DOI:
10.1039/C6RA15764E
(Review Article)
RSC Adv., 2016,
6, 94325-94351
Biodegradable polymeric nanostructures in therapeutic applications: opportunities and challenges
Received
17th June 2016
, Accepted 19th September 2016
First published on 22nd September 2016
Abstract
Biodegradable polymeric nanostructures (BPNs) have shown great promise in different therapeutic applications such as diagnosis, imaging, drug delivery, cosmetics, organ implants, and tissue engineering. BPNs exhibit superior properties over their respective bulk materials, overcoming the limitations of traditional polymers to address numerous important medical problems. Furthermore, the addition of other components can enable specific functionalities of the nanostructures to be modulated for tunable applications. This review emphasizes the state-of-the-art of biodegradable polymeric nanostructures, preparative methods, therapeutic uses, and challenging issues concerning the commercialization of emerging BPN-based therapeutics with respect to their future perspectives.
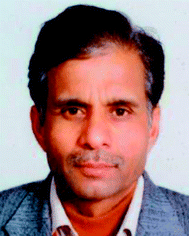 S. K. Shukla | S. K. Shukla is currently working as Faculty in Department of the Polymer Science, Bhaskaracharya College of Applied Sciences, University of Delhi, India. He was born and brought up in Gorakhpur and earned his MSc and PhD degrees from D. D. U. Gorakhpur University, India. He has published more than 100 papers and abstracts in different international and national journals and conference proceedings, along with eight contributory chapters in books of international repute. He edited a book entitled “Advanced Carbon Materials and Technology”, for John Wiley, Scrivener Publishing, LLC, 2013, ISBN: 978-1-118-68623-2. Dr Shukla is also an editorial board member for the International Journal of Nanotechnology in Diagnosis and Treatment, the Austin Journal of Biosensors & Bioelectronics, and Sensors and Actuators, and he is a member of different scientific societies. Dr Shukla has also received different prestigious national and international awards for his scientific contributions, such as the Advanced Materials Letter Award – 2012, the International Association of Advanced Materials Scientists Awards – 2011, the Indian Association of Solid State and Allied Chemists (Bronze Medal – 2011), best paper awards during the international conference on Advanced Polymeric materials – 2013, at the Central Institute of Plastics Engineering and Technology, Lucknow, the Dr N. G. Patel Award at NPTS-16, Lucknow University, Lucknow, and the Advanced Materials World Congress – 2013, Izmir, Turkey. His major research interests are advanced materials, micro-analytical techniques, nano composites, bio-conducting polymers, and opto-chemical devices. |
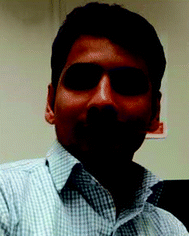 Sudheesh K. Shukla | Sudheesh K. Shukla is a Post-Doctoral Research Fellow in the Department of Biomedical Engineering at Ben-Gurion University of the Negev. Before moving to BGU, Dr Shukla was a postdoctoral research fellow at the University of Johannesburg, South Africa, with a Global Excellence and Stature (GES) postdoctoral fellowship. His present research work focuses on stimuli-encoded polymeric and 2D-based nanobioreactors for novel biosensor fabrication. He has extensive experience in polymer chemistry (polymer design, synthesis, and characterization), nanocomposite synthesis, nanobiotechnology, catalysis science, and biosensors/sensors. Dr Shukla has visiting research fellow positions at Linköping University, Sweden and the University of Johannesburg, South Africa. He worked as a managing editor of the Advanced Materials book series that is published by Scrivener Publishing and co-published with John Wiley & Sons. Dr Shukla is also serving as associate editor for the International Journal of Advanced Engineering & Global Technology and is a consulting editor for Reports in Electrochemistry. |
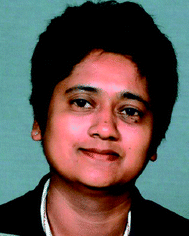 Penny P. Govender | Dr Penny P. Govender is a senior lecturer in the Department of Applied Chemistry, University of Johannesburg. Dr Govender received her PhD in 2013 at the University of the Witwatersrand, South Africa. She has extensive experience in academia and is currently the Molecular Modelling Group leader in the Department. Her research areas include bioinorganics, computational chemistry, numerical modelling, quantum chemistry and material science. She has published papers in high-impact journals and has presented her work on both national and international platforms. |
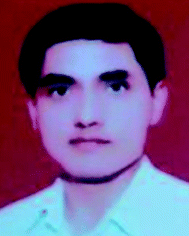 N. G. Giri | Dr N. G. Giri is an assistant professor in the Department of Chemistry, Shivaji College, University of Delhi, India. Dr Giri received his PhD degree in 2008 from the University of Delhi, India. His research areas include bio-organics, catalysts, and material science. He has published 10 papers in high-impact journals and has presented his work on several platforms. |
1. Introduction
Biodegradable polymers (BPs) are an important class of materials, and have been used for the restructuring of missing or defective organs since ancient times in both India and China.1–3 They can constitute different body organs, as well as act as biochemical regulating agents, and degrade into simpler byproducts after use.4–6 Thus, BPs with a variety of molecular architectures have been widely used as therapeutic agents with tunable properties.7 The importance of this field can be assessed from the continuous ascending trend in publications over the past decade [Fig. 1].
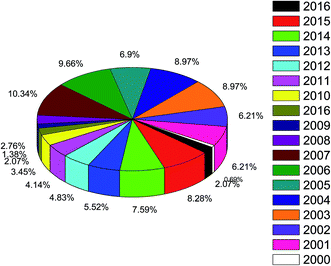 |
| Fig. 1 Schematic of publications on biodegradable polymeric nanoparticles (BPNs) from the period 2000 to 2016 (source: SciFinder May 2016). | |
BPs do not work in isolation, but rather in an organized format like macromolecular complexes, which in comparison have become sufficiently distinctive to function as organelles.8–10 This concept of synergism has initiated the close interaction between macromolecular science, cell biology, pharmacy, pharmacology, and medicine to develop suitable nanostructures for different medical applications.11–15 The recent innovation of nanoscale biopolymeric structures yields a wide spectrum of BPNs for bone replacement, surgical materials, and plasma expanders.16–19 These applications have drastically changed modern medical sciences and have shown the great potential of BPNs in medical applications.20–22 Past decades have already witnessed enormous development in the chemical engineering of BPNs for different medical applications.23,24 Some time ago, non-biodegradable polymers were also chemically engineered to make them biodegradable for different healthcare applications.25
Kundu et al. reported the preparation of nanocomposites of polystyrene and calcium sulphate (CaSO4) nanorods with different loadings by mixing pre-synthesized biopolymer (gum ghatti) and biosurfactant (rhamnolipid and surfactin). This combination resulted in higher biodegradation efficiency, as compared to the nanocomposite, gum ghatti-graft-CaSO4.26 A schematic representation, together with data (Fig. 2 and Table 1), reveals the use of BP-based nanostructures in biomedical sciences.
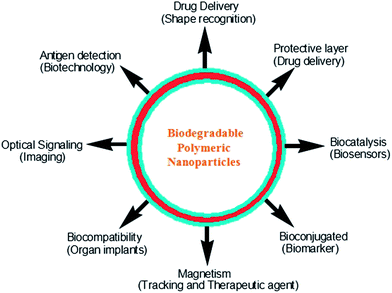 |
| Fig. 2 A typical schematic of BPNs for biomedical applications. | |
Table 1 BPNs and their biomedical applications
S. no. |
Biopolymeric nanostructure |
Application |
1 |
Core–shell structures |
Drug delivery |
2 |
Nanoparticles |
Biopharmaceuticals |
3 |
Membrane |
Organ implants |
4 |
Layer-by-layer polyelectrolyte coatings |
Pharmaceutical coatings |
5 |
Magnetic core surrounded by a polymeric layer |
Tracking and tissue separation |
6 |
Micellar nanoparticles |
Drug delivery |
7 |
DNA-based nanoprobe |
Biomarkers |
8 |
Doped transparent BPNs |
Cosmetics (sunscreen lotion) |
9 |
Metal nanocrystals containing BPNs |
Bandages |
10 |
Bioconjugated semiconductor quantum dots |
Luminescent biomarkers |
11 |
Nanostructured porous membrane |
Bio-filtration |
Recently, the combination of diagnosis and therapy has yielded a new field classed as theranostics. BPNs play an important role in diagnosis for the treatment of different types of diseases, in medical imaging, biomarkers, biosensors, nanomachines, nano-robots and nano drug delivery systems.27–29 The BPNs are also functionalized with active macromolecules to enhance their multifold applications.30,31 Thus, functionalized drug-loaded BPNs bind in high density to specific diseased sites for the imaging and killing of the respective infected cells.32 BPNs are far superior to conventional drugs, which are orally administered in a patient in either capsule or liquid forms, and which are not always effective in targeting specific cells for treatment. However, BPNs have been chemically engineered to develop highly selective and stable properties for advanced applications in therapeutics. Yang et al. reported layer-by-layer coated citral, the coating of the interfacial layer being an effective way to improve the stability of citral during storage.33,34
BPN-based drug-delivery systems, tissue engineering, cosmetics, and implants are being widely investigated.35,36 Moreover, these have become a key area in the field of pharmaceutical science for the treatment of various fatal diseases.37 They have the capability to deliver numerous drugs and genes for a sustained period of time at a specific site in the body, due to controlled degradation. In another development, nanoparticles interact with proteins present in biological bodies and produce a protein-rich layer called the “protein corona (PC)”.38,39 The composition and structure of the PC in terms of type, abundance, and conformation of the proteins can have a deep impact on the biological fate of NPs, affecting their recognition by the immune system, and targeting properties, cellular uptake, and cellular response.40 Presently, the PC is a topic of interest and is required to accurately interpret the interactions between nanoparticles and cells, being used in different biomedical applications, such as drug release and diagnostics. In this review article, we present an overview on BPNs used in biomedical science. We focus on the properties, the strategies of various methods used for fabrication of BPNs, and their functionalization steps suitable for different biomedical applications, along with clinical advances and ethical safety issues.
