DOI:
10.1039/C6RA16905H
(Communication)
RSC Adv., 2016,
6, 80986-80993
Cheese-like bulk carbon with nanoholes prepared from egg white as an anode material for lithium and sodium ion batteries
Received
1st July 2016
, Accepted 11th August 2016
First published on 12th August 2016
Abstract
Cheese-like bulk carbon with nanoholes has been successfully fabricated from egg white via a simple annealing method by using distilled water as a green clean “corrosive agent”. X-ray diffractions and SEM images show the decomposition product of boiled egg white after annealing is bulk carbon, containing NaCl and KCl with a trace amount of nitrogen doping. After ultrasonic washing and centrifugation, the distilled water removed the NaCl and KCl nanocrystals from the bulk carbon completely and retained empty spaces, which eventually leads to the formation of a cheese-like structured bulk carbon with nanoholes. Our electrochemical tests show this cheese-like bulk carbon with nanoholes has a high specific capacity and good cycling performance and rate stability when evaluated as an anode material for lithium-ion batteries. Meanwhile, the electrochemical performances as an anode material for a sodium-ion battery are also displayed for comparison.
1. Introduction
Advanced lithium-ion battery (LIB) technology has permeated portable electronics and has an important role in large-scale applications such as electric vehicles and stationary grid storage.1,2 Extensive studies have been carried out to develop new high-capacity materials for higher-energy LIBs.3,4 However, LIBs store electrical energy in electrodes through intercalation or insertion of lithium, which are prepared from limited Li-containing mineral resources. As the use of LIBs becomes widespread, increasing demand for Li commodity chemicals combined with geographically constrained Li mineral reserves will drive up the prices of LIBs. Therefore, as an alternative technology, room-temperature sodium-ion battery (SIB), which is originally investigated in parallel with Li-ion batteries, have again aroused intensive interest recently for large-scale stationary energy storage in the applications of renewable energy and smart grid because of their low cost and the infinite Na resources (the fourth most abundant element in the earth's crust).5–8 However, both for Li-ion and Na-ion batteries, the poor electronic transport properties and huge volume change for materials including transition metal oxide and alloy compound hamper the applicability of them when used as anode materials.9 Fortunately, it has been widely acknowledged that nanosized materials to improve the specific surface provides higher electrochemical performance both in buffering the local volume change and increasing the contact area between the electrode and electrolyte,10–14 meanwhile, carbon coating on the surface of materials has also been a mature and effective method to improve the electronic conductivity. However, the problems come are that harsh optimization conditions for materials limit their extensive use in commercialization. Therefore, carbon is still the dominant electrode material for commercial LIB anodes due to its high electrical conductivity, low cost and long cycling life.15–18 That is to say, to find a new potential carbon material with good electrochemical performance as anode for lithium ion or sodium ion battery is still a very meaningful work on the current situation.
Utilizing sustainable biomass for the energy applications has received much attention in the scarcity of fossil energy. Lignin (rich in paper pulp sludge) and alginate extracted from brown algae have found interesting application in LIBs.19,20 However, proteins, another main component of biomass, are not being widely concerned on the application of energy storage at present, in our daily life, there are large quantities of protein-rich biomasses available, such as beer beverage and food industry byproducts and seaweed. Millions of chicken eggs are employed to cultivate various antibodies from the egg yolk or to extract “all natural” chemicals for anti-microbial and cosmetic industries.21 This process generates large quantities of nonedible chicken egg-based wastes, therefore, the works about these egg-based wastes on the applications of environment friendly and value-added green energy is very valuable.
In this paper, we employ egg white as a model protein-based precursor, use distilled water as a green clean “corrosive agent” and a simple annealing method for industrial production to obtain cheese-like bulk carbon with nanoholes. To demonstrate our approach, the egg white was annealing at a high temperature in reducing atmosphere, then ultrasonic washing the decomposition product in distilled water, and after centrifugation and drying we achieved cheese-like bulk carbon with nanoholes. Our electrochemical tests show this cheese-like bulk carbon with nanoholes have a high specific capacity and good performance in cycling and rate stability when evaluated as anode material for Li-ion battery compared to the Na-ion anode. Most importantly, because of its good electronic conductivity and structural stability, it also has the hope to be able to combine with other nanosize materials which as a bearer frame to improve electrochemical performance jointly in future work.
