DOI:
10.1039/C6RA15965F
(Paper)
RSC Adv., 2016,
6, 87680-87689
Synthesis and chemosensory properties of two-arm truxene-functionalized conjugated polyfluorene containing terpyridine moiety†
Received
20th June 2016
, Accepted 23rd August 2016
First published on 26th August 2016
Abstract
We report the responsive fluorescence chemosensory phenomena of a truxene-functionalized conjugated polymer (P1) with pendant terminal terpyridine (tpy) groups as receptors for metal ions synthesized via a Suzuki polymerization reaction. We examined the structural effects of the tpy units on the sensory characteristics of the fluorescent chemosensors. The weight-average molecular weight (Mw) of P1 was 1.08 × 104 g mol−1, with its corresponding polydispersity index being 2.25. P1 exhibited high thermal stability at a thermal decomposition temperature of 334.6 °C with a 5% weight loss. Photoluminescence titrations demonstrated that P1 exhibited a high sensing ability to Fe3+ ions with a Stern–Volmer constant of 1.14 × 106 M−1. The limit of detection for Fe3+ was estimated to be 2.13 × 10−7 M. The first stepwise association constant (Ka1) for the P1/Fe3+ complex was 4.48 × 105 M−1. The fluorescence of the P1 solution that was quenched by Fe3+ ions was recovered upon the addition of trace CN− anions because of the considerably high stability constant of the CN−–Fe3+ complex. Our results suggest that the synthesized polymer is a promising material for chemosensory applications.
Introduction
In recent decades, the development of conjugated polymers has attracted an increasing amount of attention because of their use as highly sensitive fluorescent chemosensors for sensing various analytes, measuring pH and detecting metal ions and biological species in environmental applications by applying color or fluorescence intensities.1–8 Fluorescent chemosensors generally contain three components: (1) a receptor, which is a sorting or recognition site for selecting analytes reliably; (2) a fluorophore, the luminescent properties of which should be changed completely after being combined with the target substance and (3) an appropriate linker, which couples the two parts.9 Fluorescent chemosensors offer several crucial advantages over low molecular weight compounds. For instance, the chemical signals that are converted into electronic or optical signals when these conjugated polymers bind with an analyte can be transformed and enhanced effectively.10 In addition, the fluorophore of a conjugated polymer used as a fluorescent chemosensor is always bonded to the conjugated backbone, along which the excitons can diffuse easily, resulting in the fluorescence intensity and bathochromic or hypsochromic shifts of fluorescence wavelengths of these polymers changing more markedly.11–15 To date, a series of conjugated polymers have been synthesized to detect cations, anions, and pH by incorporating specific receptors in the polymers such as alkyl ethers,16 crown ethers,17 quinolones,18 bipyridines,19,20 and terpyridines (tpys).21,22
The 5H-diindeno[1,2-a:1′,2′-c]fluorine (truxene) unit, a C3h symmetric polycyclic polyarene with a rigid planar π-conjugated configuration, has attracted considerable interest and has been developed over the past decade as an optoelectronic functional template in the fields of organic light-emitting diodes, organic photovoltaics, transistors, and chemosensors.23 The truxene core is particularly suitable as a scaffold because its extended π-delocalized system can cause a substantial shift in the absorption spectrum toward the visible region. Because of the dπ–pπ* back-bonding of the metal ions to pyridines and a chelation effect useful for multinuclear supramolecular construction, 2,2′;6′,2′′-tpy units have a high binding affinity toward transition-metal ions.24,25 The supramolecular complexes formed between the tpy ligands and metal ions have been thoroughly investigated because of their relevant optical, electronic and magnetic properties.26–28 Several studies have investigated tpy units as recognition sites and it has been widely demonstrated that the choice of ligand has a marked influence on the detection of metal ions in biological and environmental systems.29,30 Combining these fluorophores consisting of truxene and tpy units affords a new platform to develop metallodendrimers and star-shaped macromolecules. However, thus far, only a few studies have been reported on these molecules, whose structures contain truxene and tpy units.31–33 Pei et al.31 prepared a series of linear and star-shaped rigid conjugated tpy ligands containing truxene or fluorene as the core with various linkages through coupling reactions. Moreover, their findings on the involved photophysical properties indicate that these compounds emit strong blue fluorescence in solutions, thereby providing potential for materials such as the hosts for constructing novel functionalized metallic materials in examining energy and electron-transfer processes.
In this study, we used a Suzuki coupling polymerization reaction to synthesize a novel rigid linear truxene-functionalized conjugated copolymer with pendant tpy groups (P1). We examined the structural effect of the tpy groups on the sensory characteristics of fluorescent chemosensors. Although numerous studies have investigated conjugated polymers comprising tpy units as recognition sites,24,25 research on linear truxene-based conjugated polymers with tpy recognition units is lacking. In contrast to previous studies, our contributions differ in that P1 benefits from the strong conjugation and luminescence properties of the asymmetric two-arm-substituted truxene monomer (M1) to yield enhanced sensing ability when mediated with metal ions. In this study, we found that P1 demonstrated a highly selective response and rapid, sensitive recognition of Fe3+ with a marked fluorescence change from bright purple to dark in a THF–H2O mixture. The Stern–Volmer constant (Ksv) and limit of detection (LOD) were 1.14 × 106 M−1 and 2.13 × 10−7 M, respectively. In addition, the resulting P1–Fe3+ complex exhibited a markedly selective fluorescence restoration with cyanide ions (CN−), making this polymer a promising material for high-potential chemosensory applications.
Experimental
Materials
The synthetic routes used to prepare the truxene-based monomer and the conjugated polymer are shown in Schemes 1 and 2. The terpyridine-functionalized compound 4′-(4-bromophenyl)-2,2′:6′,2′′-terpyridine (1) and the truxene-functionalized compounds, 5H-diindeno[1,2-a:1′,2′-c]fluorene (3) and 5,5,10,10,15,15-hexapropyl-10,15-dihydro-5H-diindeno[1,2-a:1′,2′-c]fluorene (4), were synthesized following the processes reported previously.34–37 Tetrahydrofuran (THF) and toluene were purified and distilled from sodium prior to use. N,N-Dimethylformamide (DMF) was dried with the appropriate drying agents, calcium hydride or sodium, then distilled under reduced pressure and stored over 4 Å molecular sieves before use. 1-Bromopropane (Aldrich, 98.5%), 3-phenylpropionic acid (Acros, 99.0%), bromine (Acros, 99.8%), bis(pinacolato)diboron (B2Pin2) (Acros, 95.0%), Pd(dppf)2Cl2 (Alfa, 99.0%) and other reagents were purchased from commercial Chemical Co. and used without further purification.
