ether, deuterated chloroform (CDCl3), deuterated DMSO-d6 and palladium-charcoal activated (10%) were purchased from Sigma Aldrich, India. D-Glucose, hexane, methanol, ethanol, silica gel (100–200 mesh), Et2O, petroleum ether, ethyl acetate, and dimethyl formamide were purchased from SRL, India. The water used in all experiments was of Millipore Milli-Q grade. NMR spectra were recorded on a Bruker DRX 300 MHz instrument in CDCl3 (with a few drops of DMSO-d6). SEM and FE-SEM images were recorded on a Hitachi SU-3500 instrument. Transmission electron microscopic studies were performed by using Hitachi Transmission electron microscope H-9500. PXRD patterns are recorded by X-ray diffractometer with Cu Kα radiation source. Differential Scanning Calorimetry (DSC) experiment was performed using TA waters Q20 instrument. Fourier transform-infrared spectroscopy studies were performed by using Agilent Technology Carry 630 FT-IR instrument. UV-vis spectrophotometer and fluorescence spectrophotometer studies were performed by Agilent Technology UV-Vis spectrophotometer and Agilent Technology Carry Eclipse Fluorescence spectrophotometers respectively. Rheological measurements were carried out with Anton Paar-Rheoplus instrument. Oscillatory experiments were performed in a 0.001–100 Hz frequency range with 0.1% constant strain on 0.5% gel of 8 in 1,2-dichloroethane at 25 °C. FT-IR spectroscopy photophysical studies were obtained from Central University of Tamil Nadu, Thiruvarur, India. Rheological study were obtained from IIT Madras, Chennai, India. Details of the synthetic procedures of gelator derivatives and instrumentation are given in the ESI.†
3. Result and discussion
3.1. Design and synthesis of benzohydrazide based N-glycosylamines
The gelators have been designed in such a way that they can form gels with different organic solvents. So the structure of the gelators has three different parts to enhance the gelating ability. The three parts are long alkyl chain, benzohydrazide and protected D-glucose moiety. The nature of long alkyl chain is hydrophobic so it has been introduced to increase solubility in organic solvents. Benzohydrazine has an aromatic ring as well as an amide group. We have seen in literature that π–π stacking of aromatic ring and amide group possess the ability to gelate organic solvents. 4,6-O-Protected D-glucopyranoside has two hydroxyl groups, so it is partially polar as well as nonpolar. This property of sugar increases the gelating ability of the designed gelators. Long alkyl chain substituted benzohydrazides (1–4) were synthesized in three steps from 4-hydroxybenzoic acid. The first step is being the esterification of 4-hydroxybenzoic acid with an alcohol in the presence of an acid catalyst. O-Alkylation of ester derivatives with long chain bromides and methyltriglycol was carried out in the next step. The 4-O-alkylated-esters were treated with hydroxylamine to the long alkyl chain substituted benzohydrazide.23 N-Glycosylamine based long alkyl chain substituted benzohydrazide derivatives (8–19) were synthesized from benzohydrazine (1–4) and 4,6-O-ethylidene-D-glucopyranose (EGP)24 4,6-O-butylidene-D-glucopyranose (BGP)25 and 4,6-O-benzylidene-D-glucopyranose (BzGP)26 (Scheme 1). The reactants were chosen to have desired functional group such as the primary amine in the aromatic moiety and active hydroxyl group in the 4,6-O-producted-D-glucose moieties. All the N-glycosylamines [8–19] were characterized using 1H and 13C NMR and subjected to gelation with a wide range of solvents. Analytical techniques viz., SEM, TEM, powder XRD and DSC were used to study the self-assembly of gel.
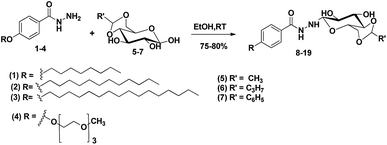 |
| Scheme 1 Synthesis of long alkyl chain substituted benzohydrazide-N-glycosylamines (8–19). | |
In the 1H NMR of the long alkyl chain substituted benzohydrazide-N-glycosylamines (8–19) the signal of amide–NH proton appears at around 10.0–9.0 ppm, the signals of aromatic protons appear in the range of 7.9–6.7 ppm and the protons present in the sugar unit appear in between 5.6–3.2 ppm. D-Glucose unit present in all the synthesized N-glycosylamines was found to exist as the β-anomer, which was identified by using coupling constant in 1H NMR. The chemical shift of the anomeric protons was found to be in the range of 5.46–3.49 ppm with corresponding coupling constant values (J = 7.0–12.0 Hz) (Table 1). However, 13C NMR studies show peaks around 41–18 ppm, 106–72 ppm and 162–110 ppm corresponding to the alkyl chain, saccharide carbon, including acetal and aromatic carbons respectively.
Table 1 Synthesis and spectral characterization of long alkyl chain substituted benzohydrazide-N-glycosylamines (8–19) in 1,2-dichloroethane
Compound no. |
R |
R′ |
t (H) |
Ano-H |
Yield (%) |
CGC (W/V%) |
8 |
OC8H17 |
CH3 |
12 |
3.49, 9.0 |
87 |
0.5 |
9 |
OC8H17 |
C3H7 |
8 |
3.45, 10.2 |
83 |
1.5 |
10 |
OC8H17 |
C6H5 |
11 |
3.72, 7.2 |
73 |
1 |
11 |
OC12H25 |
CH3 |
12 |
3.70, 8.7 |
83 |
0.5 |
12 |
OC12H25 |
C3H7 |
8 |
3.80, 8.0 |
75 |
1.5 |
13 |
OC12H25 |
C6H5 |
11 |
3.77, 8.4 |
70 |
1 |
14 |
OC16H33 |
CH3 |
12 |
3.50, 9.0 |
73 |
1.5 |
15 |
OC16H33 |
C3H7 |
8 |
3.50, 12.0 |
68 |
0.5 |
16 |
OC16H33 |
C6H5 |
14 |
3.57, 9.0 |
70 |
1 |
17 |
C7H15O4 |
CH3 |
12 |
5.48, 8.6 |
82 |
1.5 |
18 |
C7H15O4 |
C3H7 |
8 |
5.45, 9.0 |
80 |
0.5 |
19 |
C7H15O4 |
C6H5 |
11 |
5.46, 9.8 |
75 |
1.0 |
3.2. Gelation studies
Gelating abilities of different classes of sugar derivatives are available in the literature.27 The known amount of (1 mg)/N-glycosylamines (8–19) was added to 1 mL of solvent in a glass vial and heated in order to get a homogeneous solution. After cooling to room temperature, the vial was turned upside down to verify the gel formation. The reversibility of the gelation was confirmed by repeated heating and cooling.28 The critical gelation concentration (CGC: <1%) of benzohydrazide-N-glycosylamines (8–19) was determined from the minimum amount of gelator required for the formation of gel at room temperature and the gel study was carried out using twelve different solvents. The gelation results are summarized in (Table 2). Polar (ethanol, tetrahydrofuran, ethyl acetate) and non-polar (hexane, chloroform, benzene, toluene, 1,2-dichloroethane, cyclohexane, 1,2-dichlorobenzene, p-xylene) solvents were used for gelation. Among the aliphatic solvent viz., chloroform and 1,2-dichloroethane, aromatic solvents viz., toluene, p-xylene, 1,2-dichlorobenzene and benzene were found to be the best for gelation, which is due to the presence of long alkyl chains. A schematic representatives of the sol to gel upon cooling and heating due to self-assembly and dis-assembly of N-glycosylamines is shown in Fig. 1.