2. Classification of BPNs
Biodegradable polymers can be classified on the basis of polymerization methods, material composition and geometry [Fig. 3]. However, nanoconfinement predominantly depends on dimensional stability.
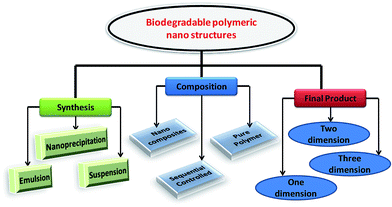 |
| Fig. 3 Classification of biodegradable polymeric nanostructures. | |
Therefore, BPN-based final products can be (i) one-dimensional (nano wires, rods, belts, and tubes), (ii) two-dimensional (mono- or multi-layered sheets, films, coatings, and tapes) and (iii) three-dimensional spherical nanocrystals, dendrimers, micelles, aquasomes, and core–shell structures. The details of the properties for an individual class of BPNs can be viewed in recent literature.41,42 Furthermore, BPNs are fine-tuned for surface behaviour and functionalised by controlling monomer sequences and layer-to-layer thicknesses.43 The sequential control of the monomer is also widely used to change different properties, such as hydrolysis, degradation, and surface energy. Li. et al. reported that the sequence control of the monomer can result in a drastic change in the degradation profile, bioavailability, and ζ-potential, which plays an important role in drug delivery. The hydrolysis rate for a poly(lactide-co-glycolide) (PLGA) with alternating sequence exhibits dramatically different hydrolysis behaviour compared with random analogues. The hydrolysis profile was nearly linear after a small initial weight loss.44 Similarly, synthetic polymers were copolymerized with other monomers to optimize their amphiphilic properties and complex behaviour. This generates various conformational transitions and assemblies to form structures with stability and responsiveness, suitable for drug carriers.45 Ganesan et al. demonstrated that interplay between compositional polydispersity and overall blocking of the polymer sequence plays a significant role in determining the morphologies, phase behaviour, and interfacial activity of gradient copolymer architectures.46,47 Fig. 4 provides an illustration that demonstrates different types of chemically engineered BPNs.
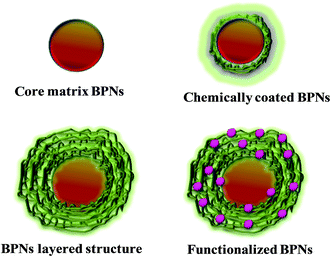 |
| Fig. 4 Illustration of various chemically engineered BPNs. | |
BPs present in different forms, either cross-linked or covalently linked onto surfaces with reversibly altered physico-chemical characteristics against external stimuli, is an important area of research.48 These are referred to as functionalized biodegradable polymer nanostructures (FBPNs); for example, light-responsive hydrogels incorporating gold nano-shells and polymer-supported liposomes for cell membranes were used for targeted drug/gene delivery.49,50 This type of system can be synthesized via grafting or surface complexation with specific macro-molecules, such as antibodies, aptamers, peptides, and polysaccharides. Natural polysaccharide-based FBPNs offer interesting alternatives to many complex and synthetic nanostructures. They have been used as drug-delivery vehicles with properties customized towards specific applications.51 The matrix-based FBPNs are formed via interactions between several polymer chains, which produce a net-like complex with interchain pores (sponge-like structures also referred to as nano-sponges), suitable for the gradual and sustained release of the entrapped drug within the body.52,53 Furthermore, BPNs coated with different asymmetric inorganic-based phospholipid membranes, such as poly(diallyldimethylammonium chloride) and poly(sodium 4-styrenesulfonate)54 systems, have found suitable matrices for use in membrane recognition and signal transduction processes, as well as controlled drug delivery.55 Alginate, chitosan, gelatin, poly(lactic acid), poly(ε-caprolactone) and poly(lactic-co-glycolic acid) are among some polymers used in the formulation of FBPNs, which are suitable vehicles for drug, protein, and gene delivery.56 Some important challenges in BPN systems are stability, solubility, and pharmacokinetic properties, which still need extensive investigation.57 The shelf life, aggregation, leakage, and toxicity of materials used to make BPNs impose further limitations.58
2.1 Core–shell nanoparticles
Core–shell nano-particles (CNPs) are composite nanostructures, usually with a concentric spherical shape. They comprise two different components, an inner core and an outer shell.59 The class of BPNs combines the characteristics and properties of shell and core, where the surface properties of the shell are translated to the core, imparting new functionality to the CNPs.60 The choice of shell materials is strongly dependent on the applications. CNPs have been found to have a large number of promising applications in the biomedical field.61–64 The schematic structure of a CNP is provided in Fig. 5, with their respective applications.
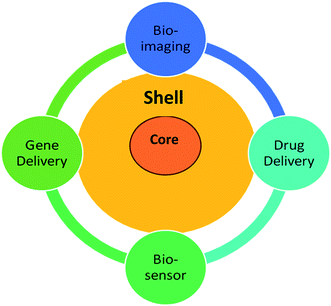 |
| Fig. 5 Illustration of CNP for different therapeutic applications. | |
The therapeutic advantages of CNPs are due to low cytotoxicity,65 high compatibility, improved stability, intracellular uptake, predictability for controlled drug release, and entrapment of multiple therapeutic agents.66–70 Further layer-by-layer (LBL) modified CNPs achieved the desired characteristics for specific applications.71–75 In this context, a polyelectrolyte multilayer coating provides a selective barrier for the diffusion of the exterior. The consequent release of proteins from the core–shell can be controlled by polyelectrolyte compositions. Such systems are highly suitable for drug delivery, with controlled-release properties.76–78 Most CNP-based drug delivery research has focused on cancer therapy, but nowadays, diabetes and cardiovascular diseases are in the immediate line of interest since they all originate from malfunctioning of certain tissue regions or cells. For example, diabetes is related to the malfunctioning of pancreatic β-cells, hence, targeting these cells for drug therapy could provide a precise and permanent cure for this problem. Chen et al.79 successfully prepared chitosan/β-lactoglobulin core/shell nanoparticles as a nutraceutical carrier for nutrient delivery, increasing the permeability of the molecules with increasing gastric residence time and providing environmental stability (that normal food processing lacks). This encapsuled nutrient system was also considered safe for oral administration.
2.2 Liposome
A ‘liposome’ is an artificially-prepared spherical ‘vesicle’ having at least one lipid bilayer with an amphiphilic nature and a diameter from 100 nm to several microns. Liposomes are prepared through the following steps: (a) drying down lipids from organic solvents, (b) dispersing the lipid in aqueous media, (c) purifying the resultant liposome and (d) analyzing the final product.80 They can be classified on the basis of their size (small, large, or giant vesicles), number of bilayers (uni- and multi-lamellar),81 and charge (neutral, anionic, or cationic).82 The dual characteristics of liposomes include hydrophilic and hydrophobic characteristics as well as biocompatibility.83 Their internal aqueous compartments work as nanoscale reaction chambers.84 A hydrophobic drug can be embedded in the lipid bilayer, while a hydrophilic one can be encapsulated in the central aqueous cavity. Liposomes have also been categorised with respect to their function, such as conventional, pH-sensitive, stealth, ligand-targeted, long-release, and triggered-release. At present, new developments have been observed in the area of liposome-based drug vehicles, exploring clinically approved products for new experimental applications, with gene delivery and cancer therapy. Some commercialized liposomes for clinical applications are given in Table 2.