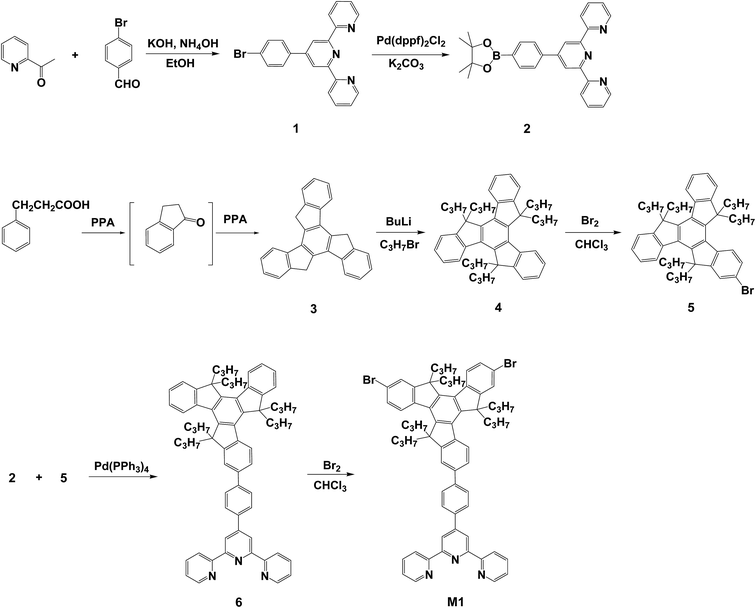 |
| Scheme 1 Synthetic routes of terpyridine-substituted monomer (M1). | |
 |
| Scheme 2 Synthetic route used to prepare the conjugated polymer (P1). | |
Measurements
All new compounds were identified by NMR and elemental analysis (EA). NMR spectra were obtained on a Bruker AMX-500 (1H NMR: 500 MHz, 13C NMR: 125 MHz) spectrometer with CDCl3 as a solvent and the chemical shifts (δ) were reported in ppm using tetramethylsilane (TMS) as an internal standard. Elemental analysis was performed on a Heraeus CHN–O rapid elemental analyzer. Fast atom bombardment mass spectra (FABMS) were obtained on a JEOL JMS-700 mass spectrometer. The weight-average molecular weight (Mw) and polydispersity index (PDI) of polymer were measured with a gel permeation chromatograph (GPC), model CR4A from Shimadzu, using tetrahydrofuran (THF) as an eluent with a rate of elution of 1.0 mL min−1; the instrument was calibrated with polystyrene standards (1000–136
000 g mol−1). Thermal analysis was performed using a differential scanning calorimeter (Perkin Elmer DSC 7) at a scanning rate of 20 K min−1 under a nitrogen atmosphere. Thermogravimetric analysis (TGA) was performed under a nitrogen atmosphere at a heating rate of 20 K min−1 using a Perkin Elmer TGA-7 thermal analyzer. UV-vis absorption spectra were obtained with a Jasco V-670 spectrophotometer. The fluorescence properties were detected with an OBB Quattro II fluorescence spectrophotometer. The fluorescence quantum yield (ΦPL) of P1 in a solution was estimated at room temperature with poly(9,9-dihexylfluorene) (PF) used as the standard (ΦPL = 1.0). Cyclic voltammograms (CV) were recorded with a voltammetric analyzer (model CV-50W from BAS) at room temperature under a nitrogen atmosphere with a scanning rate of 100 mV s−1. The measuring cell comprised a platinum wire as the working electrode, an Ag/AgNO3 electrode as the reference electrode, and a platinum wire electrode as the auxiliary electrode. The electrodes were immersed in acetonitrile containing 0.1 M (n-Bu)4NClO4 as the electrolyte. All the computational calculations were performed at the B3LYP/6-31G* level using density functional theory (DFT) with the Gaussian 09 program.38
Synthesis of intermediates and monomer
Synthesis of 4′-(4-bromophenyl)-2,2′:6′,2′′-terpyridine (1). Yield: 40.5%. Tm = 162.0 °C. 1H NMR, (CDCl3, 500 MHz): δH (ppm) = 7.49 (t, 2H), 7.80 (d, 2H), 7.92 (d, 2H), 8.01 (t, 2H), 8.75 (d, 4H), 8.81 (s, 2H). 13C NMR (CDCl3, 125 MHz, δ in ppm): 118.7, 121.5, 123.5, 124.0, 129.0, 132.2, 137.1, 149.1, 156.0. FABMS (m/z) 388 M+.
Synthesis of 4′-(4-(4,4,5,5-tetramethyl-1,3,2-dioxaborolan-2-yl)phenyl)-2,2′:6′,2′′-terpyridine (2). A mixture of 1 (0.39 g, 1.0 mmol), bis(pinacolato)diboron (B2Pin2) (0.30 g, 1.2 mmol), K2CO3 (0.69 g, 5.0 mmol), Pd(dppf)2Cl2 (43 mg, 0.070 mmol) and DMF (30 mL) was carefully degassed. The mixture was stirred for 24 h at 90 °C under a N2 atmosphere. Water (400 mL) was added and the crude product was filtered and washed with water. After drying, the crude product was dissolved in chloroform, washed with a saturated solution of EDTA and purified via recrystallization from ethanol to afford 2 as a white solid (0.24 g, 55.2%). 1H NMR (CDCl3, 500 MHz): δH (ppm) = 1.36 (s, 12H), 7.34 (t, 2H, J = 4.0 Hz), 7.83–7.92 (m, 6H), 8.65 (d, 2H, J = 8.0 Hz), 8.70–8.74 (m, 4H). 13C NMR (CDCl3, 125 MHz): δC (ppm): 21.5, 83.3, 118.7, 121.6, 123.5, 124.0, 129.1, 132.2, 137.2, 149.1, 156.1. FABMS (m/z) 435 M+.