Table 2 Gelation of long alkyl chain substituted benzohydrazide based N-glycosylamines (8–19)
Solvent |
8 |
9 |
10 |
11 |
12 |
13 |
14 |
15 |
16 |
17 |
18 |
19 |
Hexane |
I |
I |
I |
I |
I |
I |
I |
I |
I |
I |
I |
I |
Cyclohexane |
P.G. |
P.G. |
P.G. |
P.G. |
P.G. |
P.G. |
P.G. |
P.G. |
P.G. |
I |
I |
I |
Ethyl acetate |
I |
I |
I |
I |
I |
I |
I |
I |
I |
I |
I |
I |
Chloroform |
G |
G |
G |
G |
G |
G |
G |
G |
G |
S |
S |
S |
1,2-Dichlorethane |
G |
G |
G |
G |
G |
G |
G |
G |
G |
S |
S |
S |
Tetrahydrofuran |
S |
S |
S |
S |
S |
S |
S |
S |
S |
S |
S |
S |
Ethanol |
S |
S |
S |
S |
S |
S |
S |
S |
S |
S |
S |
S |
Methanol |
S |
S |
S |
S |
S |
S |
S |
S |
S |
S |
S |
S |
Toluene |
G |
G |
G |
G |
G |
G |
G |
G |
G |
P.G. |
P.G. |
G |
p-Xylene |
G |
G |
G |
G |
G |
G |
G |
G |
G |
G |
P.G. |
G |
1,2-o-Dichlorobenzene |
G |
G |
G |
G |
G |
G |
G |
G |
G |
G |
G |
G |
Benzene |
G |
G |
G |
G |
G |
G |
G |
G |
G |
P.G. |
P.G. |
P.G. |
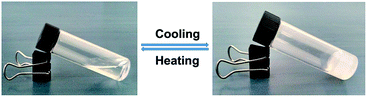 |
| Fig. 1 Photograph of solution to gel transformation of long alkyl chain substituted benzohydrazide based-glycosylamine 8. | |
3.2.1. Influence of solvent on gelation. The gelation of low molecular weight molecules in organic solvents is a result of the gelator–gelator and solvent–gelator interactions, both involve specific and nonspecific intermolecular forces. The former refers to H-bonding, which can be further distinguished as H-bond donor (solvent acidity) or H-bond acceptor (solvent basicity) interactions. The latter include dipole–dipole, dipole-induced, and instantaneous dipole-induced forces (also called dispersion forces). The influence of these forces on gelation is critical for understanding the process of gel formation. In particularly, correlations of gelation number to solvent parameters can provide useful information on the specific interactions that dominate gel formation using the sugar amide gelators.29
3.3. Synthesis of gels
The gelation ability of long alkyl chain substituted N-glycosylamines was determined by the “stable to inversion of the container” method. The gelation properties have been tested with twelve different solvents as follows: gelator (1 mg) was mixed in a close capped test tube with 1 mL of solvent to result in a concentration of 1% (g mL−1) and the mixture was heated until a clear solution is obtained. By this procedure, the solvent boiling point becomes higher than that under standard atmospheric pressure. The sample vial was cooled in air to 25 °C, left for an hour at this temperature and then turned upside down. When the gelator formed a clear or slightly opaque gel by immobilizing the solvent at this stage, it was denoted by “G”. Partial gel is denoted as P.G., insoluble is as I. Some of them remains as solution is donated S and precipitation is donated by P, respectively.30
3.4. Morphological analysis of long alkyl chain substituted benzohydrazide based N-glycosylamines
The morphology of long alkyl chain substituted benzohydrazide-N-glycosylamines in the gel state were studied with SEM and HR-TEM analysis using 0.5% w/v of N-glycosylamines 8 and 17 in 1,2-dichloroethane. The gel of N-glycosylamines 8 and 17 in 1,2-dichloroethane was prepared with CGC 0.5% and allowed to dry at ambient temperature to obtain xerogel and subjected to SEM analysis. SEM images shows that N-glycosylamines 8 and 17 self-assemble as a nanorod31 and textured spheroids network. Compound 8, long chain substituted benzohydrazide-N-glycosylamine derivatives bearing partially protected sugar, exhibits rod aggregated morphology with nanorods (Fig. 2), and methyltriglycol benzohydrazide introduced 4,6-O-protected sugar exhibits textured spheroids networks (Fig. 3).32 The formation of elongated fiber-like aggregates indicates that the self-assembly of long chain substituted benzohydrazide is driven by strong directional intermolecular interaction. To ascertain whether hydrogen bonding plays a role in the gelation process.33
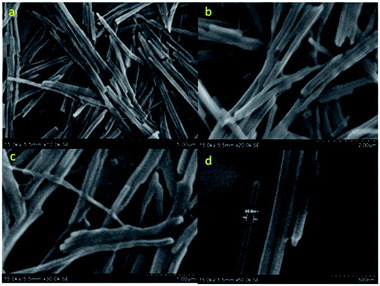 |
| Fig. 2 SEM images of organogel formed from dichloroethane under different magnification: (8a) 5 μm, (8b) 2 μm, (8c) 1 μm and (8d) 500 nm. | |
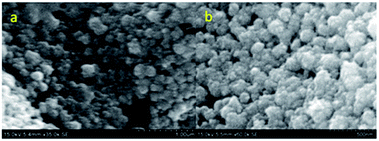 |
| Fig. 3 SEM images of compound 17 (dissolved in 1,2-dichloroethane) under different magnification: (a) 1 μm, (b) 500 nm. | |
The presence of rods and fibrous morphology within the organogels of 8 and 17 was further confirmed by HR-TEM images of their respective xerogels. The HR-TEM images at more than five-times lower concentration than the minimum gelator concentration showed similar rod and intertwined fibers of approximately 1 μm and 0.5 nm thickness for 8 and 17 (Fig. 4 and 5), which supports the corresponding FESEM images (Fig. 2 and 3).34 HR-TEM analysis of gel was carried out at lower concentration of the respective materials i.e., gels of the long chain based glycosylamines (8) were prepared in 1,2-dichloroethane at the concentration of 0.5% (w/v) and then dispersed into dichloroethane to obtain the concentration of 1 × 10−5 M.35 The interior morphology of compound 17 was determined using HR-TEM and the representative HR-TEM images is shown in Fig. 5a and b. The aggregation mode was identified as fibers due to the influence methyltriglycol. TEM analysis of the gel shows thin and lengthy rods (Fig. 4a and b)36 for compound 8.