2. Experimental
2.1. Synthesis of cheese-like bulk carbon with nanoholes
The cheese-like bulk carbon with nanoholes was prepared by a simple three-step synthesis method which can be described as follows. For the first step, placed the egg in distilled water then heated it from room temperature (25 °C) to 100 °C with keeping for 15 min. For the second step, peeled the boiled egg white and sintered it at 600 °C for 5 h in an ambience of Ar/H2 (70/30 in volume percent) in quartz tube furnace to obtain the decomposition product. For the third step, ultrasonic washed the decomposition product by distilled water and then centrifugal separated at a speed of 14
000 rpm to get black precipitations, another same twice washing and centrifugation treatments were again for this black precipitation to obtain the final cheese-like bulk carbon with nanohole products.
2.2. Physical characterization
The X-ray diffraction data of cheese-like bulk carbon with nanohole product was collected by Bruker D8 advance diffractometer with Cu Kα radiation. A graphite monochromator was used for diffracted beams, and a step scan mode was adopted with a scanning step of 0.02. SEM images of the porous carbon were obtained by Nova NanoSEM 430 (FEI, USA) scanning electron microscopy (SEM), and energy-dispersive X-ray (EDX) analysis was performed by the EDX system attached to the same electron microscope. The centrifugal process is using the Allegra X-22 Centrifuge (Beckman Coulter, USA). N2 adsorption/desorption isotherms were measured at 77 K with the QUADRASORB SI-MP (Quantachrome). X-ray photo-electron spectroscopy (XPS) was obtained for the samples using a Kratos Axis Ultra spectrometer.
2.3. Electrochemical testing
The electrochemical properties of these porous carbon products as anode materials were evaluated with two-electrode electrochemical cell by an automatic battery tester system (Land®, China). The anode films were prepared by mixing the as-synthesis sample with acetylene black and polyvinylidene fluoride (PVDF) binder in a weight ratio of 8
:
1
:
1 in N-methyl-2-pyrrolidone (NMP). The obtained slurries were coated on Cu foil uniformly and the active materials loading on electrodes were 3 mg. Two-electrode electrochemical cells were assembled in a Mikrouna glove box filled with ultra-high argon. When as Li-ion battery anode, the lithium metal foils were used as counter electrodes, Celgard 2320 as separator, and 1 M LiPF6 in EC (ethylene carbonate)
:
DMC (dimethyl carbonate) (1
:
1 vol%) was used as electrolyte. When as Na-ion battery anode, the sodium metal foils were used as counter electrodes, a glass fiber as separator, and 1 M NaPF6 in EC
:
DMC (1
:
1 vol%) was used as electrolyte. Electrochemical impedance spectroscopy (EIS) was collected on an AUTOLAB PGSTAT302N (Metrohm, Netherlands). The impedance spectra were recorded by applying an ac voltage of 5 mV in the frequency range from 1 MHz to 0.01 Hz.
3. Results and discussion
In order to prevent oxidation and minimize the loss of carbon during the annealing process as far as possible, we choose the reducing atmosphere during our annealing process. Fig. 1a and b shows the SEM images and EDX analysis of the decomposition products of boiled egg white after annealing before (a) and after (b) ultrasonic washing. It is obviously that the dimensions of decomposition product particles are bulk structure in micron size and have a smooth surface before ultrasonic washing shown in Fig. 1a. The inset XRD pattern reveals that these products are bulk carbon containing NaCl and KCl. As a common sense, sodium and potassium are indispensable elements in the biological systems, therefore, after high temperature annealing these two elements crystallized out in the form of NaCl and KCl. The atomic percentages obtained from EDX examination are the existence evidence of Na, K and C elements, where the bright spots revealed in EDX mapping show the uniform distribution of Na, K and C. Taking into account the solubility of NaCl and KCl, the distilled water removed NaCl and KCl from the bulk carbon completely which playing a role of green clean “corrosive agent”, and a cheese-like structure bulk carbon is formed eventually after ultrasonic washing and centrifugation shown in Fig. 1b. According to the distribution of the nanoholes in the SEM image, NaCl and KCl crystallized out in nanosize particles and distributed uniformly in the bulk carbon after annealing. Combined with the inset XRD pattern and EDX examination in Fig. 1b, no NaCl and KCl remained in the bulk carbon, it believed that these nanoholes are throughout the whole bulk carbon not just only on the surface.