Synthesis of 2-bromo-5,5,10,10,15,15-hexapropyl-10,15-dihydro-5H-diindeno [1,2-a:1′,2′-c]fluorene (5). To a stirred solution of 4 (0.59 g, 1.0 mmol) in chloroform (20 mL), a solution of bromine (0.08 g, 0.5 mmol) in 10 mL of chloroform was added dropwise at room temperature. After 12 h, the mixture was washed with a saturated sodium thiosulfate solution and brine. After removal of the solvent, the crude product was purified via recrystallization from ethanol to afford 5 (30.2%). 1H NMR (CDCl3, 500 MHz): δH (ppm) = 0.51 (m, 30H), 2.00–2.11 (m, 6H), 2.73–2.94 (m, 6H), 7.36–7.42 (m, 5H), 7.44–7.51 (m, 3H), 8.32–8.40 (m, 3H). 13C NMR (CDCl3, 125 MHz, δ in ppm): 14.6, 17.3, 39.3, 56.2, 121.2, 124.7, 125.7, 126.0, 126.4, 129.5, 130.1, 137.6, 138.4, 138.8, 140.3, 145.1, 154.0, 156.4. FABMS (m/z) 672 M+.
Synthesis of 4′-(4-(5,5,10,10,15,15-hexapropyl-10,15-dihydro-5H-diindeno[1,2-a:1′,2′-c]fluoren-2-yl)phenyl)-2,2′:6′,2′′-terpyridine (6). A mixture of 2 (0.87 g, 2.0 mmol), 5 (1.35 g, 2.0 mmol), tetrakis(triphenyl phosphine)palladium (Pd(PPh3)4) (69 mg, 0.06 mmol), K2CO3 (2 M, 6 mL) and toluene (20 mL) was carefully degassed. The mixture was stirred for 12 h at 90 °C under a N2 atmosphere. After removal of the solvent, the crude product was purified via recrystallization from ethanol/CH2Cl2 (1
:
5, v/v) to give 6 as a white solid (1.14 g, 63.2%). 1H NMR (CDCl3, 500 MHz): δH (ppm) = 0.50 (m, 30H), 1.92–2.23 (m, 6H), 2.65–3.00 (m, 6H), 7.16–7.61 (m, 11H), 7.67–7.91 (m, 6H), 8.03–8.22 (m, 2H), 8.59 (s, 2H), 8.61–8.76 (d, 4H). 13C NMR (CDCl3, 125 MHz, δ in ppm): 14.7, 17.7, 38.3, 46.4, 118.1, 120.9, 124.2, 125.9, 126.8, 127.9, 128.4, 130.5, 134.9, 135.9, 137.0, 139.9, 140.8, 141.1, 147.8, 148.3, 149.2, 152.1, 155.4, 156.1. FABMS (m/z) 901 M+.
Synthesis of 4′-(4-(7,12-dibromo-5,5,10,10,15,15-hexapropyl-10,15-dihydro-5H-diindeno[1,2-a:1′,2′-c]fluoren-2-yl)phenyl)-2,2′:6′,2′′-terpyridine (M1). To a stirred solution of 6 (0.45 g, 0.5 mmol) in chloroform (15 mL), a solution of bromine (0.32 g, 2.0 mmol) in 10 mL of chloroform was added dropwise at room temperature. After 12 h, the mixture was washed with a saturated sodium thiosulfate solution and brine. Removal of the solvent gave a yellow solid. Recrystallization from ethanol/CH2Cl2 (1
:
5, v/v) afforded a white solid (0.18 g, 34.0%). Tm = 168.6 °C. 1H NMR (CDCl3, 500 MHz): δH (ppm) = 0.43–0.56 (m, 30H), 1.92–2.12 (m, 6H), 2.65–2.89 (m, 6H), 7.26–7.61 (m, 11H), 7.84–8.19 (m, 6H), 8.60 (s, 2H), 8.62–8.76 (d, 4H). Anal. calcd (%) for C66H65Br2N3: C, 74.78; H, 6.18; N, 3.96. Found: C, 74.92; H, 6.22; N, 4.01. 13C NMR (CDCl3, 125 MHz, δ in ppm): 14.7, 17.6, 38.3, 46.4, 118.1, 120.9, 124.1, 125.9, 126.8, 127.9, 130.5, 134.9, 135.9, 137.1, 139.9, 140.7, 141.1, 147.8, 148.3, 149.2, 152.1, 155.4, 156.1. Anal. calcd (%) for C66H65Br2N3: C, 74.78; H, 6.18; N, 3.96. Found: C, 74.84; H, 6.09; N, 4.01. FABMS (m/z) 1060 M+.
Synthesis of the conjugated polymer
Synthesis of poly[2,7-(5,5,10,10,15,15-hexapropyl-10,15-dihydro-5H-diindeno[1,2-a:1′,2′-c]fluorene)-alt-4′-phenyl-2,2′:6′,2′′-terpyridine] (P1). The conjugated polymer P1 was prepared using a palladium-catalyzed Suzuki coupling reaction of the monomers A/M1 in toluene at 90 °C using Pd(PPh3)4 as the catalyst. A mixture of A (0.29 g, 0.5 mmol), M1 (0.53 g, 0.5 mmol), Pd(PPh3)4 (49 mg, 0.04 mmol), K2CO3 (2 M, 2 mL) and toluene (7 mL) was carefully degassed. The mixture was stirred for 72 h at 90 °C under a N2 atmosphere. Then, bromobenzene (0.08 g, 0.5 mmol) and phenylboronic acid (0.06 g, 0.5 mmol) were added for the end capping step upon refluxing for 6 h. The mixture was cooled to room temperature. After removal of the solvent, the residue was added to CH3OH (500 mL) to precipitate out the polymer. The resulting precipitate was placed in a Soxhlet apparatus and extracted with CH3OH for 72 h and then dried in vacuum to give 0.23 g (44.3%). Mw = 1.08 × 104 g mol−1, PDI = 2.25. 1H NMR (CDCl3, 500 MHz): δH (ppm) = 0.35–0.98 (br, CH3 and CH2), 1.76–2.44 (br, CH2), 2.69–3.12 (br, CH2), 7.27–9.10 (br, Ar-H). Anal. calcd (%) from feed (C25H32)(C66H65N3): C, 76.31; H, 6.78; N, 2.94. Found: C, 75.84; H, 6.54; N, 2.82.