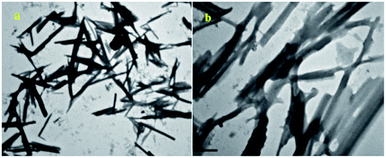 |
| Fig. 4 HR-TEM images of compound 8 (dissolved in dichloroethane) under the different magnification: (a) 1 μm and (b) 0.5 μm. | |
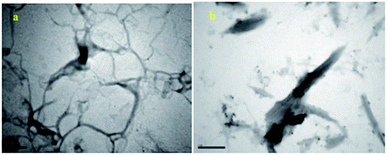 |
| Fig. 5 HR-TEM images of dried sample of compound 17 (dissolved in dichloroethane) under different magnifications: (a) 1 μm and (b) 0.5 nm. | |
3.5. FT-IR spectroscopic study
FTIR spectroscopy is a powerful tool to study non-covalent interactions, especially hydrogen bonding in self-assembled gelation. Infrared spectra of compound 8 in gel phase and in solution were recorded to reveal the interactions between gelators and solvents. From Fig. 6, in dichloroethane we could find that the stretching vibration band for NH was located at 3430–3440 cm−1, and the bands of 1627 and 1576 cm−1 were ascribed to the NH-I and NH-II bands. In gel phase, the stretch vibration band for NH split into three (3340, 3368 and 3309 cm−1) and shifted to lower wavenumber, while the NH-I and NH-II ones shifted to 1632 and 1577 cm−1, respectively. These spectral changes indicated the formation of hydrogen bonding between the acyl hydrazine groups. The FT-IR measurements also provided information on the alignment of alkyl groups. The absorption bands of the asymmetric and symmetric stretching vibrations of CH2 in 2 appeared at 2917 and 2850 cm−1 in THF solution, while in gel obtained from dichloroethane and toluene they shifted to 2919 and 2851 cm−1, respectively, which suggested that the alkyl groups of 8 were organized in the self-assembled nanostructure.37
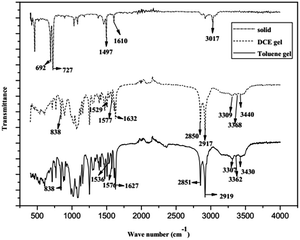 |
| Fig. 6 FTIR spectra of compound 8 (solid, Gel-I (dichloroethane), Gel-II (toluene)). | |
3.6 Rheological studies
The mechanical strengths of representative organogel were characterized by rheological experiments. Rheological studies give an indication of the flow behaviour and rigidity of the gel. The two main parameters are the storage modulus (G′), which represents the ability of the deformed material to store energy, and the loss modulus (G′′), which corresponds to the flow behaviour of the material under stress of gel 8 are shown in Fig. 7 as a function of angular frequency at a constant strain of 0.1%. Storage modulus (G′) was higher than the loss modulus (G′′) over the entire measured frequency range (1–400 rad s−1). Storage (G′) modulus was independent of the angular frequency, the rheological behaviour evidenced the viscoelastic nature of the gel.38
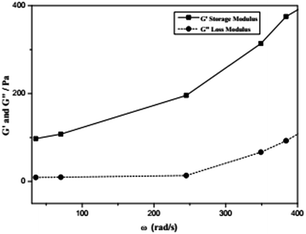 |
| Fig. 7 Angular frequency sweep (ω) dependencies of dynamic storage (G′) and loss modulus (G′′) of 0.3% w/v 8 gel in dichloroethane. | |
3.7. X-ray diffraction studies of gel
The self-assembly of the molecules in the gel state was studied using powder X-ray diffraction technique. The gel of long alkyl chain based benzohydrazide N-glycosylamine 8 was prepared at the concentration of 0.5% in dichloroethane and allowed to stand at ambient temperature to obtain the xerogel.39 A typical diffraction pattern of a two dimensional hexagonal lattice with one very strong diffraction peak at lower angle and two much weaker diffraction peaks were obtained. A diffraction pattern with a d-spacing at 22.12 Å and a broad diffuse peak (4.34 Å and 4.03 Å) were observed, which is a typical angle for diffraction from partially disordered alkyl groups, and a peak around 2θ = 22° (4.03 Å) is characteristic of a typical π–π stacking distance at wide-angle region was obtained.40 From the powder XRD (Fig. 8) pattern it was confirmed that the self-assembly of gelator 8 was also due to van der Waals force between the alkyl chain and π–π interaction between the benzohydrazide core.
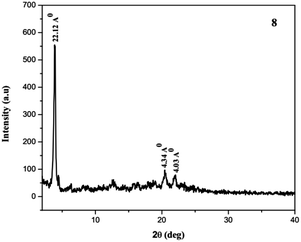 |
| Fig. 8 Powder X-ray diffraction of xerogel 8 (dichloroethane 0.5%). | |
The strong diffraction at a low 2θ angle of 3.88 corresponds to van der Waals force present in the compound and a broad peak at 21.99° arises from π–π interaction unit. Peak at 22.54° shows the crystalline nature of the gel rod.41 The crystallite size was calculated by using Debye–Scherrer equation; the average crystallite size of 10.72 nm was measured. The average dislocation density (6.383 × 1016 m−2) for the compound 8 was measured.
3.8. Thermal stability
The thermal properties of the long alkyl chain substituted benzohydrazide-N-glycosylamine 8 was understood from DSC studies. The DSC experiment was carried out for the compound in the solid state as well as in the gel state. The peaks observed in both cases were overlapped. The overlapped DSC graph is shown in Fig. 9. The melting heat peak of 8 observed at 175.14 °C with ΔH value of 26.87 J g−1 in the solid phase whereas the same compound in the gel state melted at 123.87 °C with the ΔH value of 10.32 J g−1. Generally, the ΔH value of the gel depend on the nature of the solvent used for gelation was well reported in literature.42 In general, for a good gelator the different should be too wide. Here, in this case the difference in the melting temperature (Tm) between the solid and gel was found to be exactly 48 °C. Hence, the gel formed from the compound 8 was found to be a good gelator.