Table 2 Liposomal drugs approved for clinical application
Active drug |
Product name |
Indicatora |
In different countries, the same drug could be approved for different indications, or be at different phases of clinical trials. |
Daunorubicin |
DaunoXome |
Kaposi's sarcoma |
Doxorubicin |
Mycet |
Combinational therapy of recurrent breast cancer |
Doxorubicin in PEG-liposomes |
Doxil/Caelyx |
Refractory Kaposi's sarcoma; ovarian cancer; recurrent breast cancer |
Amphotericin B |
AmBisome |
Fungal infections |
Cytarabine |
DepoCyt |
Lymphomatous meningitis |
Vincristine |
Onco TCS |
Non-Hodgkin's lymphoma |
Lurtotecan |
NX211 |
Ovarian cancer |
Nystatin |
Nyotran |
Topical antifungal agent |
Platinum compounds |
Platar |
Solid tumors |
Liposomes have the advantages of biocompatibility, biodegradability, low toxicity, and the capacity for modification of surface and size.85 However, a drawback associated with the use of liposomes is their fast elimination from the blood and accumulation by the cells of the reticulo-endothelial system, primarily in the liver. A number of developments have aimed to reduce this problem. To increase liposomal drug accumulation in the desired tissues and organs, the use of targeted liposomes with surface-attached ligands capable of recognizing and binding to cells of interest has been suggested. Polyethylene glycol (PEG) is a non-toxic polymer and has promising applications in pharmaceuticals and particularly in food additives. PEG-based liposomes focus on attaching PEG in a removable fashion to facilitate liposome capture by cells after PEG-liposomes have accumulated at the target site, through the enhanced permeability and retention (EPR) effect.86 This initial achievement with liposome-based drugs has stimulated further clinical investigations. Recently, the selective delivery of the anticancer agent, doxorubicin, in a PEG liposome for the treatment of solid tumors in patients with metastatic breast carcinoma, have resulted in subsequent improvement in patients' survival.87–89 The PEG coating is detached under the action of local pathological conditions (e.g. decreased pH in tumors). Murrel et al. investigated the effect of the adhesion of liposome and the role of membranes in the generation of mechanical stresses on cellular length scales. They also found that the modulation of hydrostatic pressure due to membrane tension and adhesion can be channeled to achieve a particular mechanical performance in a biological environment.90
2.3 Dendrimers
Dendrimers are novel three-dimensional globular hyperbranched polymeric nanostructures with sizes of ∼5–10 nm. They are non-toxic, non-immunogenic, and biopermeable, allowing them to target a specific structure in their use as therapeutic agents. These structures are a promising material for drug delivery because of their narrow polydispersity and ability to be designed with many combinations in respect to size, shape, and surface chemistry.91 The first dendrimer molecule was introduced in 1980 by Donald Tomalia and his co-workers. After that, dendrimers were extensively exploited for different medical applications and are also referred to as “Polymers of the 21st century”.92 Dendrimers contain a chemically addressable group called the focal point and often adopt a spherical three-dimensional morphology.93 A perfect dendrimer has monodispersity, a defined molecular size, and a defined number of end groups. Dendrimers contain a shell structure, structural precision, a hydrophilic or lipophilic balance, an accessible molecular surface, and good flexibility, making them potentially suitable for biomedical applications. Moreover, the photophysical, photochemical, electrochemical, and catalytic functions of dendrimers have attracted the attention of many researchers.
Generally, the growth of dendrimers starts from a central core of a molecule and proceeds in an outer direction through a chain of polymerization reactions. Therefore, precise control of size and shape can be achieved by tuning the degree of polymerization. The surface groups of dendrimers are amenable to modification and can be tailored for specific applications. The chemical modification of dendrimers can be tailored to nanomedical applications by attaching therapeutic and diagnostic agents on the surface.94 As dendritic polymers have analogous properties to proteins, enzymes, and viruses, it is easy to functionalize dendrimers. Therefore, nanotechnology has propagated dendrimers as advanced tools in therapeutics with different products under trial, for example SPL7013 dendrimer-based Viva Gel.95 SPL7013 is a polylysine dendrimer with anionic sulfate surface functional groups demonstrating antiviral activity against both HIV and Herpes Simplex Virus (HSV), similar to the polyanionic polymers that were used in the first generation of vaginal microbicides.96 Tyssen et al. found that SPL7013 binds to the HIV glycoprotein envelope (gp120), and HIV gp120 binds to receptors on cells, inhibiting viral attachment.97,98 A combinatorially large number of smart therapeutic nanodevices can easily be synthesized from dendrimeric components performing for different rationales, such as (1) diseased cell recognition, (2) diagnosis of disease states, (3) drug delivery, (4) location reporting, and (5) reporting outcomes of therapy. Dendrimers can also be used as coating agents to deliver drugs to precise sites in the body and as time-release vehicles for biologically active agents. The acetylated poly(amidoamine) (PAMAM) dendrimers have better bioavailability, along with reduced cytotoxicity. Such dendrimers seem to be potentially useful carriers for anti-tumour drugs, with reduced toxicity. Further study reveals that a decrease in nonspecific binding was evident for surface-modified dendrimers, compared to their unmodified counterparts. These studies point out the novel strategies for minimizing PAMAM dendrimer toxicity and maximizing their trans-epithelial permeability.99
2.4 Polymeric micelles (PMs)
PMs are colloidal structures (usually, below 50 nm in diameter), in which the component molecules (e.g. amphiphilic AB-type or ABA-type block copolymers, where A and B are hydrophobic and hydrophilic components, respectively) are generally arranged in a spheroidal structure with hydrophobic cores shielded from the water by a mantle of hydrophilic groups. The properties of polymeric micelles can be readily adjusted by precise engineering of the constituent block copolymers for improving drug loading, release rate, pharmacokinetics, and tumor-targeting ability.100 The end-group segment can be modified with ligands capable of recognition of cell-specific surface receptors, providing cellular selectivity and superior intracellular delivery to the polymeric micelles. The effect of various ligands,101 including antibodies,102 antibody fragments,103 aptamers,104 peptides,105,106 and small molecules,107 installed on micelles have been confirmed to improve their targeting efficiency. The efficiency of these ligand carriers depends on design aspects, such as the density of ligands on the surface, and also on the characteristics of the selected ligand–receptor system, including the binding affinities, which may be enhanced due to the multivalent effect of several ligands on the carriers, the receptor internalization, the biodistribution and availability of the receptors, and the variable expression of the receptors, such as tumor stage.108 Micelles with specialized structures become suitable carriers for poorly water soluble drugs. Around 90% of new chemical entities generated through drug discovery have poor aqueous solubility. These classes of compounds also include many important drugs, such as anti-cancer drugs.109,110 The insoluble drugs are often characterized by poor bioavailability, rapid clearance after administration, and characteristics that are associated with low therapeutic efficacy and high toxicity.111–113 Thus, this is a big challenge for pharmaceutical research.
Drug solubility has been greatly improved because of the hydrophilic shell of the micelle, with tunable shape and size. The drugs can be directed to site-specific tissues, with enhanced permeability for tumors and inflammatory tissue for the systemic delivery of water-insoluble drugs. Loaded drugs are trapped physically within the hydrophobic cores or can be covalently linked to component molecules of the micelle. The stability of polymer micelles also affects the stability of encapsulation of guest molecules, which is a crucial condition for controlled drug-delivery applications. BPNs are used for this purpose, through self-assembly after mixing the drug with the polymers; capsules may be formed spontaneously by emulsion techniques as nanosized droplets. These nanostructures contain solid cores that are highly stable, with a relatively uniform size, and are capable of controlled drug release.114 For water-soluble polymers, drugs can be covalently bonded to increase circulation time and limit cytoxicity to normal tissues.115–117 Polymers have been refined with the addition of PEG, to avoid opsonization and increase circulation time, the use of targeting ligands, and the use of pH-sensitive or hypothermic polymer conjugates. Currently, polylactide (PLA) and poly(lactide-co-glycolide) (PLGA)-based biodegradable micelles are used for the synthesis of approved medicines, whereas many others are under clinical trials.118 It is possible that polymer micelles are disintegrated after being administered in the body due to extreme dilution, which takes them below the critical micelle concentration of the polymer. If this happens, the entrapped guest, such as a drug, can leak out quickly, which renders any strategy for site-specific transport of the micelles useless.119 Thus, concentration monitoring is an important strategy to overcome this problem. In this regard, it is reported that structural tailoring of copolymers is a new strategy for designing drug carriers of high efficiency to solve the problem.