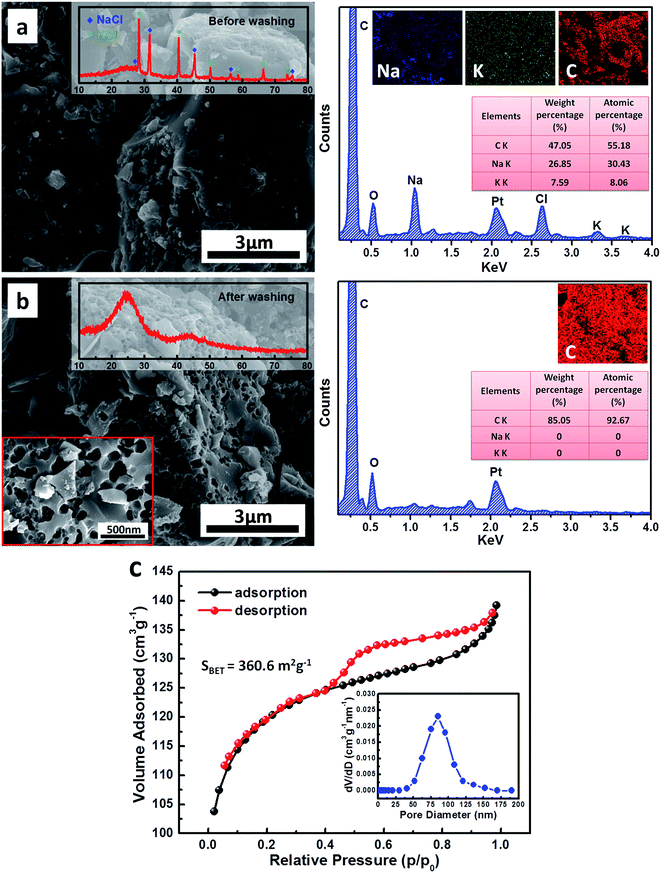 |
| Fig. 1 SEM images, XRD patterns and EDX analysis of the egg white after annealing before (a) and after (b) ultrasonic washing; (c) nitrogen adsorption–desorption isotherms and the corresponding pore size distribution (inset) for the cheese-like structure bulk carbon. | |
The porous feature of the carbon was further investigated by Brunauer–Emmett–Teller (BET) analysis via nitrogen adsorption and desorption measurement, and the corresponding Barrett–Joyner–Halenda (BJH) pore size distribution plot are as an inset shown in Fig. 1c. The nitrogen sorption isotherm reveals a typical type IV isotherm with H3-type loop hysteresis. The adsorption jump in the isotherm appears between the partial pressures (p/p0) of 0.5–1.0 due to the capillary condensation in the porous channels and/or cages. The pore size distribution curve shows that the as-prepared cheese-like structure bulk carbon has a main pore distribution from 40 to 150 nm with a mean pore size of 85.3 nm, which corroborates the SEM observations. The specific surface area of cheese-like structure bulk carbon is calculated to be 360.6 m2 g−1. This porous structure increases the specific surface enormously, not only buffering the local volume change, but also improve the ionic conductivity by increasing the contact area between the electrode and electrolyte which will greatly contribute to the improvement of electrochemical performance discussed in following.
Since the egg white is a protein-rich biomass, to confirm whether there are main characteristic elements of protein doping in the decomposition product after heat treatment is needed. Fig. 2 shows the XPS results of C, N, S, Na, K and Cl elements before (black frame) and after (green frame) ultrasonic washing after annealing process. It reveals that there is still trace amount of nitrogen existed in the bulk carbon after annealing process. The XPS of C 1s exhibits three peaks by curve fitting, the main peak at 284.8 eV is related to the graphite-like sp2 C (C1), which indicates that most of the C atoms in the bulk carbon are arranged in a conjugated honeycomb lattice. The two weak peaks located at 285.8 eV and 288.1 eV reflect the different bonding structures of the C–N bonds, corresponding to the N–sp2 C (C2) and N–sp3 C bonds (C3) respectively.22,23 The N 1s peak can also be split into three peaks which means nitrogen atoms in the prepared bulk carbon also exist in three states, namely, pyridine-like (N1), pyrrolic (N2) and graphitic (N3) nitrogen atoms, corresponding to binding energy peaks at 398.6 eV, 400.6 eV and 403.8 eV respectively.22,23 Combined with the above results of C 1s and N 1s, we can infer that the trace amount of N is doping in the carbon structure instead of a mixture of impurity.