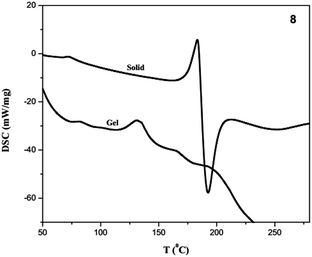 |
| Fig. 9 DSC spectra of compound 8 of 1,2-dichloethane 0.5%. | |
3.9. Photophysical studies
The UV-Vis absorption spectra of the long alkyl chain substituted benzohydrazide-N-glycosylamines (8) was investigated using absorption and emission spectroscopy techniques. The absorption spectra of the N-glycosylamine was recorded at the concentration of 5 × 10−5 M in different types of solvents i.e., polar (chloroform and methanol) and non-polar (tetrahydrofuran, cyclohexane, 1,2-dichloroethane, and toluene) solvents. The n–π* transition bands of 8 appeared at 266 and 285 in the non-aggregating solvent (dimethyl sulphoxide) and aggregating solvent (toluene) respectively which shows a blueshift. These blue-shift phenomena indicates that the n–π* stacking interaction between phenyl groups happened during the formation of the gel and the aryl groups were enforced to adopt an H-aggregation mode43 (face-to-face mode) (Fig. 10). The number of methylene units in the alkyl chain and the 4,6-O-protecting group in the D-glucose unit influenced the absorption maxima in the solution state and the gel state.44 Upon exciting the N-glycosylamine 8 at its absorption maxima of 256 nm, the fluorescence emission spectra of 8 in non-aggregating solvent and aggregating solvent appeared at 369 and 367 respectively as shown in Fig. 11.45
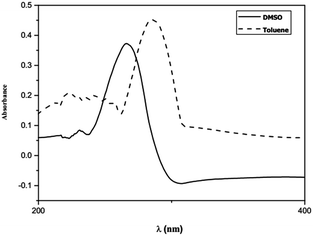 |
| Fig. 10 Absorption spectra of long alkyl chain substituted benzohydrazide-N-glycosylamines (8) (1 × 10−5 M) in non-aggregating solvent (dimethyl sulfoxide) and aggregating solvent (toluene). | |
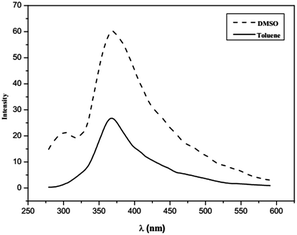 |
| Fig. 11 Emission spectra of long alkyl chain substituted benzohydrazide-N-glycosylamines (8) (1 × 10−5 M) in non-aggregating solvent (dimethyl sulfoxide) and aggregating solvent (toluene). Excitation wavelength is 250 nm. | |
4. Experimental section
4.1. General procedure for the synthesis of long alkyl chain based N-glycosylamines
To a solution of long alkyl chain substituted benzohydrazide (1–4) (1 mmol) in dry MeOH and 4,6-O-protected-D-glucopyranose (BGP, EGP, BzGP) (5–7) (1 mmol) were added. After stirring at room temperature for given period of time, the solvent was evaporated off under reduced pressure. The crude product was slurred with silica gel and purified by column chromatography.
4.2. Synthesis of N′-(4,6-O-ethylidene-β-D-glucopyranosyl-4-(octyloxy)benzohydrazide) (8)
Compound 8 was obtained by the reaction of 4,6-O-ethylidine-β-D-glucopyranose (1 mmol, 0.27 g), and 4-(octyloxy)benzohydrazide 1 (1 mmol, 0.31 g) in methanol as a colourless solid. Yield: 0.45 g (87%); Mp 150–152 °C. 1H NMR (CDCl3 + DMSO-d6): δ 9.48 (d, J = 3.6 Hz, 1H, –NH), 7.84 (d, J = 8.4 Hz, 2H, Ar-H), 6.89 (d, J = 8.4 Hz, 2H, Ar-H), 5.61 (s, 1H, Sac-H), 5.38 (s, 1H, Sac-H), 4.80 (d, J = 3.3 Hz, 1H, Sac-H), 4.69 (d, J = 4.8 Hz, 1H, Sac-H), 4.15–4.07 (m, 2H, Sac-H), 3.99 (t, J = 6 Hz, 2H, –OCH2), 3.65 (t, J = 3.0 Hz, 1H, Sac-H), 3.49 (t, J = 9 Hz, 1H, Ano-H), 3.31 (t, J = 12 Hz, 2H, Sac-H), 3.16 (d, J = 6 Hz, 1H, Sac-H) 1.78 (t, J = 5.1 Hz, 2H, –CH2), 1.45–1.29 (m, 13H, –CH2), 0.87 (s, 3H, –CH3) ppm. 13C NMR (CDCl3 + DMSO-d6): δ 167.1, 161.1, 128.9, 128.7, 123.8 (2C), 113.6, 98.8, 91.5, 79.9, 72.9, 71.4, 67.6, 67.6, 31.9, 28.7, 28.6, 28.5, 25.4, 22.9, 22.0, 19.9, 13.2 ppm. Anal. calcd for C23H36N2O7: C, 60.42; H, 7.98; N, 6.17.
4.2.1. Synthesis of N′-(4,6-O-butylidene-β-D-glucopyranosyl-4-(octyloxy)benzohydrazide) (9). Compound 9 was obtained by the reaction of 4,6-O-butylidine-β-D-glucopyranose (1 mmol, 0.63 g), and 4-(octyloxy)benzohydrazide (1) (1 mmol, 0.60 g) in methanol as a colourless solid. Yield: 0.92 g (88%); Mp 162–165 °C. 1H NMR (CDCl3 + DMSO-d6): δ 9.72 (s, 1H, –NH), 7.85 (d, J = 8.7 Hz, 2H, Ar-H), 6.89 (d, J = 8.7 Hz, 2H, Ar-H), 5.72 (s, 1H, Sac-H), 5.41 (d, J = 3 Hz, 1H, Sac-H), 5.04 (s, 1H, Sac-H), 4.83–4.67 (m, 1H, Sac-H), 4.51–4.43 (m, 1H, Sac_H), 4.12–4.07 (m, 2H, –CH2), 3.99 (t, J = 6 Hz, 2H, Sac-H), 3.77 (t, J = 6 Hz, 1H, Sac-H), 3.71–3.57 (m, 2H, Sac-H), 3.45 (d, J = 10.2, 1H, Ano-H), 1.77 (t, J = 6 Hz, 2H, –CH2), 1.59–1.54 (m, 2H, –CH2), 1.41 (t, J = 6 Hz, 2H, –CH2), 1.30 (d, J = 7.8 Hz, 10H, 7–-CH2), 0.91–0.86 (m, 3H, –CH3) ppm. 13C NMR (CDCl3 + DMSO-d6): δ 166.8, 161.3, 129.0, 129.0, 113.5 (2C), 101.6, 96.6, 91.7, 80.2, 72.9, 71.6, 67.8, 67.5, 61.5, 35.7, 31.1, 28.7, 28.6, 28.5, 25.4, 22.0, 16.8, 13.6, 13.5 ppm. Anal. calcd for C25H40N2O7: C, 61.85; H, 8.35; N, 5.81.