2.5 Aquasomes
Aquasomes are recently developed drug delivery systems and are also referred to as “bodies of water”. They have a spherical shape and a size of 60–300 nm. They comprise three-layered structures (i.e. core, coating, and guest), which are self-assembled through noncovalent bonds, ionic bonds, and van der Waals forces.120 The core provides structural stability to a largely immutable solid.121,122 A typical core of an aquasome consists of ceramic, whose surface is non-covalently modified with carbohydrates to obtain a sugar ball, which is then exposed to adsorption of a therapeutic agent. The aquasome protects and preserves fragile biological molecules and thus offers an attractive mode of delivery for therapeutic agents. Aquasomes are able to overcome some inherent problems of delivery systems. These problems include suitable routes of delivery, physical as well as chemical instability, poor bioavailability, and potent side effects. Sometimes polyhydroxy sugars present in aquasomes act as a dehydroprotectant and maintain a water-like state, thereby helping to preserve the molecular conformation of bioactive molecules in the dry solid state. Their conformational integrity, as well as a high degree of surface exposure, is also exploited to target bioactive molecules, such as proteins, hormones, enzymes, antigens, and genes, at specific sites.123,124 Different peptides and proteins have been successfully delivered for the treatment of a broad spectrum of diseases, and many more are under medical investigation. The coating protects against dehydration and stabilizes the biochemically active molecules. A delivery system based on carbohydrates has been successfully utilised for the delivery of insulin, hemoglobin, antigens, and enzymes, such as serratiopeptidase. The self-assembly of a polymer in an aqueous environment is purposeful for creating smart nanostructured materials in the course of naturally occurring biochemistry. This mechanism is governed basically by three physicochemical processes: (i) the interactions of charged groups, (ii) dehydration effects, and (iii) structural stability. Kaushik and Bhat explained the mechanism of protein stabilization by trehalose (a coating material). They observed that trehalose increases the transition temperature (ΔTm) of proteins to a maximum, as indicated by the comparatively high value of ΔTm of 18.2 °C (ΔG° ∼ 4.5 kcal mol−1) for RNAse at a 2 M concentration of trehalose and pH 2.5. Trehalose proved to be one of the best candidates as a universal stabilizer because of its inertness towards protein surfaces.125 In this way, toxicity is reduced, and a hemoglobin concentration of 80% is achieved and reported to deliver blood in a non-linear manner like natural blood cells. Aquasomes are also used as vaccines for delivery of viral antigen and immune deficiency virus to evoke the correct antibody. These types of vaccine therapy must be triggered by conformational specific target molecules. Aquasome-based pharmaceutical delivery systems have been developed because the drug activity increased by 60%, but unfortunately the administration and toxicity of the system has not yet been reported.125 Some representative FDA-approved proteins transferred through aquasomes are plasminogen activator, dithranol, and piroxicam.126–129
3. Overview of the preparative methods
BPNs can be prepared either from preformed polymers or by polymerisation of monomers.130 The formation of polymeric nanostructures comprises two steps: (a) polymerisation into the nano-range and (b) stabilization of the resultant particles.131,132 An illustration of different preparative routes for BPNs is shown in Fig. 6.
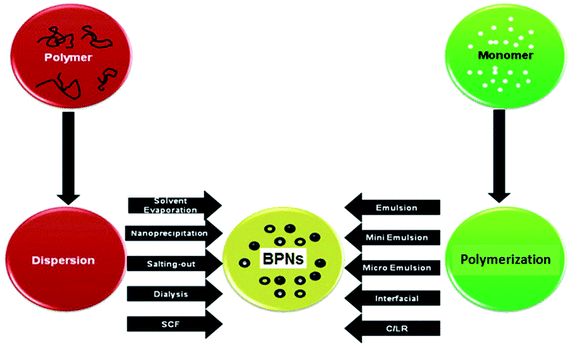 |
| Fig. 6 A schematic of different methods for the preparation of polymeric nanostructures. | |
A preparative method is selected according to the type of polymeric system, area of application, and size requirement. BPNs required in biomedical fields should be completely free from impurities, additives such as monomers, stabilizers, surfactants, or traces of solvents. Thus, the rapid expansion of a supercritical solution (RESS) or rapid expansion of a supercritical solution into a liquid solvent (RESOLV) can be used because they do not utilize any surfactant or organic solvent. For micronisation purposes, methods such as solvent evaporation, salting-out, dialysis, and supercritical fluid technology have been used.133 The method of micronisation has been developed and successfully utilized to prepare BPNs by dispersing commercially available polymers. Some representative BPNs prepared in this fashion, using different dispersion methods, are listed in Table 3.
Table 3 Preparation of BPNs by different methods
Polymers |
Methods |
Size (nm) |
References |
Polylactic acid (PLA) |
Emulsion |
200 |
134 |
Poly(D,L-lactide-co-glycolide) (PLGA) |
Solvent evaporation |
>200 |
135 |
Poly(lactic acid)/chitosan |
Precipitation |
250 |
136 |
Chitosan |
Ionotropic gelation |
84 |
137 |
Chitosan/alginate |
Ionic gelation |
202 |
138 |
PLGA |
Salting out |
200 |
139 |
PLGA |
Dialysis |
84–338 |
140 |
Polycaprolactam |
Dialysis |
200 |
141 |
Cellulose |
Dialysis |
86 |
142 |
Chitosan |
Nano-precipitation |
300 |
143 |
Guar gum |
Nano-precipitation |
20–50 |
144 |
PLA |
Rapid expansion of supercritical solution into liquid solvent |
<100 nm |
145 |
The common methods for the preparation of polymeric structures by monomers are emulsion, block copolymerization, interfacial polymerization, radiation-induced polymerization, in situ polymerization chemical functionalization (IPCF), and controlled radical polymerization.146,147 The modified forms of seeded emulsion polymerization, mini-emulsion, micro-emulsion, and nano-emulsion are also used to synthesize BPNs in a range of different sizes.148–153 A different emulsion method varies their storage time and droplet size.154 Nanoemulsions are thermodynamically steady isotropic structures, in which two immiscible liquids appear in a single phase. Since, they are stable isotropic systems, careful equilibrium between three phases is essential.155 In micro-emulsion polymerization, a large amount of surfactant/co-surfactant is required in order to make smaller nanoparticles. However, addition of a surfactant limits the solid polymer content in the dispersion. The removal of surfactant from a resultant dispersion without affecting its stability is extremely difficult but not impossible. Efforts have been made to overcome this problem by continuous and slow addition (dropwise) of monomers into a micro-emulsion during polymerization. Semi-continuous addition of monomers into a dispersion via micro-emulsion polymerization was developed to make full use of the surfactant to increase the solid content.
Nanoparticles of PLGA polymers were synthesized by using an emulsion-solvent evaporation technique and were characterized with regard to their size, drug loading, and in vitro release application.156 The results revealed that the solid-state drug polymer solubility depends on the polymer composition, molecular weight, and functional end-groups (ester or carboxyl) in the polymer chains. The solid-state drug polymer solubility depends on the quantity of drug encapsulation in the BPN. Drug-encapsulated nanoparticles were present in the form of a molecular dispersion (dissolved state) in the polymeric vehicle. Besides being investigated as carriers for insoluble drugs, BPNs, such as cross-linked polyvinylpyrrolidone nanoparticles, are also used for delivery of hydrophilic drugs.157 Abraham et al. prepared stable and aggregation-free gold nanoparticle–polymeric conjugates, employing hydrogen bonding between surface-capping ligands and polymeric micelles.158 Individual gold nanoparticles were initially capped using a phosphatidylthioethanol lipid and further conjugated with a star poly(styrene-block-glutamic acid) copolymer micelle, using a one-pot preparation method resembling atom transfer radical polymerization (ATRP). Star-shaped poly(styrene-b-glutamic acid) copolymers with linear necklace-like arrays of spherical micelles in aqueous media were obtained. This class of molecular chimera was successfully utilized for the synthesis of polymer-conjugates, reinforcing the surface-capped nanoparticles and avoiding inter-particle aggregation. However, only a few polymeric systems have so far been developed for the stabilization of nanoparticles; for example, the phosphothioethanol lipid is used as a capping ligand for a new class of block copolymers, as a stabilizing micelle.159 This preparative protocol demonstrates the robust nature and capability of a new class of molecular chimera to form nanostructures and will broaden the application area of polypeptides in nanotechnology.