Fluorescent titration with metal ions
Fluorescent titration experiments were performed in THF solutions. Stock solutions (1.0 × 10−3 M) of the chloride or nitrate salts of Ag+, Al3+, Ba2+, Ca2+, Fe2+, Fe3+, K+, Mg2+, Mn2+, Ni2+, Pb2+, Li+, and Zn2+ in deionized water were prepared. Titrations were performed by adding the stock solutions to a test tube containing the polymer solution. All optical measurements were conducted immediately after the test solutions were prepared and thoroughly mixed. The final concentration of the polymer was 1.0 × 10−6 M. The percentage of water in THF was approximately 1.0%. The Stern–Volmer constant (Ksv) was estimated according to following equation:where Io and I are the intensities of the PL spectra without and with a quencher, respectively, Ksv is the Stern–Volmer constant (quenching coefficient) and [Q] is the concentration of the quencher ions. Stabilization of the P1–Fe3+ complex (1.0 × 10−6 M in THF) was studied in the presence of some anions, including F−, Cl−, Br−, NO3−, SO42−, S2−, and CN− (2.0 × 10−5 M). The limit of detection (LOD) of P1 was calculated on the basis of fluorescence titrations. To obtain the slope, the fluorescence emission at 393 nm was plotted against the concentration of Fe3+. The LOD was calculated using the following equation: |
Limit of detection (LOD) = 3σ/S
| (2) |
where σ is the standard deviation of the blank signal and S is the slope between the fluorescence intensity versus [Fe3+].
Results and discussion
Synthesis of the intermediates and monomer
Scheme 1 outlines the synthetic route used to prepare the tpy-functionalized monomer M1. The bromo-functionalized compound 5, 2-bromo-5,5,10,10,15,15-hexapropyl-10,15-dihydro-5H-diindeno[1,2-a:1′,2′-c]fluorene, was prepared through the bromination reaction of compound 4 (5,5,10,10,15,15-hexapropyl-10,15-dihydro-5H-diindeno[1,2-a:1′,2′-c]fluorene) at room temperature. The crude product was purified via recrystallization from ethanol to afford 5, thus creating compound 5 in a yield of 30.2%. We prepared 4′-(4-(5,5,10,10,15,15-hexapropyl-10,15-dihydro-5H-diindeno[1,2-a:1′,2′-c]fluoren-2-yl)phenyl)-2,2′:6′,2′′-terpyridine (6) in 63.2% yield using a Suzuki coupling reaction between 4′-(4-(4,4,5,5-tetramethyl-1,3,2-dioxaborolan-2-yl)phenyl)-2,2′:6′,2′′-terpyridine (2) and compound 5 in the presence of potassium carbonate at 90 °C. The reaction was performed using Pd(PPh3)4 as the catalyst. The target monomer, 4′-(4-(7,12-dibromo-5,5,10,10,15,15-hexapropyl-10,15-dihydro-5H-diindeno[1,2-a:1′,2′-c]fluoren-2-yl)phenyl)-2,2′:6′,2′′-terpyridine (M1), was prepared using a procedure similar to that used for compound 5, except that compound 6 was used instead of compound 4, resulting in M1 in 34.0% yield. The chemical structures and constitutional composition of the synthesized monomer were confirmed using proton nuclear magnetic resonance (1H NMR) spectroscopy. Fig. S1a† shows the 1H NMR spectrum of M1 and its corresponding structural assignments. The characteristic chemical shifts of δ = 8.62–8.76 (Ha) and δ = 8.60 ppm (Hb) were attributed to the terpyridyl doublet and singlet proton signals in M1, respectively, and those at δ = 2.65–2.89, 1.92–2.12 and 0.43–0.56 ppm were ascribed to the aliphatic methylene (Hc and Hd) and methyl (He) groups of the truxene unit, respectively. 13C NMR measurements (Fig. S2†) were used to confirm the structure of intermediates 1 and 5. Fig. S3 and S4† show the FABMS (fast atom bombardment mass spectroscopy) of the two representative tpy-functionalized intermediate 1 and monomer M1, respectively. In addition, the chemical structures of the synthesized monomer were also confirmed using elemental analysis.
Synthesis and thermal properties of the conjugated polymer
P1 was successfully synthesized from M1 and A via a palladium-mediated Suzuki coupling reaction (Scheme 2). Fig. S1b† shows the 1H NMR spectrum of P1 in CDCl3. The 1H NMR spectrum of P1 exhibited peaks at approximately 8.97–9.10 ppm, which were assigned to shifts of the ortho and meta positions labeled Ha of the pyridine unit, whereas the protons (Hb) were the two aromatic protons of the 2,2′:6′,2′′-terpyridyl segment at 8.82–8.96 ppm. The other aromatic protons appeared at 7.27–8.54 ppm. Finally, the 1H NMR spectrum of P1 had the alkyl group peaks at approximately 2.69–3.12, 1.94–2.38, 0.78–0.95 and 0.40–0.66 ppm assigned to the aliphatic protons labeled Hc, Hd+e, Hf, and Hg+h, respectively. The weight-average molecular weight (Mw) of P1 was 1.08 × 104 g mol−1 with a corresponding polydispersity index of 2.25 (Table 1). The molar percentage of the tpy-functionalized monomer (M1) in P1 was estimated at approximately 44.5% according to the integrated peak areas of the aliphatic group of truxene (Hc and Hd) and the methylene protons of fluorene (He). The estimated molar percentage of M1 in the polymer was lower than that of the feed (50.0%), indicating that the lower percentage was attributed to the steric effect and electron-deficient character of the tpy monomer, resulting in reduced monomer reactivity. P1 exhibited high thermal stability with a thermal decomposition temperature (Td) of 334.6 °C at 5% weight loss (Fig. 1). The residual weight of P1 at 800 °C was approximately 61.2%, indicating its high thermal stability.