4.2.2. Synthesis of N′′-4,6-O-benzylidene-β-D-glucopyranosyl-4-(octyloxy)benzohydrazide (10). Compound 10 was obtained by the reaction of 4,6-O-benzylidine-β-D-glucopyranose (1 mmol, 0.32 g), and 4-(octyloxy)benzohydrazide 1 (1 mmol, 0.36 g) in methanol as a colourless solid. Yield: 0.42 g (73%); Mp 165–167 °C. 1H NMR (CDCl3 + DMSO-d6): δ 9.54 (d, J = 4.1 Hz, 1H, –NH), 7.85 (d, J = 8.7 Hz, 2H, Ar-H), 7.48 (d, J = 3.1 Hz, 2H, Ar-H), 7.33 (t, J = 6 Hz, 3H, Ar-H), 6.53 (t, J = 6 Hz, 2H, Ar-H), 5.64 (s, 1H, Sac-H), 5.52 (d, J = 8.7 Hz, 1H, Sac-H), 5.37 (J = 3 Hz, 1H, Sac-H), 5.00 (d, J = 3.6 Hz, 1H, Sac-H), 4.33–4.17 (m, 3H, Sac-h), 3.99 (t, J = 6 Hz, 3H, –OCH2), 3.72 (d, J = 7.2 Hz, 1H, Ano-H), 3.48 (t, J = 6 Hz, 2H, Sac-H), 1.41 (t, J = 6 Hz, 2H, –CH2), 0.93 (d, J = 7.5 Hz, 10H, –CH2), 0.50 (d, J = 6.6 Hz, 3H, –CH3) ppm. 13C NMR (CDCl3 + DMSO-d6): δ 172.7, 167.0, 142.5, 140.5, 134.4 (2C), 133.1, 131.5 (2C), 119.1, 119.0, 106.6, 97.28, 86.1, 83.1, 78.4, 76.8, 73.7, 73.2, 73.1, 36.7, 34.2 (2C), 34.1, 34.0, 30.9, 27.5, 19.1 ppm. Anal. calcd for C28H38N2O7: C, 64.63; H, 7.40; N, 5.41.
4.2.3. Synthesis of N′-4,6-O-ethylidene-β-D-glucopyranosyl-4-(dodecyloxy)benzohydrazide (11). Compound 11 was obtained by the reaction of 4,6-O-ethylidine-β-D-glucopyranose (1 mmol, 0.62 g), and 4-(dodecyloxy)benzohydrazide (2) (1 mmol, 0.45 g) in methanol as a colourless solid. Yield: 0.93 g (83%); Mp 158–160 °C. 1H NMR (CDCl3 + DMSO-d6): δ 9.91 (d, J = 3.2 Hz, 1H, –NH), 7.82 (d, J = 8.7 Hz, 2H, Ar-H), 6.90 (d, J = 8.4 Hz, 2H, Ar-H), 5.50 (s, 1H, Sac-H), 5.39 (s, 1H, sac-H), 4.70 (d, J = 5.0 Hz, 1H, Sac-H), 4.50 (d, J = 2.4 Hz, 1H, sac-H), 4.16–4.09 (m, 2H, –OCH2), 3.99 (t, J = 6 Hz, 2H, Sac-H), 3.70 (d, J = 8.7 Hz, 1H, Ano-H), 3.51 (t, J = 12 Hz, 1H, Sac-H), 3.40–3.26 (m, 3H, Sac-H), 1.80–1.74 (m, 2H, –CH2), 1.45 (t, J = 6 Hz, 2H, –CH2), 1.34 (d, J = 5.1 Hz, 6H, –CH2), 1.31–1.22 (m, 13H, –CH2), 0.87 (t, J = 6 Hz, 3H, –CH3) ppm. 13C NMR (CDCl3 + DMSO-d6): δ 172.6, 166.8, 134.0 (2C), 128.9 (2C), 118.9, 106.6, 104.2, 96.6, 86.0, 78.1, 76.4, 72.9 (2C), 36.5, 34.2, 34.2, 34.0, 33.9, 33.7, 30.6, 27.3, 25.0, 18.4 (2C) ppm. Anal. calcd for C27H44N2O7: C, 62.99; H, 8.69; N, 5.49.
4.2.4. Synthesis of N′-(4,6-O-butylidene-β-D-glucopyranosyl)-4-(dodecyloxy)benzohydrazide (12). Compound 12 was obtained by the reaction of 4,6-O-butylidine-β-D-glucopyranose (1 mmol, 0.32 g), and 4-(dodecyloxy)benzohydrazide 2 (1 mmol, 0.27 g) in methanol as a colourless solid. Yield: 0.36 g (75%); Mp 159–161 °C. 1H NMR (CDCl3 + DMSO-d6): δ 9.53 (s, 1H, –NH), 7.81 (d, J = 8.4 Hz, 2H, Ar-H), 6.87 (d, J = 8.4 Hz, 2H, Ar-H), 5.61 (s, 1H, Sac-H), 5.41 (s, 1H, Sac-H), 4.88 (s, 1H, Sac-H), 4.49 (d, J = 4.8 Hz, 2H, Sac-H), 4.10 (d, J = 9.9 Hz, 2H, Sac-H), 3.95 (d, J = 6.3 Hz, 2H, –O–CH2), 3.80 (t, J = 8 Hz, 1H, Ano-H), 3.72–3.60 (m, 1H, Sac-H), 3.49–3.41 (m, 2H, Sac-H), 1.75 (t, J = 6 Hz, 2H, –CH2), 1.56 (d, J = 5.1 Hz, 3H, –CH2), 1.39 (t, J = 6 Hz, 5H, –CH2), 1.34–1.23 (m, 14H, –CH2), 0.85 (t, J = 6 Hz, 6H, –CH3) ppm. 13C NMR (CDCl3 + DMSO-d6): δ 172.2, 166.7, 134.1, 129.5 (2C), 118.8, 106.9, 96.8, 85.3, 83.2, 79.7, 78.1, 76.7, 73.0, 72.8, 41.1, 40.9, 36.5, 36.2, 34.2, 34.2, 34.0, 33.9, 33.7, 30.6, 27.3, 22.1, 18.9, 18.7 ppm. Anal. calcd for C29H48N2O7: C, 64.38; H, 8.97; N, 5.20.
4.2.5. Synthesis of N-4,6-O-benzylidene-β-D-glucopyranosyl-4-(dodecyloxy)benzohydrazide (13). Compound 13 was obtained by the reaction of 4,6-O-benzylidine-β-D-glucopyranose (1 mmol, 0.32 g), and 4-(dodecyloxy)benzohydrazide (2) (1 mmol, 0.36 g) in methanol as a colourless solid. Yield: 0.42 g; Mp 158–160 °C. 1H NMR (CDCl3 + DMSO-d6): δ 8.99 (s, 1H, –NH), 7.81 (s, 2H, Ar-H), 7.47 (s, 2H, Ar-H), 7.33 (s, 3H, Ar-H), 6.90 (s, 2H, Ar-H), 5.48 (t, J = 9 Hz, 2H, Ano-H), 5.29 (s, 1H, Sac-H), 4.25 (d, J = 27 Hz, 3H, Sac-H), 3.98 (d, J = 6 Hz, 2H, Sac-H), 3.77 (t, J = 6 Hz, 2H, Sac-H), 3.48 (s, 3H, Sac-H), 2.15 (d, J = 6 Hz, 2H, –CH2), 1.77 (s, 2H, –CH2), 1.43–1.26 (m, 16H, –CH2), 0.86 (d, J = 4.2 Hz, 3H, –CH3) ppm. 13C NMR (CDCl3 + DMSO-d6): δ 172.4, 162.0, 137.2, 129.2 (2C), 128.9 (2C), 128.0, 126.2, 124.3 (2C), 114.0, 101.5, 91.8, 86.3, 81.4, 80.8, 73.2, 71.6, 68.0, 31.6, 29.4 (2C), 29.3, 29.1, 29.1 (2C), 28.9, 25.7, 22.4, 13.9 ppm. Anal. calcd for C32H46N2O7: C, 66.59; H, 8.08; N, 4.90.