4. Applications as therapeutics
BPNs embody a key part of the development of bio-medical science at the molecular to nanoscale range. They bring enormous benefit to a variety of domains due to their fascinating properties. The applications are expanding exponentially, thus it is quite possible that a few important contributions may be omitted in the present review, but an honest attempt has been made to compile the roles of commercially significant BPNs in therapeutics.160,161
4.1 Strategic properties
Nanostructures have evolved from a concept of organizing molecules into a specific structure with a wide spectrum of desirable properties. This integrates nanotechnology and biomedical sciences to improve the medical outcomes of various therapeutics and diagnostics by enhancing the accumulation of the embedded active species into the target sites of diseased tissues via passive and/or active targeting.162,163 The synergism between nanotechnology and medicine has been affected by shifts in the research enterprise, which include multidisciplinary science; changing values of data, information and knowledge; and the requirement for transparency, data sharing and disruption of funding streams. Researchers have developed techniques to create complex multifunctional conjugates and coatings, targeting specific molecules, drugs, tracking moieties, and imaging agents on BPN platforms. The major research challenge in the field of BPNs is to develop appropriate biocompatibility, surface properties, and barrier and functional properties, which are essential for synthetic reproducibility, scale-up procedures, in vivo assessment, tracking and imaging. Furthermore, nano-chemoprevention by oral consumption was developed by encapsulation of (−)-epigallocatechin-3-gallate (EGCG) with nanoparticles that were electrostatically assembled from bioactive casein phosphopeptides and chitosan, which is highly biocompatible and has the ability to enhance the bioavailability of EGCG.164 In the present scenario, the development of sophisticated methodological techniques is indispensable on the part of a chemical engineer, as there is a vital need to characterize the physical and chemical properties of BPNs and their target performance. Obviously, these aspects are inextricably linked, since physical and chemical properties contribute to the biocompatibility and performance of nanomaterials. Many BPNs have been used as medicinal products in clinical routines over the last several decades, without taking into account either their specific structure or the resulting complexity of their mechanical, chemical, and pharmacological properties. BPNs have unique physical and chemical properties that potentially offer great promise. Nevertheless, these properties may also need further examination to determine product safety and attributes. The Food and Drug Administration (FDA) has invested in a nanotechnology regulatory science program to address the key scientific gaps in knowledge, methods, and particular tools necessary for regulatory assessments of nanotechnology products for healthcare applications.165 The major bottleneck in commercialization is the lack of standard ‘nano’ nomenclature; lack of precise control over nanoparticles; manufacturing parameters, and control assay. Moreover, compounds/components for nano-drug synthesis often pose problems for commercial-scale good manufacturing production (cGMP) due to the lack of quality control issues pertaining to the separation of undesired nanostructures during manufacturing, scalability complexities, and enhancing the production rate to increase the yield. However, rapid advances towards product development in BPNs continues in full swing as it influences pharmaceutical device development in biotechnology as well as healthcare industries.166,167 The most robust sectors within biomedicine are nanotech-enabled drug delivery, diagnosis, tissue engineering, nutraceuticals, and cosmetics.168
4.2 Drug delivery
This formulates the transporting of a pharmaceutical compound to a particular site of the body organ for a desired effect.163,164 The use of BPNs in drug delivery enables passive and active targeting as carriers, like a vehicle, to inflamed and diseased tissues with increased vascular leakiness, overexpression of specific epitomes, and cellular uptake.27,169,170 Many biodegradable polymeric nanostructures exist in the form of micelles, emulsions, particulates, liposomes, nanogels, and dendrimers, which are used as drug-delivery vehicles.171,172 Khan et al. developed PEG-based hyperbranched cationic polymeric structures with spherical nanoparticle morphology.173 This interaction protects the RNA against enzymatic degradation; the outcome is illustrated in Fig. 7.
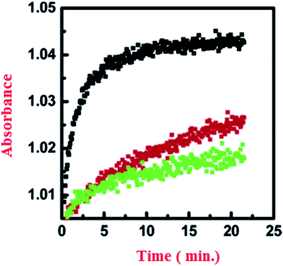 |
| Fig. 7 Degradation trends of RNA under different conditions: black – native RNA; red and green are RNA and a PNP-based complex (reproduced with permission from ref. 173. Copyright 2006 American Chemical Society). | |
Hydrophobic interactions are the leading driving force in the assembly of amphiphiles in aqueous media, when their concentrations exceed the critical micelle concentration (CMC). The majority of nanostructured micellar delivery systems are made up of amphiphilic polymers, which consist of PEG and low-molecular-weight hydrophobic core-forming blocks. Usually, the molecular weight of PEG is higher than that of the hydrophobic core-forming block, with sizes less than 100 nm for specific uses, e.g. the outer corona component.174 Polymer-based micellar delivery systems have reduced toxicity and are thermodynamically more stable to dilution compared to classical micelles. The bio-distribution and pharmacokinetics of drugs, such as doxorubicin, cisplatin, and paclitaxel, are altered favorably to increase the circulation half-life and tumor accumulation as compared to the free drug. According to molecular architecture, micellar drug delivery systems are mainly classified into four kinds: (i) phospholipid micelles comprise a lipid bilayer-coated particle to deliver drugs with a promising future for high drug delivery. The ‘head’ of a phospholipid micelle is hydrophilic (water attracting), while the ‘tail’ is hydrophobic (water repelling) and is forced to aggregate. When it is placed in water, it forms a variety of structures, depending on the specific properties of the phospholipid used. Dubertret et al.175 have reported nanocrystal-encapsulated phospholipid block-copolymer micelles for in vitro and in vivo imaging, which act as a fluorescence probe after conjugation with DNA. The micelles were nontoxic (5 × 109 nanocrystals per cell), autonomous, and slow to photobleach. (ii) Pluronic micelles are block copolymers composed of poly(ethylene oxide)-block-poly(propylene oxide)-block-poly(ethylene oxide) (PEO-PPO-PEO) with temperature-responsive characteristics. They are generally non-biodegradable, but their hybrid form shows considerable biodegradability.176 PEG grafting of PEI for subsequent condensation of nucleic acids (NAs) does not necessarily result in the formation of a PEI/NA core with a PEG corona, but in the presence of PEG interference; this can happen due to electrostatic interaction between PEI and NAs. This produces multilayered biodegradable micelles, which solves the critical drawbacks associated with current PEI-based systems.177 Pluronic micelles self-assemble into spherical micelles, having a core that is presumably dominated by PPO middle blocks, surrounded by a corona of hydrophilic PEO side blocks in aqueous solution above a certain critical micelle temperature (CMT).178 They initially had a hollow nanocapsule structure below the CMT, but they collapsed at a certain concentration and are therefore suitable as drug carriers. The effect of high-frequency ultrasound on pluronic micelles has been studied for the intracellular release of doxorubicin (DOX) for promyelocytic leukemia HL-60 cells, drug-sensitive ovarian carcinoma and multidrug-resistant (MDR) cells (A2780 and A2780/ADR respectively), and breast cancer MCF-7 cells.179,180 (iii) Polyester micelles are composed of co-polymers, such as PEG–poly(lactic acid) (PLA), PEG–poly(lactic-co-glycolic acid) (PLGA), and PEG–poly(caprolactone) (PCL), which are biocompatible and biodegradable in nature. These micelles have been applied to the parental delivery of taxanes with varying degrees of success. Polyester micelles possess a unique core–shell structure generated through the self-assembly of amphiphilic copolymers in aqueous media. The polyester-based nanoparticles possess comparatively greater stability and drug-targeting capacity, but they frequently display a significant burst effect, as a drawback.181 (iv) Poly(L-amino acid) micelles are prepared for their potential for pH-dependent release at diseased organs, such as tumor sites. Poly(L-amino acid) block copolymers or poly(L-amino acid) backbones with hydrophobic substituents self-assemble into micelles and vesicles, leading to promising drug delivery capabilities.182 The nature of the self-assembly depends on the chemistry of the constituent molecules. Micelles loaded with drugs form polymer–drug conjugates, while supra molecular aggregates have been employed as drug solubilisers, tumour targeting agents, gene delivery vectors, and facilitators of intracellular drug uptake. Polymer-based nanoparticles made from copolymers are able to optimise circulation half-life and reduce uptake and inactivation.183 Poly(lactic acid) (PLA), poly(glycolic acid) (PGA), poly(lactic-glycolic acid) (PLGA), poly-e-caprolactone (PC) and chitosan nanoparticles are the most studied co-polymers due to their biocompatible and nontoxic nature for humans.184–186 PLA and PLGA degrade by hydrolysis to lactic acid and glycolic acid, which are metabolites via natural pathways in the body. BPNs have been extensively advocated as particulate carriers in the pharmaceutical and medical fields because of their promising role as drug delivery systems, due to their controlled and sustained release properties, subcellular size, and biocompatibility with tissues.