Table 1 Molecular weight and thermal properties of the conjugated polymer
Polymer |
Yield (%) |
Mwa (×104) |
PDIa |
Tgb (°C) |
Tdc (°C) |
The Mw and PDI of the conjugated polymer were determined by gel permeation chromatography using polystyrene standards in THF. The glass transition temperatures were determined using DSC under an N2 atmosphere at a heating rate of 20 °C min−1. The temperatures at 5% weight loss. |
P1 |
44.3 |
1.08 |
2.25 |
113.5 |
334.6 |
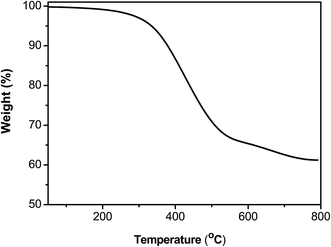 |
| Fig. 1 TGA curve obtained for P1 at a heating rate of 20 °C min−1 under a nitrogen atmosphere. | |
Optical and electrochemical properties
Fig. 2 shows the absorption and PL spectra of P1 in THF (1.0 × 10−6 M) and as thin films spin-coated from the THF solutions (10 mg mL−1) with the corresponding data summarized in Table 2. In THF, P1 exhibited main absorption peaks at 284 and 309 nm, which resulted from a π–π* transition in the truxene units, consistent with a finding reported in the literature.39 Another weak transition shoulder in P1 was observed at approximately 363 nm, which was attributed to the intramolecular charge transfer (ICT) between the truxene donor groups and the tpy acceptor, leading to the observed ICT interaction and bathochromic shift.40,41 In the thin film state, the absorption maximum of P1 was red-shifted (ca. 53 nm) relative to the solution state, resulting from aggregation caused by intra-chain or interchain interactions. The fluorescence maximum of P1 in THF was 393 nm. In the film state, the emission maximum was situated at 433 nm, implying that excimers or exciplexes form in the thin film state. Table 2 lists the Stokes shift of P1 at 96 nm and the large shift may result from structural differences between the ground and excited states as well as migrated excitons in the segments of the chain, where the ring rotates more flexibly.
 |
| Fig. 2 Normalized UV-vis absorption and PL spectra of P1 in THF solution (solid line) and as a thin film (dotted line). | |
Table 2 Optical electrochemical properties of the polymer
Polymer |
UV-vis λmaxa sol'n (nm) |
UV-vis λmaxa film (nm) |
PL λmaxb sol'n (nm) |
PL λmaxb film (nm) |
Stokes shiftc |
ΦPLd |
Measured in THF solution (1.0 × 10−6 M); sh = shoulder. The excitation wavelength was 300 nm in THF for the polymer. Stokes shift = PL(film)/nm − UV(film)/nm. The values obtained for the quantum yield were measured using PF as a standard (1.0 × 10−7 M, assuming a quantum yield of unity). |
P1 |
284, 309, 363sh |
337 |
393 |
433 |
96 |
1.82 |
Fig. S5† depicts the estimated cyclic voltammogram of P1 in CH2Cl2 at a scanning rate of 100 mV s−1 and Table 3 presents a summary of the electrochemical data. The highest occupied molecular orbital (HOMO) and lowest unoccupied molecular orbital (LUMO) energy levels were evaluated according to the equations, EHOMO = −(Eox + 4.8) eV and ELUMO = EHOMO + Eoptg, where Eox is the onset oxidation potential regarding the standard ferrocene/ferrocenium (FOC) redox system. The optical band gap (Eoptg) was determined based on the onset absorption wavelength. The onset oxidation peaks of P1 were observed at approximately 0.27 and 0.55 V. We attributed these two oxidation potentials of P1 to the charge delocalization of the extended π-systems in the truxene and fluorene groups, respectively. The estimated molecular orbital levels of P1 are −5.07/−5.35 (HOMO/HOMO−1) and −2.06 eV (LUMO). The optical bandgap (Eoptg) value for P1, determined according to the onset of absorption in the solution state, was 3.01 eV (412 nm).
Table 3 Optical and electrochemical properties of the polymer
Polymer |
UV-vis λonseta (nm) |
Eox (onset)b (V) |
EHOMOc (eV) |
ELUMOd (eV) |
Eg (opt)e (eV) |
Eg (the)f (eV) |
The onset wavelength of the polymer in the absorption spectra. The cyclic voltammograms obtained for the polymer in CH2Cl2 (1.0 × 10−4 M) at a scanning rate of 100 mV s−1 (vs. FOC+/FOC). EHOMO = −(Eox + 4.8) eV. ELUMO = EHOMO + Eg (opt). The optical band gap was determined from the onset of absorption in CH2Cl2 (Eg (opt) = 1240/λonset). The theoretical band gap was calculated using density functional theory calculations. The molecular orbital was HOMO−1. |
P1 |
412 |
0.27, 0.55 |
−5.07, −5.35g |
−2.06 |
3.01 |
3.97 |
Theoretical calculations
To obtain further insight into the electronic effect of the polymer on the molecular geometry and electronic structure, density functional theory (DFT) calculations were performed using the B3LYP/6-31G* level of theory to predict the minimum-energy conformations and the frontier molecular orbitals of the model compounds of P1. As presented in Fig. S6,† the optimized structures of the model compound (i.e., repeating unit n = 1 in Scheme 2) revealed that the HOMO for P1 is nearly delocalized on the entire backbone of the model compound, whereas the LUMO is mainly located on the tpy unit, indicating the efficient charge separation of the model compound. According to the DFT calculations, the HOMO and LUMO values of P1 were −5.49 and −1.52 eV, respectively. This also means that the theoretical bandgap (Etheg) value for P1 was 3.97 eV. This result was different from the data of the UV-vis absorption and CV experiments (i.e., Eoptg = 3.01 eV), for which we attributed the larger bandgap to the small repeating unit number (i.e., n = 1) of the model compound. In addition, we found that the model compound exhibited a high coplanar arrangement, in which the largest dihedral angle was only 36.8° between truxene and tpy units, indicating that an efficient conjugation effect between truxene and tpy units was induced, leading to an effective ICT.
Ion sensing properties
The tpy groups are generally employed as ligands to combine with metal cations to generate complexes. Fig. 3a shows the effect of complexation with various metal ions (Ag+, Al3+, Ba2+, Ca2+, Fe2+, Fe3+, K+, Mg2+, Mn2+, Ni2+, Pb2+, Li+, and Zn2+) on the fluorescence spectra of P1 in a THF–H2O solution. The fluorescence intensity of the P1 solution decreased significantly upon the addition of Fe2+, Fe3+, Ag+, Zn2+, or Ni2+ ions (Fig. 3a), indicating that the tpy chelating moiety in the side chain effectively transferred energy from the conjugated polymer backbone to these cations, thus leading to the fluorescence quenching of P1. In particular, P1 was quenched completely by Fe3+ ions with an ion concentration of 5.0 × 10−5 M, indicating their high selectivity toward this cation. The quenching mechanism of the polymer can be attributed to the photoinduced energy transfer occurring in a collision (dynamic quenching) between the excited fluorophore and the metal ions. We also attributed the quenching behavior to strong cation binding between the Fe3+ ions and the tpy chelating groups; the binding results from the effective energy transfer migration between the receptors and polymer chains. Thus, P1 can be used in polymer chemosensors for detecting metal ions.