4.2.6. Synthesis of N′-4,6-O-ethylidene-β-D-glucopyranosyl-4-(hexadecyloxy)benzohydrazide (14). Compound 14 was obtained by the reaction of 4,6-O-ethylidine-β-D-glucopyranose (1 mmol, 0.62 g), and 4-(hexadecyloxy)benzohydrazide (3) (1 mmol, 0.42 g) in methanol as a colourless solid. Yield: 0.66 g (73%); Mp 156–158 °C. 1H NMR (CDCl3 + DMSO-d6): δ 9.35 (s, 1H, –NH), 7.83 (d, J = 8.7 Hz, 2H, Ar-H), 6.90 (d, J = 8.4 Hz, 2H, Ar-H), 5.56 (s, 1H, Sac-H), 5.41 (s, 1H, Sac-H), 4.66 (d, J = 2.7 Hz, 1H, Sac-H), 4.53 (t, J = 3 Hz, 1H, Sac-H), 4.1 (t, J = 9 Hz, 2H, Sac-H), 4.00 (t, J = 3 Hz, 2H, –O–CH2), 3.68 (d, J = 8.7 Hz, 1H, Sac-H), 3.50 (t, J = 9 Hz, 1H, Ano-H), 3.36–3.24 (m, 2H, Sac-H), 1.79 (t, J = 6 Hz, 2H, –CH2), 1.61 (d, J = 5.1 Hz, 2H, –CH2), 1.41 (t, J = 6 Hz, 4H, –CH2), 1.39–1.21 (m, 24H, –CH2), 0.96 (t, J = 6 Hz, 3H, –CH3) ppm. 13C NMR (CDCl3 + DMSO-d6): δ 172.4, 166.7, 156.2, 134.1, 129.7, 118.8, 107.0, 96.7, 85.1, 82.9, 78.1, 76.6, 72.9, 40.9, 36.5 (2C), 34.2 (2C), 34.0, 33.9, 33.7, 30.6 (2C), 27.3 (2C), 22.1, 18.8, 18.6 (2C) ppm. Anal. calcd for C31H52N2O7: C, 65.27; H, 9.23; N, 4.94.
4.2.7. Synthesis of N′-(4,6-O-butylidene-β-D-glucopyranosyl)-4-(hexadecyloxy)benzohydrazide (15). Compound 15 was obtained by the reaction of 4,6-O-butylidine-β-D-glucopyranose (1 mmol, 0.32 g), and 4-(hexadecyloxy)benzohydrazide 3 (1 mmol, 0.24 g) in methanol as a colourless solid. Yield: 0.33 g (68%); Mp 159–161 °C. 1H NMR (CDCl3 + DMSO-d6): δ 9.35 (s, 1H, –NH), 7.84 (d, J = 8.4 Hz, 2H, Ar-H), 6.91 (d, J = 8.4 Hz, 2H, Ar-H), 5.54 (s, 1H, Sac-H), 5.44 (s, 1H, Sac-H), 4.66 (d, J = 2.7 Hz, 2H, Sac-H), 4.53 (t, J = 4.8 Hz, 1H, Sac-H), 4.14 (t, J = 6 Hz, 2H, –OCH2), 4.00 (t, J = 6 Hz, 2H, Sac-H), 3.68 (d, J = 8.7 Hz, 1H, Sac-H), 3.50 (t, J = 12 Hz, 1H, Ano-H), 3.47–3.24 (m, 3H, Sac-H), 1.79 (t, J = 6 Hz, 2H, –CH2), 1.63 (d, J = 5.1 Hz, 2H, –CH2), 1.46–1.32 (m, 27H, –CH2), 0.92 (d, J = 6 Hz, 6H, –CH3) ppm. 13C NMR (CDCl3 + DMSO-d6): δ 172.4, 166.7, 134.1 (2C), 129.0, 118.8 (2C), 107.0, 96.7, 85.1, 78.1, 76.6, 73.0, 72.9, 72.8, 40.9, 36.5, 34.3 (5C), 34.2, 34.0, 33.9, 33.7, 30.6 (2C), 27.3, 22.1 (2C), 18.7, 18.6 ppm. Anal. calcd for C33H56N2O7: C, 66.39; H, 9.48; N, 4.91.
4.2.8. Synthesis of N-4,6-O-benzylidene-β-D-glucopyranosyl-4-(hexadecyloxy)benzohydrazide (16). Compound 16 was obtained by the reaction of 4,6-O-benzylidine-β-D-glucopyranose (1 mmol, 0.31 g), and 4-(hexadecyloxy)benzohydrazide 3 (1 mmol, 0.27 g) in methanol as a colourless solid. Yield: 0.36 g (70%); Mp 158–160 °C. 1H NMR (CDCl3 + DMSO-d6): δ 9.18 (d, J = 3.9 Hz, 1H, –NH), 7.27 (d, J = 7.2 Hz, 2H, Ar-H), 6.88 (d, J = 3.6 Hz, 2H, Ar-H), 6.75 (d, J = 3 Hz, 3H, Ar-H), 6.32 (d, J = 8.1 Hz, 2H, Ar-H), 5.17 (s, 1H, Sac-H), 4.91 (t, J = 9 Hz, 2H, Ano-H), 4.67 (d, J = 3.9 Hz, 1H, Sac-H), 3.67 (d, J = 9.3 Hz, 1H, Sac-H), 3.57 (d, J = 9 Hz, 1H, Sac-H), 3.43–3.36 (m, 3H, Sac-H), 3.30–3.18 (m, 2H, Sac-H), 2.78–2.71 (m, 2H, Sac-H), 1.13 (d, J = 6.6 Hz, 2H, –CH2), 1.16 (s, 2H, –CH2), 0.86–0.67 (m, 24H, –CH2), 0.28 (t, J = 6 Hz, 3H, –CH3) ppm. 13C NMR (CDCl3 + DMSO-d6): δ 172.0, 166.4, 155.2, 140.3 (2C), 134.2, 133.7 (2C), 132.8 (2C), 131.2, 118.8 (2C), 106.2, 96.9, 85.3, 78.1, 77.9, 72.8, 36.5 (2C), 34.2, 34.0 (6C), 33.9 (2C), 33.7, 30.6 (2C), 27.2 (2C), 18.9 ppm. Anal. calcd for C36H54N2O7: C, 68.70; H, 8.66; N, 4.45.