Alternative approaches for drug delivery systems include dispersion of drug particles in the nano-size range, such as an emulsion in different solvents. Nanosuspensions of drug particles are produced by two different methods. The first method involves the breaking down of larger particles to nano-size using high-pressure homogenization of drug suspensions in the presence of surfactants.187 The second method involves crystallization (building) of the nanoparticles from the supersaturated solution state.188 The nanosuspensions are chemically more stable, provide a high drug-loading capacity, and are especially useful for therapeutic compounds that need a high dose. The polymer-based stabilizers and surfactants (e.g. PEGlycolated phospholipid) covering the surface of the nanocrystals provide steric or ionic stabilization of drug particles against aggregation. Polymer composite-based hydrogels have been developed to release drugs in a temperature-responsive manner. A dual-layered hydrogel was further developed to prepare a protein delivery system with a better sustained release. These findings have improved composite hydrogel systems by tailoring desired release profiles.189 It has also been recognised that structural aspects have profound influences on cell function, fate, and tissue formation.190–192
Macromolecule-based core–shell nanoparticles find increasing applications in the biomedical and pharmaceutical areas, mainly to overcome the limitations of poorly soluble drugs or long-acting injectable formulations and specific drug-targeting options.193 They can provide controlled release properties due to the biodegradability, pH, and ion and/or temperature sensibility of polymers (stimuli-responsive polymers). Polysaccharide-based core–shell nanoparticles improve the utility of encapsulated drugs and reduce their toxic side effects.194 At present, such nanoparticles have been widely used to deliver expensive drugs, polypeptides, proteins, vaccines, nucleic acids, and genes. It is noteworthy herein that artificial viruses195–198 are promising complex gene delivery systems, which today remain in their infancy with the most prominent limitation to date being stability in vivo, and intracellularity.199 Briefly, they consist of a cationic core (plasmid DNA/functional peptides) and an anionic shell (targeting ligands can be attached for internalization via endocytosis). Nonetheless, the modern trend in advanced nanostructured drug delivery systems seems to maintain focus on (a) combination of polymeric materials to obtain suitable and proper drug release profiles and kinetics, (b) optimisation of drug encapsulation efficiencies and loading capacities, (c) shell modification to enhance specific targeting capabilities, and (d) optimization of preparatory techniques for clinical use and industrial production. To this end, numerous polymeric materials, including PGA, PLA, chitosan, alginates, the poly(acrylic acid) family, proteins, and polypeptides (e.g. gelatin and fibrin) have been investigated.
Microcapsules made up of biological macromolecules are of interest to the scientific community because of technological reasons.200 Electrostatic interactions between polycations and polyanions form a multilayered microcapsule shell to control the loading and release of charged drug molecules. Oppositely charged biopolymers were alternately deposited onto the synthesised particles using electrostatic layer-by-layer (LBL) self-assembly, and glutaraldehyde was used to cross-link the multilayered shell structure for drug delivery application. Micron-sized calcium carbonate (CaCO3) particles were synthesised and integrated with chondroitin sulfate (CS) through a reaction between sodium carbonate and calcium nitrate tetrahydrate solutions containing suspended CS macromolecules. These microcapsules, integrated with CS inside the multilayered shells, were obtained after decomposition of the CaCO3 templates. The integration of a matrix permitted the subsequent selective control of drug loading and release. The CS-integrated microcapsules were loaded with a model drug, bovine serum albumin (BSA), labeled with fluorescein isothiocyanate (FITC-BSA), and it was revealed that the pH was an effective means of controlling, loading, and releasing FITC-BSA. Such CS-integrated microcapsules may be used for controlled localised drug delivery as biodegradable devices, which have advantages in reducing systemic side effects and increasing drug effectiveness, besides being investigated as carriers for insoluble drugs.201,202
Protein corona on nanoparticles are an important consideration for effective drug release. Thus, the properties of the nanoparticles are under intensive research and the subsequent conditions for protein corona formation significantly control the drug release profile.203 Moreover, the protein corona significantly reduces the burst effect of protein-conjugated nanoparticles or carriers with surface-loaded drugs, thus allowing them to be used to load therapeutics.204 Furthermore, the rate of passive release can be manipulated by varying the corona composition around nanoparticles. It was revealed that the effect of buffer strength and human serum concentration plays an important role in passive release. Cross-linked hydroxyethyl starch (HES) nano-capsules, with a size of 170–300 nm after coupling a folic acid conjugate, serve as a model targeting agent, due to the high affinity of folic acid to a variety of human carcinoma cell lines, which overexpress the folate receptor on the cell surface.205 The primed HES-based nano-carrier is biodegradable, when PEGylated and modified with mannose, and able to target dendritic cells (DCs) due to the influence of the protein corona.206
4.3 Diagnosis and detection
Qualitative and quantitative estimation has had a major impact in the medical field over many decades.207,208 The current constraint in developed techniques is to enhance spatial resolution and contrast, such as in functional magnetic resonance imaging (MRI). In this context, polymer nanotechnology has the potential to transform the field of medicine with biomacromolecules because of its novel opportunities for sensing clinically relevant markers, molecular disease imaging, and use as tools for therapeutic intervention.209 A different nano-dimension system had afforded the possibility of intracellular imaging through attachment of quantum dots (QDs) or synthetic chromophores for selected polymeric molecules. For example: by incorporating naturally occurring fluorescent proteins in optical techniques, nano-sized biochips can be prepared for the detection of specific bio-molecular interactions for molecular diagnostics. Further advances in polymer-based nanosensors for fluorescence resonance energy transfer are capable of detecting low concentrations of DNA in a separation-free format. This uses QDs linked to DNA probes and, in this system, a fluorescence resonance energy transfer donor–acceptor ensemble forms as a result of the binding of the target strand to a dye-labeled corresponding strand. In addition, BPNs also function as a concentrator that amplifies the target signal by confining several targets in a nanoscale range. The new probes, based on bioluminescence resonance energy transfer, convert chemical energy into photon energy, resulting in a vivid increase in fluorophore excitation, as well as reductions in the effects of tissue autofluorescence. The conjugates can be prepared by coupling QDs to a bioluminescent protein. Compared with existing QDs, self-illuminating QD conjugates have greatly enhanced sensitivity.210
The chronic disease, diabetes mellitus, is caused by the defective action and/or under-secretion of insulin, aggravating complications in the body, such as neuropathy, retinopathy, and cataract.211,212 Polymer-based nanobiosensors are being explored for the lifetime management of diabetic patients by accurate monitoring of glucose levels in the blood, in a non-persistent manner [213]. In this context, glucose oxidase (GOD) coupled to core–shell bio-macromolecule-based nanocomposites (Fig. 8) serves as a catalytic biomolecular sensor for glucose detection.71
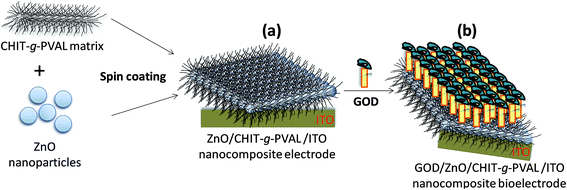 |
| Fig. 8 Illustration of (a) fabrication of ZnO/CHIT-g-PVAL core–shell nanocomposite electrode and (b) immobilization of GOD onto core–shell nanocomposite electrode. Reproduced with permission from ref. 71, Copyright 2012, Elsevier Publishers. | |
The feasibility of the original ultrasensitive protein nanoprobe systems based on self-assembled supra-molecular protein nanoparticles, which would bind antibodies as a marker of level (I) diabetes and the early phase of pancreatic β-cell destruction, can be scrutinized. Single-molecule detection for the study of molecular diversity in a pathology laboratory has been possible with BPNs.214 Furthermore, self-assembled glucose oxidase, and a metal-compound macromolecular matrix, were shown to be responsive to glucose levels, and functioned better as a biosensor with superior sensitivity and enhanced signal response.215–217 Molecularly engineered polymeric nano-surfaces are widely used for the detection of different biomolecules, such as blood constituents and DNA. A different sensing substrate has been partially successfully commercialized. A list of polymer-based biosensors is compiled in Table 4.218–232
Table 4 Polymeric nanoparticle-based biosensors
PNPs |
Analytes |
Sensing parameters |
References |
Chitosan–polyaniline |
Creatine |
Amperometric |
218 |
Alginate–pyrrole |
Glucose |
Amperometric |
219 |
Au nanoparticles/chitosan/TiO2–graphene |
α-Fetoprotein |
Amperometric |
220 |
Graphene oxide–chitosan |
DNA |
Amperometric |
221 |
Chitosan/ionic liquid–graphene composites |
Bovine serum albumin (BSA) |
Electrical |
222 |
Alginate–titanium dioxide nanocomposite |
Protein |
Electrochemical impedance |
223 |
Silver/guar gum |
NH3 |
Optical |
224 |
Ferrite magnetic/chitosan |
Glucose |
Potentiometric |
225 |
Copper nanoparticle/chitosan/carbon nanotube |
H2O2 |
Amperometric |
226 |
Chitosan/CNT |
Cholesterol |
Electrical |
227 |
Chitosan-g-polypyrrole (CHIT-g-PPy) nanomicelles |
Urea |
Optical |
228 |
Titanate nanotubes/chitosan |
Choline |
Electrochemiluminescent |
229 |
Chitosan/Ce(IV) |
DNA |
Polymerisation |
230 |
Chitosan–Au nanocomposite |
Dopamine |
SERS |
231 |
Carboxymethylcellulose/gelatin/TiO2 |
Superoxide dismutase |
Amperometric |
232 |
Recently, the integration of diagnosis and therapy has emerged as a new discipline of science called theranostics.233 This combines emerging material science and nanotechnologies in an attempt to develop novel platforms and methodologies to tackle practical issues in clinics. These attempts have become cutting-edge and potentially translatable, and this field has grown rapidly. This is advantageous and extremely attractive for personalized medicine and for the therapy of many diseases, such as cancer. Because treating cancer is not a one-size-fits-all scenario, it requires therapy to be adapted to the patient's specific biomolecules. In this concept, BPNs have an important role in studying the cell protein interaction; the change in plasma protein concentration is useful for early cancer detection. Hajipour et al.234 have demonstrated that alteration in plasma protein concentrations and structures, such as those induced by different disease states, impacts the formation of the PC. On the basis of these latest findings, Hajipour has reported the ground-breaking concept of the personalized protein corona (PPC), which has a tremendous impact for cancer detection, where many proteins are differently expressed with respect to physiological conditions. The different types of cancer or stages of cancer can alter relevant proteins.