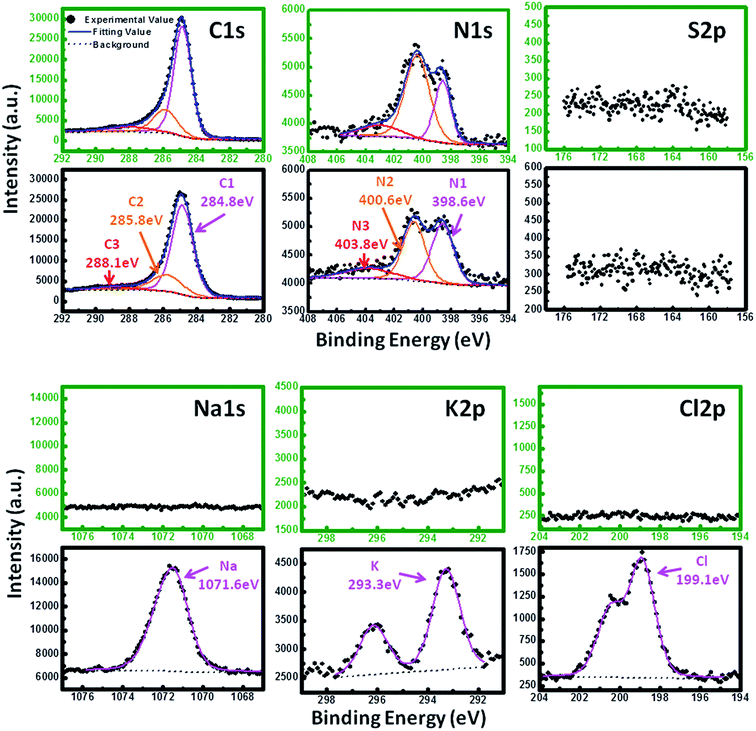 |
| Fig. 2 XPS of C, N, S, Na, K and Cl elements before (black frame) and after (green frame) ultrasonic washing. | |
Meanwhile, it is worth noting that there is no sulfur existed in the decomposition product from the results of XPS examination, it could attribute to pyrolysis release in the form of SOx during the annealing process. According to the XPS results of Na 1s (1071.6 eV), K 2p (292.3 eV) and Cl 2p (199.1 eV), the NaCl and KCl are removed from the bulk carbon completely after ultrasonic washing which is consistent with the EDX examination shown in Fig. 1b.
In order to describe the formation mechanism simply and vividly, we put forward a schematic diagram for this process shown in Fig. 3. When we placed the boiled egg white in a high temperature annealing for some time, the bulk carbon containing nanocrystal NaCl and KCl particles were obtained, which is similar to the jujube uniformly distributed in the jujube cake. After ultrasonic washing, the nanocrystal NaCl and KCl were removed by distilled water completely, and the places where they existed left empty spaces with nanosize which leads to the formation of cheese-like bulk carbon with nanoholes eventually.
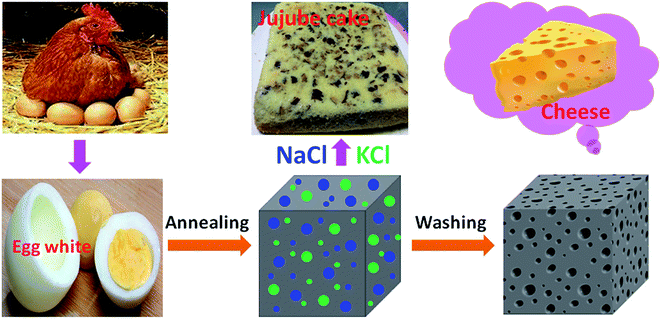 |
| Fig. 3 Schematic diagram for the formation of cheese-like bulk carbon. | |
Fig. 4a shows the typical discharge–charge curves for the first three cycles of cheese-like bulk carbon with nanoholes electrodes when as Li-ion and Na-ion anode materials. The first discharge and recharge capacities of 1183 mA h g−1 and 687.9 mA h g−1 (Li-ion anode), 386.1 mA h g−1 and 110.1 mA h g−1 (Na-ion anode) can be obtained during the first cycle, while the corresponding initial coulombic efficiencies (CEs) are 58.2% and 28.5%. The discharge capacities are larger than the recharge capacities for the first cycle, leading to the relatively poor initial CEs. This is a common drawback for these high-capacity anode materials caused by the great fading capacity between insertion and de-insertion process.9 It can be attributed to the side reactions such as formation of solid electrolyte interface (SEI) layer and irreversible reaction related to the Li and Na storage mechanism during the first insertion process discussed in Fig. 4e. After the first cycle, both of their CEs quickly increase and remain as nearly 100% thereafter, and the electrode reactions show a high reversibility as the third cycle can repeat well with the specific capacity of the second cycle for the two electrodes. From the second cycle, both of their discharge–charge capacities slowly decrease along the cycle number, after 100 cycles, charge capacities of ∼470 mA h g−1 (Li-ion anode) and ∼35 mA h g−1 (Na-ion anode) were obtained, with the capacity retentions of ∼72% and ∼31% compared to the second cycle shown in Fig. 4b. It is obviously that the electrochemical performance of Li-ion anode is much better than the Na-ion anode, this could be attributed to the smaller Li-ion radius with higher Li-ion conductivity compared to the Na-ion anode.