4.2.9. Synthesis of N′-4,6-O-ethylidene-β-D-glucopyranosyl-4-(triethylene glycol monomethyl ether) benzohydrazide (17). Compound 17 was obtained by the reaction of 4,6-O-benzylidine-β-D-glucopyranose (1 mmol, 0.31 g), and 4-(mono methyl glycol)benzohydrazide 4 (1 mmol, 0.27 g) in methanol as a colourless solid. Yield: 0.36 g (70%); Mp 158–160 °C. 1H NMR (CDCl3 + DMSO-d6): δ 8.93 (s, 1H, –NH), 7.81 (d, J = 8.1 Hz, 2H, Ar-H), 6.93 (d, J = 8.1 Hz, 2H, Ar-H), 5.48 (d, J = 8.6 Hz, 1H, Ano-H), 4.72–4.76 (m, 2H, Sac-H), 4.13 (d, J = 15.3 Hz, 6H, –OCH2), 3.87 (d, J = 3.3 Hz, 4H, Sac-H), 3.73 (d, J = 4.5 Hz, 3H, Sac-H), 3.60–3.65 (m, 3H, –OCH2), 3.54 (t, J = 6.6 Hz, 4H, –OCH2), 3.37 (s, 3H, –OMe), 1.35 (s, 3H, –CH3) ppm. 13C NMR (CDCl3 + DMSO-d6): δ 167.9, 160.0, 129.2, 124.4 (2C), 114.3, 99.4, 92.9, 91.6, 80.6, 80.0, 73.4, 71.9, 70.7, 70.4, 70.4, 69.4, 68.1, 67.5, 58.8, 20.2 ppm. Anal. calcd for C22H34N2O10: C, 54.20; H, 7.02; N, 5.75.
4.2.10. Synthesis of N′-(4,6-O-butylidene-β-D-glucopyranosyl)-4-(triethylene glycol mono methyl ether) benzohydrazide (18). Compound 18 was obtained by the reaction of 4,6-O-benzylidine-β-D-glucopyranose (1 mmol, 0.31 g), and 4-(mono methyl glycol)benzohydrazide 4 (1 mmol, 0.27 g) in methanol as a colourless solid. Yield: 0.36 g (70%); Mp 158–160 °C. 1H NMR (CDCl3 + DMSO-d6): δ 9.45 (s, 1H, –NH), 7.84 (d, J = 6.3 Hz, 2H, Ar-H), 6.93 (d, J = 6.3 Hz, 2H, Ar-H), 5.45 (t, J = 9 Hz, 1H, Ano-H), 4.53 (d, J = 3.9 Hz, 2H, Sac-H), 4.34 (s, 4H, –OCH2), 3.87 (s, 4H, Sac-H), 3.63 (t, J = 18 Hz, 3H, –OCH2), 3.55 (s, 3H, Sac-H, –OCH2), 3.47 (d, J = 10.5 Hz, 4H, –OCH2), 3.36 (s, 5H, Sac-H, –OMe), 1.61 (s, 2H, –CH2), 1.42 (t, J = 6 Hz, 2H, –CH2), 0.91 (d, J = 6.9 Hz, –CH3) ppm. 13C NMR (CDCl3 + DMSO-d6): δ 167.7, 161.6, 129.2, 124.3 (2C), 114.1 (2C), 102.1, 91.7, 80.2, 73.3, 71.6, 71.5, 70.6, 70.3, 70.2, 69.3, 68.2, 67.4, 58.6, 36.0, 36.0, 17.2, 14.3 ppm. Anal. calcd for C24H38N2O10: C, 55.85; H, 7.42; N, 5.42.
4.2.11. Synthesis of N′′-4,6-O-benzylidene-β-D-glucopyranosyl-4-4-(triethylene glycol mono methyl ether)benzohydrazide (19). Compound 19 was obtained by the reaction of 4,6-O-benzylidine-β-D-glucopyranose (1 mmol, 0.31 g), and 4-(mono methyl glycol) benzohydrazide (4) (1 mmol, 0.27 g) in methanol as a colourless solid. Yield: 0.36 g (70%); Mp 158–160 °C. 1H NMR (CDCl3 + DMSO-d6): δ 8.11 (s, 1H, –NH), 7.72 (d, J = 8.7 Hz, Ar-H), 7.45 (d, J = 3.6 Hz, 2H, Ar-H), 7.31 (t, J = 0 Hz, 3H, Ar-H), 6.90 (d, J = 8.4 Hz, 2H, Ar-H), 5.46 (d, J = 9.8 Hz, 1H, Ano-H), 4.27–4.11 (m, 4H, Sac-H), 3.84 (t, J = 3 Hz, 3H, Sac-H), 3.71–3.76 (m, 9H, –OCH2), 3.53 (t, J = 3 Hz, 3H, Sac-H), 3.46 (t, J = 9 Hz, 3H, –OCH2), 3.36 (s, 3H, –OMe) ppm. 13C NMR (CDCl3 + DMSO-d6): δ 172.1, 166.3, 142.3, 134.2, 133.7 (2C), 132.8, 131.2, 131.1, 129.6 (2C), 118.9, 106.3, 97.0, 85.9, 78.1, 76.7, 76.5, 75.4, 75.2, 75.1, 74.2, 73.3, 72.9, 72.3, 63.5 ppm. Anal. calcd for C27H36N2O10: C, 58.87; H, 6.58; N, 5.09.
5. Conclusion
In conclusion we have synthesized a series of sugar-benzohydrazide derivatives and characterized using different spectroscopic techniques. It is found that the synthesized derivatives can form stable gels in organic solvents through hydrogen bonding and van der Waals interaction. In different solvents, the organogelators are able to self-assemble into various architectures, such as rod and textured spheroids networks.
Acknowledgements
The authors acknowledge SERC-DST, New Delhi, India for financial support. T. M. acknowledges Central University of Tamil Nadu (CUTN), Thiruvarur, Tamil Nadu for infrastructure facilities. K. S. thanks acknowledge Central University of Tamil Nadu (CUTN), Thiruvarur for research fellowship and R. P. acknowledge SERC-DST for research fellowship.
References
- Y. F. Zhou and D. Y. Ya, Chem. Commun., 2009, 1172 RSC.
- C. Wang, X. Wang and X. Zhang, Acc. Chem. Res., 2012, 45, 608 CrossRef CAS PubMed.
- S. Yagai, Bull. Chem. Soc. Jpn., 2015, 88, 28 CrossRef.
- H. Adachi, Y. Hirai, T. Ikeda, M. Maeda, R. Hori, S. Kutsumizu and T. Haino, Org. Lett., 2016, 18, 924 CrossRef CAS PubMed.
- X. M. Li, J. Y. Li, Y. A. Gao, Y. J. Kuang, F. Shi and B. Xu, J. Am. Chem. Soc., 2010, 132, 17707 CrossRef CAS PubMed.