BPNs have also been identified as biomarkers to gain an understanding of a diagnosis and in turn treat specific disorders based on a precise diagnosis. This predominantly utilizes the unique properties of nanoparticles to achieve identification and drug delivery. Nanotheranostics can be applied to non-invasively discover and target image biomarkers and further deliver treatment based on the biomarker distribution.235 For instance, depending on the surface functionalization, BPN has an increased circulation time in the blood in vivo over standard chemotherapeutics. The longer circulation half-life increases the chances for nanoparticles to extravasate from tumor blood vessels and into tumour tissues, through the leaky, underdeveloped tumour vasculature. Furthermore, nanoparticles have a high surface-area-to-volume ratio, providing a high loading capacity for imaging probes, targeting ligands, and therapeutic molecules, which can further be functionalised to become nanotheranostic. PEGylation has been applied to most nanotheranostic development to decrease the immunogenicity of the nanoparticles and increase the blood circulation half-life in vivo. BPNs, usually made up of amphiphilic polymers, provide inner cores for hydrophobic molecules, such as chemotherapeutics or imaging agents, and an external shell for ligand conjugation to target specific disease biomarkers. Choi et al.236,237 have optimised a PEGylated tumor homing polymeric nanostructure, comprised of an FDA-approved hyaluronic acid (P-HA-NPs) that can deliver hydrophobic compounds to the intracellular space of cancer cells with reduced toxicity. It clearly visualised tumours and also effectively suppressed tumor growth, as illustrated in Fig. 9a.
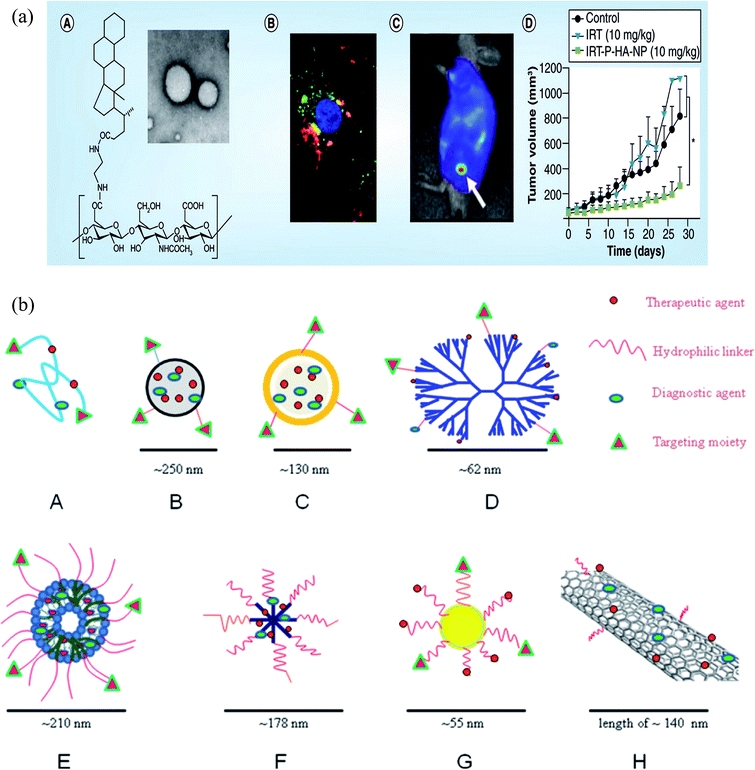 |
| Fig. 9 (a) The design of PEGylated tumour-homing BPN system (A) chemical structure of P-HA-NP. Insert and TEM image of PHA-NP, (B) selective tumour cell update and drug release of P-HA-NPs (green, P-HA-NP; red, drug; blue, nucleus), (C) near-infrared optical imaging of tumour accumulation (arrow) of Cy5.5-labeled P-HA-NPs in an HT29 colon tumour-bearing mouse and (D) therapeutic efficacy of irinotecan-encapsulated polymeric nanoparticles, IRT-P-HA-NPs. *p < 0.05. HA: hyaluronic acid; IRT: irinotecan; NP: nanoparticles.236 Copyright 2012, Elsevier Publishers. (b) Schematic of BPNS used in theranostics (A) polymer–drug conjugate, (B) polymeric nanoparticle, (C) solid lipid nanoparticle, (D) dendrimer, (E) liposome, (F) micelle, (G) gold nanoparticle and (H) carbon nanotube.237 | |
The different polymeric nanostructures used in theranostics are shown in Fig. 9b. The theranostics approach using BPNs, which target molecular biomarkers with advanced designs, is likely to contribute to increased precision for many incurable diseases like cancer.
In addition, the theranostics approach using polymer nanostructures (i.e. nano-theranostics), which target molecular biomarkers with advanced designs, also promises to contribute to increased precision in various therapeutic issues.238 Some important polymer nanostructures are listed in Table 5, with their promising features.239–246
Table 5 Polymer nanoparticles for different theranostic applications
Polymers |
Types of nanostructure |
Diseases |
Properties |
References |
SYBR |
Collapsing vapor nanobubble |
Malaria |
Optical scattering |
239 |
HSP72 protein |
HSP72 protein |
Treatment of stroke |
Fluorescence |
240 |
PLGA |
Core–shell |
Tumour |
Fluorescent imaging |
241 |
Protein |
Liposome |
Ovarian CA |
Autofluorescence |
242 |
Maleimide–PEG–poly(lactic acid) (PLA) |
PNP |
Tumor |
accumulation |
243 |
PEG–PLA |
PNP |
Brain delivery |
Biodegradable |
244 |
PEG |
Nanocomposite |
Tumor |
Biocompatibility |
245 |
Porphyrin–lipid conjugate |
Nanovesicles |
Intrinsic fluorescence |
Tunable extinction co-efficients |
246 |
4.4 Artificial implants and tissue engineering
The ultimate treatment for end-stage organ failure is transplantation. The demand for transplantation far exceeds the number of available donor organs.247–249 Organ transplantation is an interdisciplinary field, which integrates biology, engineering, materials science, and medicine. It focuses on the development of substitutes to restore, replace, maintain, or enhance tissue and organ function. Both synthetic and natural polymers are used for organ implants and tissue engineering. The synthetic polymers consist of relatively hydrophobic materials, such as α-hydroxy acids (a family that includes poly(lactic-co-glycolic)acid, PLGA), polyanhydrides and naturally occurring polymers, complex sugars (hyaluronan and chitosan), and inorganics (hydroxyapatite). The materials used in tissue engineering depend on a multiplicity of anatomical locations, cell types, and their special applications. For instance, relatively strong mechanical properties may be required in situations where the device may be subjected to weight-loading or strain, or where maintenance of a specific cytoarchitecture is required.250 Over the past few decades, continuous progress in the field of artificial organ transplants has led to intensive research on BPNs for tissue engineering. Some of them have already been used in humans (e.g. skin and cartilage), while some are at the stage of clinical trials (e.g. bladder and blood vessels).251,252 Nevertheless, most tissue engineering strategies rely on the principle that, under appropriate bioreactor conditions, cells seeded or recruited into three-dimensional (3D) biocompatible scaffolds are able to reassemble into functional structures resembling native tissues. Early artificial scaffolds were designed to provide a cell's structural integrity on a macroscopic level, but only moderate success has been achieved. However, it is widely accepted that to recapitulate proper tissue functionality, scaffolds should also establish a tissue-specific micro-environment to maintain and regulate cell behaviour and function.253
The decellularization of donor organs, such as heart, liver, and lung can provide a cellular, naturally occurring 3D biological scaffold material, which can be seeded with selected cell populations. However, true regeneration of human tissue has become increasingly plausible. Macromolecule-based nano-ceramics provides cellular adhesion, and enhances osteoblast proliferation and differentiation, combining biopolymers and bioactive ceramics that mimic bone structure as an alternative system, and creating ideal materials for elegant implants.254,255 However, it is difficult to find a single material having all the required properties. For instance, metal alloys and ceramics, such as alumina and zirconia, can be chosen for hard tissue replacement due to their mechanical competence but, due to the stress-shielding effect, a mismatch between the stiffness of the implant and the host tissue may lead to bone atrophy.