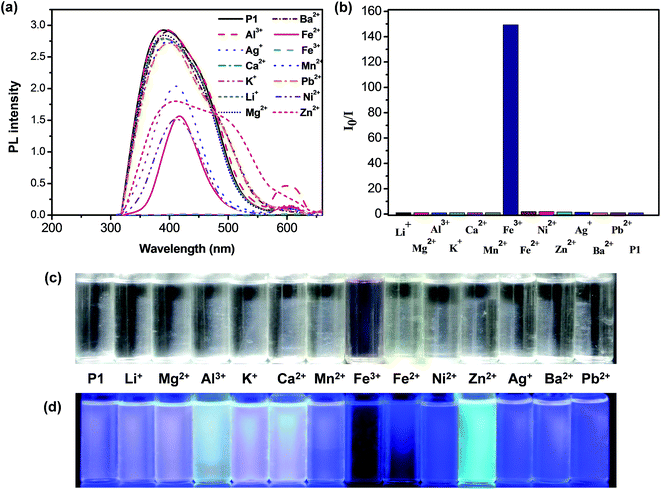 |
| Fig. 3 (a) Photoluminescence spectra, (b) PL response profiles and images of P1 in the presence of various cations under (c) sunlight and (d) UV excitation (λex = 300 nm). The concentration of conjugated polymer was 1.0 × 10−6 M in THF and the concentration of metal ions was 5.0 × 10−5 M. | |
Fig. 3b shows the photoluminescence response profiles (i.e., Io/I) of P1 in the presence of various metal ions with an ion concentration of 5.0 × 10−5 M. The emission colors of the fluorescence responses to various metal ions were visible (Fig. 3d). We found that P1 showed a high sensitivity toward Fe3+ with a marked fluorescence change from bright purple to dark in the THF–H2O mixture. These results indicated that the shorter diameter (1.28 Å) and higher charge (1.83) of Fe3+ may lead to a higher electron-accepting capability and consequently, more stable complexes. Moreover, these two factors (i.e., diameter and charge) may play a vital role in determining the coordination strength of Fe3+ ions accompanying the tpy units.42 The five electrons (Fe3+: d5 electron configuration) may be present as two orbitals occupied by pairs of electrons and one having single occupancy, leading to the inner-orbital complex, which was more stable than the other host/metal complexes.43 The ΦPL value of P1 in the presence of Fe3+ ions decreased by approximately 99.8% (i.e., from 1.82 to 0.6 × 10−2). Moreover, adding Zn2+ ions induced a broad red-shifted emission in the range of 500–600 nm for P1, which was ascribed to the enhancement of the ICT processes. Adding metal ions (Ag+, Al3+, Ca2+, Fe2+, Fe3+, K+, Mg2+, Ni2+, and Li+) into P1 led to a new emission peak at approximately 601 nm, which was attributed to the phosphorescence derived from the P1–metal ion complexes.44–46 These results also indicated that these shifts were ascribed to the similar energy level of metal ions and truxene triplets and to the spin–orbit coupling induced by the metal ions,45 or to the formation of the polymeric self-assembled structure by the metal ion coordination, which hinders the intramolecular rotations and motions of the core chromophore and favors radiative deactivation of the luminescent excited state.46
To quantitatively evaluate the fluorescence quenching attained by adding Fe3+, the fluorescence spectra and the response of P1 to titration with Fe3+ were studied in THF. The emission intensities at 393 nm (i.e., I393 nm) gradually decreased with increasing Fe3+ concentration (Fig. 4a). The slope of the Stern–Volmer plot turned noticeably upwards (i.e., static quenching), resulting from strong tpy chelation to the transition metal ions, when the Fe3+ concentrations were greater than 2.0 × 10−5 M. Ksv for P1 in the presence of low Fe3+ concentrations (below approximately 1.5 × 10−5 M) was 1.14 × 106 M−1. The estimated detection limit for P1 toward Fe3+ was as low as 2.13 × 10−7 M, enabling P1 to be a selective and sensitive Fe3+ probe. As shown in Fig. 3b, a new absorption peak at 580 nm appeared in the P1 solution when it was titrated with Fe3+ ions. The color of the P1 solution under sunlight changed from colorless to purple (Fig. 3c), suggesting that P1 can be employed as a useful probe for detecting Fe3+ ions. The first stepwise association constant (Ka1) for the P1–Fe3+ complex was calculated following the photoluminescence titration experiment.47,48 The Ka1 of this system for P1 was 4.48 × 105 M−1 at 393 nm (Fig. 4a).
 |
| Fig. 4 (a) Photoluminescence and (b) absorption spectra of P1 in the presence of various concentrations of Fe3+ ions. The inset in (a) shows the Stern–Volmer plot of PL quenching with various concentrations of Fe3+ ions. The concentration of conjugated polymer was 1.0 × 10−6 M in THF. | |
Fig. 5 shows the specificity of P1 (1.0 × 10−6 M in THF) toward Fe3+ ions in the presence of various cations (1.0 × 10−5 M in water). We examined whether P1 can retain its sensing response to Fe3+ in the presence of competitive cations. The changes in the enhanced ratio of fluorescence intensity (i.e., Io/I) of the polymer were measured on the addition of other metal ions. No responses were found, as shown by the black bars in this figure. Adding 1.0 × 10−5 M of Fe3+ in P1 solutions then yielded 160- to 310-fold emission enhancements with and without other metal ions, respectively (Fig. 5, red bars). In other words, the presence of other metal ions seemed to have no effect on the response of P1 towards Fe3+. Therefore, the fluorescence interference titration indicated that the Fe3+-dependent fluorescence response of P1 was not affected by other transition metal ions.