- A. Vintiloiu and J.-C. Leroux, J. Controlled Release, 2008, 125, 179 CrossRef CAS PubMed.
- A. Ajayaghosh, V. K. Praveen, C. Vijayakumar and S. J. George, Angew. Chem., Int. Ed., 2007, 46, 6260 CrossRef CAS PubMed.
- K. M. Aoki, T. Nishi, A. Ikeda, S. Shinkai and K. Murata, Chem. Commun., 1991, 1715 Search PubMed.
- J. J. D. de Jong, L. N. Lucas, R. M. Kellogg and J. H. Van Esch, Science, 2004, 304, 278 CrossRef CAS PubMed.
- A. Baral, S. Roy, S. Ghosh, D. Hermida-Merino, I. W. Hamley and A. Banerjee, Langmuir, 2016, 32(7), 1836 CrossRef CAS PubMed.
- S. S. Babu, V. K. Praveen and A. Ajayaghosh, Chem. Rev., 2013, 114, 11973 Search PubMed.
- E. L. Bonifazi, V. C. Edelsztein, G. O. Menendez, C. S. Lopez, C. C. Spagnuolo and P. H. D. Chenna, ACS Appl. Mater. Interfaces, 2014, 6, 8933 CAS.
- C. Y. Bao, R. Lu, M. Jin, P. C. Xue, C. H. Tan, Y. Y. Zhao and G. F. Liu, Carbohydr. Res., 2004, 339, 1311 CrossRef CAS PubMed.
- P. C. Xue, R. Lu, Y. Huang, M. Jin, C. H. Tan, C. Y. Bao, Z. M. Wang and Y. Y. Zhao, Langmuir, 2004, 20, 6470 CrossRef CAS PubMed.
- P. C. Xue, R. Lu, D. M. Li, M. Jin, C. Y. Bao, Y. Y. Zhao and Z. M. Wang, Chem. Mater., 2004, 16, 3702 CrossRef CAS.
- R. Maheswari, J. Manjula, S. Veeramanikandan and H. Benita Sherine, Chem. Sin., 2014, 5(6), 42 Search PubMed.
- C. Zhou, W. Gao, K. Yang, L. Xu, J. Ding, J. Chen, M. Liu, X. Huang, S. Wang and H. Wu, Langmuir, 2013, 29, 13568 CrossRef CAS PubMed.
- H. M. Zaid and R. T. Mohd, Asian J. Sci. Res., 2011, 2(11), 587 Search PubMed.
- M. Ikeda, T. Tanida, T. Yoshii and I. Hamachi, Adv. Mater., 2011, 23, 2819 CrossRef CAS PubMed.
- M. B. Dowling, R. Kumar, M. A. Keiblar, J. R. Hess, G. V. Bochicchio and S. R. Raghavan, Biomaterials, 2011, 32, 3351 CrossRef CAS PubMed.
- W. Edwards and D. K. Smith, J. Am. Chem. Soc., 2013, 135, 5911 CrossRef CAS PubMed.
- A. Pal, R. D. Mahapatra and J. Dey, RSC Adv., 2014, 4, 7760 RSC.
- C. Tan, L. Su, R. Lu, P. Xue, C. Bao, X. Liu and Y. Zhao, J. Mol. Liq., 2006, 124, 32 CrossRef CAS.
- R. Barkar and D. L. MacDonald, J. Am. Chem. Soc., 1960, 82, 2301 CrossRef.
- P. L. Mellies, C. L. Mehltretter and E. C. Rist, J. Am. Chem. Soc., 1951, 73, 294 CrossRef.
- N. Goyal, H. P. R. Mangunuru, B. Parikh, S. Shrestha and G. Wang, J. Org. Chem., 2014, 10, 3111 Search PubMed.
- R. Rajaganesh, A. Gopal, T. Mohan Das and A. Ajayaghosh, Org. Lett., 2012, 14, 748 CrossRef CAS PubMed.
- M. K. Dhinalkaran and T. Mohan Das, Org. Biomol. Chem., 2012, 10, 2077 Search PubMed.
- G. Zhu and J. S. Dordick, Chem. Mater., 2006, 18, 5988 CrossRef CAS.
- S. Nagarajan, T. Mohan Das, P. Arjun and N. Raaman, J. Mater. Chem., 2009, 19, 4587 RSC.
- F. Zhang, G. Li, W. Zhang and Y. L. Yan, Inorg. Chem., 2015, 54, 7325 CrossRef CAS PubMed.
- Z. Chen, Q. Zhao, M. Xu, L. Li, J. Duan and H. Zhu, Electrochim. Acta, 2015, 186, 117 CrossRef CAS.
- H. Cao, F. Wang, H. Zeng, R. Gong and H. Xin, J. Mol. Liq., 2014, 196, 94 CrossRef CAS.
- T. Kar, S. K. Mandal and P. K. Das, Chem.–Eur. J., 2011, 17, 14952 CrossRef CAS PubMed.
- Q. Zhou, T. Chen, J. Zhang, L. Wan, P. Xie, C. C. Han, S. Yan and R. Zhang, Tetrahedron Lett., 2008, 49, 5522 CrossRef CAS.
- S. N. Park, Y. S. Lee and B. H. Kim, Chem. Commun., 2003, 2912 RSC.
- T. Kar, S. Mukherjee and P. K. Das, New J. Chem., 2014, 38, 1158 RSC.
- H. Wang, H. Wu, C.-J. Lee, X. Lei, J. Zhe, F. Xu, F. Cheng and G. Cheng, Langmuir, 2016, 32, 6544 CrossRef CAS PubMed.
- M. K. Dhinalkaran, K. Soundarajan and T. Mohan Das, New J. Chem., 2014, 38, 2874 RSC.
- B. Bai, X. Maoa, J. Wei, Z. Wei, H. Wanga and M. Li, Sens. Actuators, B, 2015, 211, 268 CrossRef CAS.
- T. S. Hu, K. T. Lin, C. C. Mu, H. M. Kuo, M. C. Chen and C. K. Lai, Tetrahedron, 2014, 70, 9204 CrossRef CAS.
- A. Hemamalini and T. Mohan Das, RSC Adv., 2014, 4, 41010 RSC.
- P. Choudhury, D. Mandal, S. Brahmachari and P. K. Das, Chem.–Eur. J., 2016, 22, 5160 CrossRef CAS PubMed.
- M. K. Dhinakaran, K. Soundarajan and T. Mohan Das, New J. Chem., 2014, 38, 4371 RSC.
- X. Yang, R. Lu, H. Zhou, P. Xue, F. Wang, P. Chen and P. Zhao, J. Colloid Interface Sci., 2009, 339, 527 CrossRef CAS PubMed.
Footnote |
† Electronic supplementary information (ESI) available. See DOI: 10.1039/c6ra18715c |
|
This journal is © The Royal Society of Chemistry 2016 |