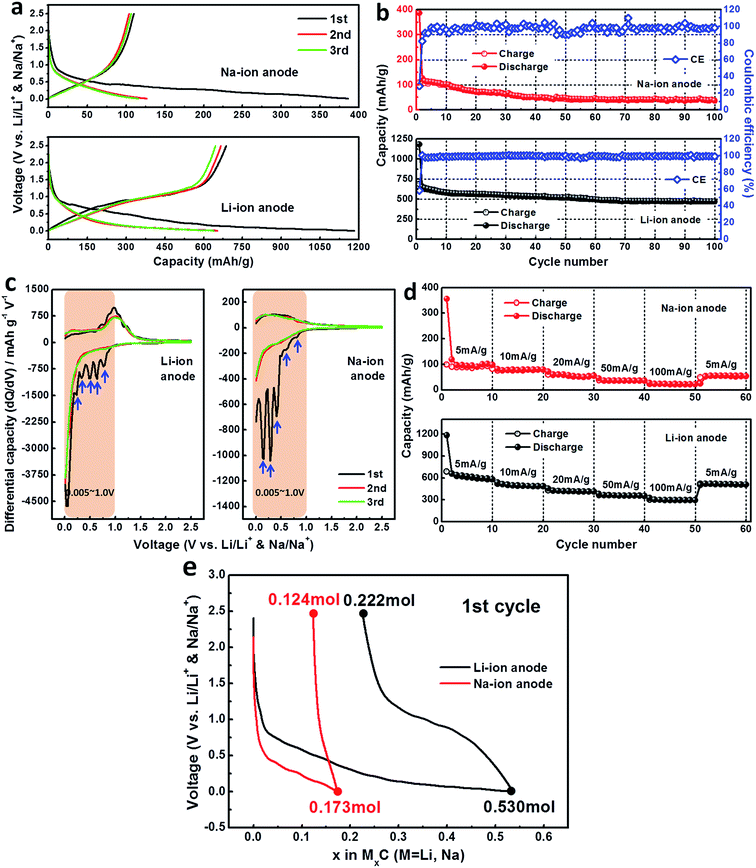 |
| Fig. 4 (a) The first three galvanostatic discharge/charge curves and (b) cycle performance with CEs at a current density of 5 mA g−1 between 0.005 and 2.5 V vs. Li/Li+ and Na/Na+; (c) EVS differential capacity data corresponding to (a); (d) rate abilities at various current densities; (e) amount of Li+/mol and Na+/mol insertion for the two electrodes during the first cycle. | |
The first three consecutive electrochemical voltages spectroscopy (EVS) differential capacity curves are shown in Fig. 4c. Differences of discharge EVS curves with that first cycling procedure are evidences for the existence of side reactions such as the formation of SEI layer and irreversible reactions which leading to the great fading capacity shown in Fig. 4a. The number of reduction peaks for the first insertion process is more than the following cycles during the mainly phase transformation between 0.005 V and 1.0 V. It is believed that more complex side reactions and irreversible reactions happened due to a higher surface activity caused by the high specific surface area of the carbon electrode.24,25 The oxidation peaks observed in EVS curves of the 1st–3rd cycle remain fairly consistent, indicating a similar de-insertion process, and the overlapping of EVS curves in subsequent cycles reveals good reversibility of electrochemical reactions for the two electrodes.
Fig. 4d shows the rate capability of them at various discharge/charge current densities. In the 1st–10th cycle, they have reversible discharge–charge capacities of ∼580 mA h g−1 (Li-ion anode) and ∼95 mA h g−1 (Na-ion anode) at 5 mA g−1 which are consistent with the cycling stability in Fig. 4b. As the current density changed from 100 mA g−1 to 5 mA g−1 after 50 cycles, considerable higher capacity ∼510 mA h g−1 can still be recovered for the Li-ion anode electrode compared to the Na-ion anode electrode (∼50 mA h g−1), indicating a stable rate as well as a good cycling capability.