 |
| Fig. 5 Fluorescent quenching rate of P1 in the presence of different cations with and without Fe3+ ions in THF solution. The black bars represent the addition of the appropriate metal ion (1.0 × 10−5 M in water) to a solution of the conjugated polymer (1.0 × 10−6 M in THF). The red bars represent the subsequent addition of Fe3+ (1.0 × 10−5 M in water) to the former solution. | |
To determine whether the P1–Fe3+ complex can be used as an anion selective probe, the response of the complex toward anions (F−, Cl−, Br−, NO3−, SO42−, S2−, and CN−) was investigated (Fig. 6a). The fluorescence response profiles (i.e., I/Io) of the P1–Fe3+ complexes in the presence of CN− anions at a concentration of 2.0 × 10−5 M are provided in Fig. 6b. These data illustrate that the fluorescence intensity of the P1–Fe3+ complex increased considerably on the addition of CN− ions, whereas complexation with other anions induced no obvious shift in the emission peak because of the poor coordination of Fe3+ with these anions. However, in the presence of S2−, the PL spectrum of the P1–Fe3+ complex exhibited a slight increase in its fluorescence intensity. The complex demonstrated a markedly selective fluorescence “turn-on” behavior exclusively with CN− ions, indicating that CN− ions can effectively coordinate with Fe3+ instead of P1. This finding was attributed to the high stability of the Fe3+–CN− complex.49,50 Hence, the fluorescence intensities of P1 can be revived by the transformation from the polymer–Fe3+ complex (quenched) to the free polymer (revived). When the concentration of added CN− was 1.8 × 10−5 M, the fluorescence intensity recovered by up to 48.6% of the original intensity, whereas adding S2− led to a recovery of up to 6.5% of the original intensity (Fig. 7). Moreover, the addition of other anions induced a recovery of approximately 0.1% of the original intensity, enabling P1 to be used as a sensitive CN− sensor.
 |
| Fig. 6 (a) Photoluminescence spectra and (b) PL response profiles of P1–Fe3+ in the presence of various anions. | |
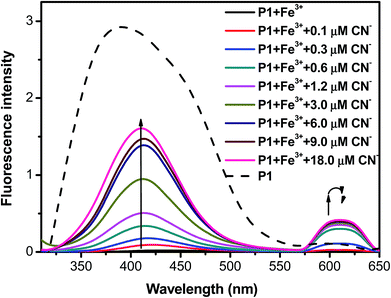 |
| Fig. 7 Photoluminescence spectra of P1–Fe3+ in the presence of various concentrations of CN− anions. | |
Conclusions
In summary, a new truxene-functionalized conjugated copolymer with tpy groups as receptors (P1) was synthesized, and its corresponding optical and sensory characteristics were evaluated. P1 exhibited high thermal stability with the thermal decomposition temperature being 334.6 °C at 5% weight loss. P1 exhibited high sensitivity toward Fe3+ ions (“turn-off”) with a Stern–Volmer constant (Ksv) of 1.14 × 106 M−1 and an LOD of 2.13 × 10−7 M. Furthermore, the polymer–Fe3+ complex demonstrated particularly selective fluorescence restoration (“turn-on”) ability with CN− ions. These results indicate that P1 can meet the selective requirements for environmental applications and is sufficiently sensitive to detect Fe3+ ions in environmental water samples, including drinking water.
Acknowledgements
Financial support for this study by the grants from the Ministry of Science and Technology, Taiwan (No. 103-2221-E-155-072 and 105-2221-E-155-079) is gratefully appreciated.
Notes and references
- P. G. Del Rosso, M. J. Romagnoli, M. F. Almassio and C. A. Barbero, Sens. Actuators, B, 2014, 203, 612–620 CrossRef CAS
. - Y. Yang, G. Zhang, H. Luo, J. Yao, Z. Liu and D. Zhang, ACS Appl. Mater. Interfaces, 2016, 8, 3635–3643 CAS
. - Y. Zhou, L. Tang, G. Zeng, C. Zhang, Y. Zhang and X. Xie, Sens. Actuators, B, 2016, 223, 280–294 CrossRef CAS
. - O. S. Wolfbeis, Chem. Soc. Rev., 2015, 44, 4743–4768 RSC
. - M. Saleem and K. H. Lee, RSC Adv., 2015, 5, 72150–72287 RSC
. - S. Cao, Z. Pei, Y. Xu, R. Zhang and Y. Pei, RSC Adv., 2015, 5, 45888–45896 RSC
. - L. Zhang, X. Lou, Y. Yu, J. Qin and Z. Li, Macromolecules, 2011, 44, 5186–5193 CrossRef CAS
. - X. Lou, Y. Zhang, S. Li, D. Ou, Z. Wan, J. Qin and Z. Li, Polym. Chem., 2012, 3, 1446–1452 RSC
. - Y. Long, H. Chen, H. Wang, Z. Peng, Y. Yang, G. Zhang, N. Li, F. Liu and J. Pei, Anal. Chim. Acta, 2012, 744, 82–91 CrossRef CAS PubMed
. - Z. Chen, Q. Wang, X. Wu, Z. Li and Y. B. Jiang, Chem. Soc. Rev., 2015, 44, 4249–4263 RSC
. - C. M. Darr, V. Korampally, B. Chen, K. Gangopadhyay and S. Gangopadhyay, Sens. Actuators, B, 2014, 202, 1088–1096 CrossRef CAS
. - A. C. Bhasikuttan and J. Mohanty, Chem. Commun., 2015, 51, 7581–7597 RSC
. - L. Qin, B. Lu, J. Xu, G. Zhang and S. Zhang, RSC Adv., 2014, 4, 28368–28376 RSC
. - C. Kaewtong, N. Niamsa, B. Pulpoka and T. Tuntulani, RSC Adv., 2014, 4, 52235–52240 RSC
. - D. Mao, X. Liu, Q. Qiao, W. Yin, M. Zhao, J. M. Cole, J. Cui and Z. Xu, Analyst, 2015, 140, 1008–1013 RSC
. - A. Balamurugan, V. Kumar and M. Jayakannan, Chem. Commun., 2014, 50, 842–845 RSC
. - G. Xiang, L. Wang, W. Cui, X. An and L. Zhou, Sens. Actuators, B, 2014, 196, 495–503 CrossRef CAS
. - H. Kim, G. R. You, G. J. Park, J. Y. Choi, I. Noh, Y. Kim, S. J. Kim, C. Kim and R. G. Harrison, Dyes Pigm., 2015, 113, 723–729 CrossRef CAS
. - F. Jin, W. Shu, X. Yu, Y. Zhang, L. Sun, Y. Liu, D. Tao, H. Zhou and Y. Tian, Dyes Pigm., 2015, 121, 379–384 CrossRef CAS
. - A. Bellusci, M. Ghedini, L. Giorgini, F. Gozzo, E. I. Szerb, A. Crispini and D. Pucci, Dalton Trans., 2009, 7381–7389 RSC
. - S. Jing, C. Zheng, S. Pu, C. Fan and G. Liu, Dyes Pigm., 2014, 107, 38–44 CrossRef CAS
. - Z. B. Zheng, S. Y. Kang, Y. Zhao, N. Zhang, X. Yi and K. Z. Wang, Sens. Actuators, B, 2015, 221, 614–624 CrossRef CAS
. - F. Goubard and F. Dumur, RSC Adv., 2015, 5, 3521–3551 RSC
. - M. Zheng, H. Tao, Z. Xie, L. Zhang, X. Jing and Z. Sun, ACS Appl. Mater. Interfaces, 2013, 5, 1078–1083 CAS
. - S. Karmakar, D. Maity, S. Mardanya and S. Baitalik, Dalton Trans., 2015, 44, 18607–18623 RSC
. - U. S. Schubert, H. Hofmeier and G. R. Newkome, Modern Terpyridine Chemistry, Wiley-VCH, Weinheim, Germany, 2006 Search PubMed
. - A. Wild, A. Winter, F. Schlütter and U. S. Schubert, Chem. Soc. Rev., 2011, 40, 1459–1511 RSC
. - E. C. Constable, Chem. Soc. Rev., 2007, 36, 246–253 RSC
. - P. Mahato, S. Saha, P. Das, H. Agarwalla and A. Das, RSC Adv., 2014, 4, 36140–36174 RSC
. - Y. Zhou and J. Yoon, Chem. Soc. Rev., 2012, 41, 52–67 RSC
. - S. C. Yuan, H. B. Chen, Y. Zhang and J. Pei, Org. Lett., 2006, 8, 5701–5704 CrossRef CAS PubMed
. - B. Ventura, A. F. Barigelletti, S. Diring and R. Ziessel, Inorg. Chem., 2010, 49, 8333–8346 CrossRef CAS PubMed
. - J. L. Wang, Y. T. Chan, C. N. Moorefield, J. Pei, D. A. Modarelli, N. C. Romano and G. R. Newkome, Macromol. Rapid Commun., 2010, 31, 850–855 CrossRef CAS PubMed
. - S. K. Lee, D. H. Hwang, B. L. Jung, N. S. Cho, J. Lee, J. D. Lee and H. K. Shim, Adv. Funct. Mater., 2005, 15, 1647–1655 CrossRef CAS
. - F. Dumur, C. R. Mayer, E. Dumas, F. Miomandre, M. Frigoli and F. Sécheresse, Org. Lett., 2008, 10, 321–324 CrossRef CAS PubMed
. - X. Y. Cao, H. Zi, W. Zhang, H. Lu and J. Pei, J. Org. Chem., 2005, 70, 3645–3653 CrossRef CAS PubMed
. - J. Pei, J. L. Wang, X. Y. Cao, X. H. Zhou and W. B. Zhang, J. Am. Chem. Soc., 2003, 125, 9944–9945 CrossRef CAS PubMed
. - M. J. Frisch, G. W. Trucks, H. B. Schlegel, G. E. Scuseria, M. A. Robb, J. R. Cheeseman, G. Scalmani, V. Barone, B. Mennucci, G. A. Petersson, H. Nakatsuji, M. Caricato, X. Li, H. P. Hratchian, A. F. Izmaylov, J. Bloino, G. Zheng, J. L. Sonnenberg, M. Hada, M. Ehara, K. Toyota, R. Fukuda, J. Hasegawa, M. Ishida, T. Nakajima, Y. Honda, O. Kitao, H. Nakai, T. Vreven, J. A. Montgomery Jr, J. E. Peralta, F. Ogliaro, M. Bearpark, J. J. Heyd, E. Brothers, K. N. Kudin, V. N. Staroverov, R. Kobayashi, J. Normand, K. Raghavachari, A. Rendell, J. C. Burant, S. S. Iyengar, J. Tomasi, M. Cossi, N. Rega, J. M. Millam, M. Klene, J. E. Knox, J. B. Cross, V. Bakken, C. Adamo, J. Jaramillo, R. Gomperts, R. E. Stratmann, O. Yazyev, A. J. Austin, R. Cammi, C. Pomelli, J. W. Ochterski, R. L. Martin, K. Morokuma, V. G. Zakrzewski, G. A. Voth, P. Salvador, J. J. Dannenberg, S. Dapprich, A. D. Daniels, O. Farkas, J. B. Foresman, J. V. Ortiz, J. Cioslowski and D. J. Fox, Gaussian 09, Revision A.1, Gaussian, Inc., Wallingford CT, 2009 Search PubMed
. - Y. Wu, X. Hao, J. Wu, J. Jin and X. Ba, Macromolecules, 2010, 43, 731–738 CrossRef CAS
. - P. Leriche, A. Frère, A. Cravino, O. Alévêque and J. Roncali, J. Org. Chem., 2007, 72, 8332–8336 CrossRef CAS PubMed
. - Y. J. Cheng, S. H. Yang and C. S. Hsu, Chem. Rev., 2009, 109, 5865–5923 CrossRef PubMed
. - J. E. House, Inorganic Chemistry, Academic press, Elsevier, Burlington, MA, 2008 Search PubMed
. - Y. Cui, Q. Chen, D. D. Zhang, J. Cao and B. H. Han, J. Polym. Sci., Part A: Polym. Chem., 2010, 48, 1551–1556 CrossRef
. - B. Du, D. Fortin and P. D. Harvey, Inorg. Chem., 2011, 50, 11493–11505 CrossRef CAS PubMed
. - B. Ventura, A. Barbieri, F. Barigelletti, S. Diring and R. Ziessel, Inorg. Chem., 2010, 49, 8333–8346 CrossRef CAS PubMed
. - A. Fermi, G. Bergamini, M. Roy, M. Gingras and P. Ceroni, J. Am. Chem. Soc., 2014, 136, 6395–6400 CrossRef CAS PubMed
. - M. Brzezinski and T. Biela, Macromolecules, 2015, 48, 2994–3004 CrossRef CAS
. - Z. Qu, H. Jin, Y. Guo, S. Li, H. Li, Y. Li, X. Wang and G. Yang, J. Phys. Chem. B, 2012, 116, 2048–2058 CrossRef PubMed
. - Q. Zeng, P. Cai, Z. Li, J. Qin and B. Z. Tang, Chem. Commun., 2008, 1094–1096 RSC
. - X. Lou, D. Ou, Q. Li and Z. Li, Chem. Commun., 2012, 48, 8462–8477 RSC
.
Footnote |
† Electronic supplementary information (ESI) available. See DOI: 10.1039/c6ra15965f |
|
This journal is © The Royal Society of Chemistry 2016 |
Click here to see how this site uses Cookies. View our privacy policy here.