Fig. 4e shows the amount of Li+/mol and Na+/mol insertion for the two electrodes during the first cycle. As we can see, a total of 0.530 mol Li+ and 0.173 mol Na+ per formula are inserted in the electrodes during the first insertion, while 0.222 mol Li+ and 0.124 mol Na+ are residual after de-insertion in the electrodes, which means a total of 0.308 mol Li+ and 0.049 mol Na+ per formula participate in the reversible reaction for the first cycle. According to the amount of Li+/mol and Na+/mol insertion for the two electrodes during the first cycle, the following equations are proposed for this cheese-like bulk carbon system:
|
C + 0.530Li+ + 0.530e− → Li0.53C
| (1) |
|
C + 0.173Na+ + 0.173e− → Na0.173C
| (2) |
|
Li0.53C → Li0.222C + 0.308Li+ + 0.308e−
| (3) |
|
Na0.173C → Na0.124C + 0.049Na+ + 0.049e−
| (4) |
|
Li0.222C + xLi+ + xe− ⇄ Li0.222+xC
| (5) |
|
Na0.124C + xNa+ + xe− ⇄ Na0.124+xC
| (6) |
A total of 0.530 mol Li+ and 0.173 mol Na+ per formula are inserted in the initial discharge process (eqn (1) and (2)) to form an structure of Li0.53C and Na0.173C corresponding to the actual capacity of 1183 mA h g−1 and 386.1 mA h g−1. When the electrodes material were recharged to 2.5 V, only 0.308 mol Li+ and 0.049 mol Na+ per formula extracted from the electrodes with a reversible capacity of 687.9 mA h g−1 and 110.1 mA h g−1 (eqn (3) and (4)). Meanwhile, two new phases of Li0.222C and Na0.124C were completely formed, and these two phases are responsible for the subsequent reversible discharge/charge cycles (eqn (5) and (6)). That is to say, the full discharged products Li0.53C and Na0.173C cannot be returned to the original carbon material completely by the first de-insertion process and the Li0.222C and Na0.124C are the final recharged products in our voltage window. These residual 0.222 mol Li+ and 0.124 mol Na+ in the electrodes after the first de-insertion contribute to the formation of SEI layer and irreversible reaction which are responsible for the large irreversible capacity loss and poor CEs in the first cycle shown in Fig. 4a and b.
To understand the electrochemical dynamic behavior of electrodes, electrochemical impedance spectroscopy (EIS) were measured after running three cycles with an applied voltage at 2.5 V (rest for 3 hours) shown in Fig. 5. The EIS curves for the electrodes when as anode for Li-ion and Na-ion batteries are similar and the inset is an equivalent electrical circuit model for analyzing impedance spectra in Fig. 5a. Each resistance Ri, Ri||CPEi and W components are sectioned as circuit elements (i) and (ii) with W (iii) which are attributed to regions shown in the Nyquist plots.26 The depressed semicircles are indicative of inhomogeneous nature of the electrode and this is accounted for by considering constant phase elements (CPEs) instead of pure capacitors in the equivalent circuit.27–29 Amplified region of high and intermediate frequency for the two electrodes is shown in Fig. 5b. The region (i) higher than 20 kHz represented by Re is attributed to the resistance of the electrolyte and cell components. The depressed semicircle observed in the intermediate frequency range (20 kHz to 31.6 Hz and 19.9 kHz to 12.6 Hz) is attributed to section (ii), which represents the charge transfer resistance Rct, and double layer capacitance CPEct. The straight line in the low-frequency region (<31.6 Hz and <12.6 Hz) is attributed to the diffusion of the lithium or sodium ions into the bulk of electrode material. It was clear to observe that the radius of the semicircle, at the intermediate frequency region for Li-ion electrode, is smaller than the Na-ion electrode which may indicate lower charge transfer impedance leading to a better electrochemical performance compared to the Na-ion anode electrode.
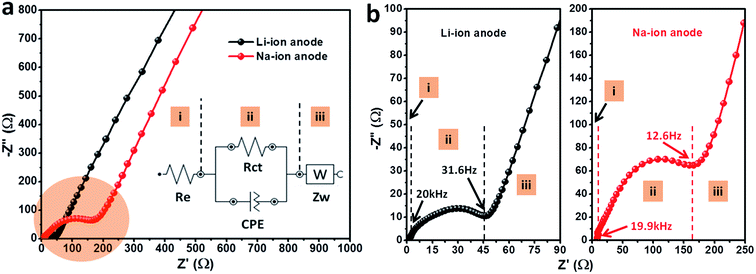 |
| Fig. 5 (a) EIS plots of the two electrodes after 3 cycles and relationship equivalent circuit used for fitting the experimental impedance spectra as inset; (b) the amplified region of high and intermediate frequency for the two electrodes. | |
4. Conclusion
Cheese-like bulk carbon with nanoholes has been successfully fabricated from egg white via a simple clean and environment friendly method. It was found that the decomposition products of boiled egg white after annealing are bulk carbon containing NaCl and KCl with trace amount of nitrogen doping before ultrasonic washing. The distilled water removed the uniformly distributed nanocrystal NaCl and KCl from the bulk carbon completely which is playing a role of green clean “corrosive agent” during the washing process. These porous structures increase the specific surface area of the carbon enormously, and improve the ionic conductivity by increasing the contact area between the electrode and electrolyte which greatly contribute to the improvement of electrochemical performance when evaluated as anode material although is not good enough for sodium-ion batteries. Meanwhile, the void space in the bulk carbon buffers the local volume change during Li-ion and Na-ion insertion–deinsertion to alleviate the problem of pulverization and aggregation of the electrode material. Most importantly, because of good electronic conductivity and structural stability of this cheese-like bulk carbon, it also has the hope to be able to combine with other nanosize materials as a bearer frame to improve the electrochemical performance jointly in future work.
Conflict of interest
The authors declare no competing financial interests.
Acknowledgements
This work was funded by NSFC Grant (No. 51372089, 51172077, 51373205) supported through NSFC Committee of China, and the Foundation of (No. 2014ZB0014) supported through the Fundamental Research Funds for the Central Universities.
References
- B. Dunn, H. Kamath and J. M. Tarascon, Electrical energy storage for the grid: a battery of choices, Science, 2011, 334, 928–935 CrossRef CAS PubMed.
- Z. G. Yang, J. L. Zhang, M. W. W. Kintner-Meyer, X. C. Lu, D. Choi, J. P. Lemmon and J. Liu, Electrochemical energy storage for green grid, Chem. Rev., 2011, 111, 3577–3613 CrossRef CAS PubMed.
- M. Armand and J. M. Tarascon, Building better batteries, Nature, 2008, 451, 652–657 CrossRef CAS PubMed.
- A. S. Aricò, P. Bruce, B. Scrosati, J. M. Tarascon and W. Schalkwijk, Nanostructured materials for advanced energy conversion and storage devices, Nature, 2005, 4, 366–377 CrossRef PubMed.
- Y. Sun, L. Zhao, H. Pan, X. Lu, L. Gu, Y. S. Hu, H. Li, M. Armand, Y. Ikuhara, L. Q. Chen and X. Huang, Direct atomic-scale confirmation of three-phase storage mechanism in Li4Ti5O12 anodes for room-temperature sodium-ion batteries, Nat. Commun., 2013, 4, 1870–1880 CrossRef PubMed.
- A. Hayashi, K. Noi, A. Sakuda and M. Tatsumisago, Superionic glass-ceramic electrolytes for room-temperature rechargeable sodium batteries, Nat. Commun., 2012, 3, 856–860 CrossRef PubMed.
- S. P. Ong, V. L. Chevrier, G. Hautier, A. Jain, C. Moore, S. Kim, X. Ma and G. Ceder, Voltage, stability and diffusion barrier differences between sodium-ion and lithium-ion intercalation materials, Energy Environ. Sci., 2011, 4, 3680–3688 CAS.
- S. Wenzel, T. Hara, J. Janek and P. Adelhelm, Room-temperature sodium-ion batteries: improving the rate capability of carbon anode materials by templating strategies, Energy Environ. Sci., 2011, 4, 3342–3345 CAS.
- M. V. Reddy, G. V. Subba Rao and B. V. R. Chowdari, Metal oxides and oxysalts as anode materials for Li ion batteries, Chem. Rev., 2013, 113, 5364–5457 CrossRef CAS PubMed.
- P. Poizot, S. Laruelle, S. Grugeon, L. Dupont and J. M. Tarascon, Nano-sized transition-metal oxides as negative-electrode materials for lithium-ion batteries, Nature, 2000, 407, 496–499 CrossRef CAS PubMed.
- P. G. Bruce, B. Scrosati and J. M. Tarascon, Nanomaterials for rechargeable lithium batteries, Angew. Chem., Int. Ed., 2008, 47, 2930–2946 CrossRef CAS PubMed.
- C. H. Jiang, E. Hosono and H. S. Zhou, Nanomaterials for lithium ion batteries, Nano Today, 2006, 1, 28–33 CrossRef.
- Z. Y. Wang, L. Zhou and X. W. Lou, Metal oxide hollow nanostructures for lithium-ion batteries, Adv. Mater., 2012, 24, 1903–1911 CrossRef CAS PubMed.
- C. Luo, R. M. Huang, R. Kevorkyants, M. Pavanello, H. X. He and C. S. Wang, Self-assembled organic nanowires for high power density lithium ion batteries, Nano Lett., 2014, 14, 1596–1602 CrossRef CAS PubMed.
- L. W. Ji, Z. Lin, M. Alcoutlabi and X. W. Zhang, Recent developments in nanostructured anode materials for rechargeable lithium-ion batteries, Energy Environ. Sci., 2011, 4, 2682–2699 CAS.
- C. Liu, F. Li, L. P. Ma and H. M. Cheng, Advanced materials for energy storage, Adv. Mater., 2010, 22, E28–E62 CrossRef CAS PubMed.
- B. Scrosati, J. Hassoun and Y. K. Sun, Lithium-ion batteries. A look into the future, Energy Environ. Sci., 2011, 4, 3287–3295 CAS.
- K. Jost, C. R. Perez, J. K. McDonough, V. Presser, M. Heon, G. Dion and Y. Gogotsi, Carbon coated textiles for flexible energy storage, Energy Environ. Sci., 2011, 4, 5060–5067 CAS.
- G. Milczarek and O. Inganas, Renewable cathode materials from biopolymer/conjugated polymer interpenetrating networks, Science, 2012, 335, 1468–1471 CrossRef CAS PubMed.
- I. Kovalenko, B. Zdyrko, A. Magasinski, B. Hertzberg, Z. Milicev, R. Burtovyy, I. Luzinov and G. Yushin, A major constituent of brown algae for use in high-capacity Li-ion batteries, Science, 2011, 333, 75–79 CrossRef PubMed.
- E. N. Lee, H. H. Sunwoo, K. Menninen and J. S. Sim, In vitro studies of chicken egg yolk antibody (IgY) against Salmonella enteritidis and Salmonella typhimurium, Poult. Sci., 2002, 81, 632–641 CrossRef CAS PubMed.
- L. L. Tian, X. Y. Wei, Q. C. Zhuang, C. H. Jiang, C. Wu, G. Y. Ma, X. Zhao, Z. M. Zong and S. G. Sun, Bottom-up synthesis of nitrogen-doped graphene sheets for ultrafast lithium storage, Nanoscale, 2014, 6, 6075–6083 RSC.
- H. B. Wang, C. J. Zhang, Z. H. Liu, L. Wang, P. Han, H. Xu, K. Zhang, S. Dong, J. Yao and G. Cui, Nitrogen-doped graphene nanosheets with excellent lithium storage properties, J. Mater. Chem., 2011, 21, 5430–5434 RSC.
- C. He, S. Wu, N. Zhao, C. Shi, E. Liu and J. Li, Carbon-encapsulated Fe3O4 nanoparticles as a high-rate lithium ion battery anode material, ACS Nano, 2013, 7, 4459–4469 CrossRef CAS PubMed.
- X. Liu and Y. M. Zhao, Nanotube Li2MoO4: a novel and high-capacity material as a lithium-ion battery anode, Nanoscale, 2014, 6, 13660–13667 RSC.
- N. Sharma, G. V. Subba Rao and B. V. R. Chowdari, Electrochemical properties of carbon-coated CaWO4 versus Li, Electrochim. Acta, 2005, 50, 5305–5312 CrossRef CAS.
- N. Sharma, K. M. Shaju, G. V. Subba Rao and B. V. R. Chowdari, Mixed oxides Ca2Fe2O5 and Ca2Co2O5 as anode materials for Li-ion batteries, Electrochim. Acta, 2004, 49, 1035–1043 CrossRef CAS.
- K. M. Shaju, G. V. Subba Rao and B. V. R. Chowdari, Li-ion kinetics and polarization effect on the electrochemical performance of Li(Ni1/2Mn1/2)O2, Electrochim. Acta, 2004, 49, 1565–1576 CrossRef CAS.
- K. M. Shaju, G. V. Subba Rao and B. V. R. Chowdari, Electrochemical kinetic studies of Li-ion in O2-structured Li2/3(Ni1/3Mn2/3)O2 and Li(2/3)+x(Ni1/3Mn2/3)O2 by EIS and GITT, J. Electrochem. Soc., 2003, 150, Al–A13 CrossRef.
|
This journal is © The Royal Society of Chemistry 2016 |