An artificial implant must possess both structural and surface compatibility with the host tissue. Particular reference to bone implants, and mechanical and physicochemical compatibility, is necessary. Materials used in orthopedic implants have their own advantages suitable for specific applications. Synthetic polymers can stimulate isolated cells to regenerate the tissues in a defined shape and size for transplantation in vivo as well as in vitro.256 Sharma et al.257 have written an exhaustive review on cartilage tissue engineering. Cartilage is a type of vascular connective tissue present in many parts of the body, such as in the joints between the bones, and the ribs, ear, nose, and discs. It consists of single cell types known as chondrocytes. Several diseases (osteoarthritis, traumatic rupture of cartilage) are also associated with cartilage, which is not easy to revamp, as cartilage possesses limited self-repair capacity due to the lack of a sufficient supply of healthy chondrocytes to the defective sites. The restoration of cartilage can be achieved by use of a suitable cell source, scaffold and growth factors. Reported materials used for cartilage scaffolds are polyhydroxyalkanoates (PHAs), collagen,258 chitosan,259 and alginate.260 The development of a 3D cartilaginous skeleton has challenged researchers for decades, as the pursuit for suitable cell sources, biomaterials, and growth factor combinations is not yet over. Implant-associated infections are the result of bacterial adhesion to an implant surface and subsequent biofilm formation at the implantation site. Formation of a biofilm takes place in several stages; a preliminary stage, with rapid surface attachment, is followed by multilayered cellular proliferation and intercellular adhesion in an extracellular polysaccharide matrix.261,262 Recently, much effort has been devoted to developing nanostructured composite materials in the form of coatings and thin layers, where bioactive compounds are embedded within an inorganic nanostructured matrix. BPNs combine with biopolymers, which mimic the organic component of the extracellular matrix of bone (e.g. collagen), and bioactive nano-ceramics to induce biomineralization.263,264 It has been previously reported that structural aspects can have profound influences on cell function, fate, and tissue formation. Carbon nanofiber/polyurethane composites have induced an improved response in osteoblasts,265 which is closely linked to the unique surface properties and high surface energy of nanomaterials. Yao et al.266 prepared a chitosan–chondroitin sulfate-blended scaffold for corneal cell culture and transplantation. These results revealed that the prepared blend had good compatibility in blended membranes. Chondroitin sulfate improved the homogeneity, crystallisation, transparency, and tensile strength, and decreased the water content of the blended membrane in a blending ratio of 1
:
0.1. Corneal cells grew and formed a confluent monolayer on chitosan–chondroitin sulfate-blended membranes (CH-CS3). A photoactive coating (photocatalyst) material was also used by Chung et al. to accelerate a chemical reaction, when irradiated with appropriate electromagnetic waves. Extensive research has been conducted on the photocatalytic mechanism, improvement of the microstructure, antimicrobial behaviour, and photocatalytic performance, leading to various environmental applications to remove contaminants from both water and air.267
Fenglan et al. reported an artificial cornea, consisting of a porous nanohydroxyapatite/poly(vinyl alcohol)hydrogel (n-HA/PVAH) skirt and a transparent center of poly(vinyl alcohol) hydrogel (PVA-H).268 The polymeric structure was used on rabbits, and an image of the respective blood vessels is shown in Fig. 10. The observation reveals the presence of new blood vessels at the surface layer of the cornea.
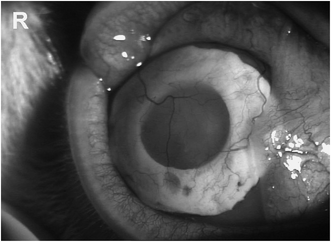 |
| Fig. 10 The blood vessels observed within the prepared skirt material of the artificial cornea. Reproduced with permission from ref. 242. Copyright 2007, Springer Publishers. | |
Nowadays, traumatic brain injury (TBI) is a major public health problem and a devastating event, as it carries high mortality and severe disability,269 but no effective clinical remedy is known to date. Nerve repair after TBI is a challenging clinical problem, which compels scientists to explore new ways of treatment of neurological function after TBI. Shi et al. accounted for the preparation of brain-derived neurotrophic factor (BDNF), i.e. a blended genipin (GP) chitosan scaffold with human umbilical cord mesenchymal stem cells (HUC-MSCs),270 investigating the scaffold for HUC-MSC cytotoxicity in vitro and determining the scaffold's effect on neural stem cell differentiation. The extracellular matrix (ECM) used to create these scaffold materials is harvested from many different tissues, including skin, small intestinal submucosa, urinary bladder, blood vessels, and heart valves.271 Gordana et al. in 2011 discussed the up-and-coming bioengineering approaches to regeneration of heart muscle as a paradigm for regenerative medicine.272 The focus was on biologically inspired strategies for heart regeneration and on how to make a ‘perfect’ heart graft. However, many challenges remain to be addressed for heart regeneration, with respect to clinical authenticity. Muthu et al.273 explained the general aspects of the different types of stimuli that can be used to modulate biological responses with stimuli-responsive polymeric (natural and as well as synthetic) systems, with an overview of their biomedical applications in tissue engineering. Furthermore, Mastrobattista and co-workers274 examined several illustrations of the most commonly-investigated in situ gelling and photo-polymerizable hydrogel-based systems, and their potential medical applications.
4.5 Cosmetics
Cosmetics are defined by the FDA as “articles intended to be applied to the human body or any part thereof for cleansing, beautifying, promoting attractiveness or altering the appearance”.275 An important trend in the formulation of cosmetics is the use of sensitive ingredients, such as vitamins or various plant-based dynamic substances. This class of materials is also referred to as “cosmeceuticals”, a term used to describe a product that fits the niche between drugs and cosmetics.276 Cosmeceuticals are the fastest growing segment in the personal care industry, and a number of topical cosmeceutical treatments, such as for photo-aging, hyperpigmentation, wrinkles, and hair damage have attracted extensive attention. In the area of cosmeceuticals, nanostructures play an important role in developing suitable ingredient materials.277,278 These ingredients need to be delivered into deeper layers of the skin to increase effectiveness. BPN-based delivery systems protect and transport these active ingredients to the targeted skin layer and have increasingly gained importance in cosmetic products.279 A variety of BPN-based delivery systems is available in cosmetics, such as liposomes, solid lipid nanoparticles, and inorganic hybrid nanoparticles. The most common nanoscale carrier system in cosmetics was introduced by Muller et al. in 2004 as a ‘liposome for anti-aging lotion’. Liposomes for cosmetics are produced from lecithin, a mixture of phospholipids and triglycerides, extracted from soya beans. Furthermore, small flexible liposomes are used to transport substances into deeper skin layers, and rigid liposomes function as a depot for substances in the upper skin layers, as they do not penetrate the skin.280 These particles can be used to prevent penetration of active ingredients into the skin, or to protect the skin against sensitizing organic substances in the cosmetic formulation.
The important features of cosmetic products are biodegradation and biocompatibility. Biodegradable cosmetics applications are superior to non-biodegradable cosmetics, because when they come into contact with the human body, they break down or metabolize from the body via normal metabolic pathways.281 Polyesters based on polylactide (PLA), polyglycolide (PGA), and their copolymers have been extensively employed as biomaterials, with degradation yielding the corresponding hydroxy acids, which are safe for in vivo employment. Biodegradable polymers, such as modified polysaccharides, are widely used in cosmetic industries.282 Lipid nanoparticles have a similar structure to that of nanoemulsions; the only difference is that the lipid core is solid and not liquid. Lipid nanoparticles are interesting materials for the formulation of cosmetics, because they improve the chemically stable active ingredient that allows controlled release, with an improvement in skin hydration and protection throughout film formation on the skin. The liquid core comprises the active component, which is further encapsulated with a polymer membrane.283 This encapsulation technology stabilizes the sensitive ingredients over existing emulsion technology and is referred to as nanocapsules. Nanocapsules are small enough to penetrate through the first layers of the skin.284 In deeper layers, the skin's enzymes dissolve the outer polymer membrane of the nanocapsules, and the active ingredient is released inside the skin. This delivery principle is of particular significance for some ingredients, such as retinol and β-carotene, which must be delivered into the deeper layers of the skin to be most effective.
4.6 Therapeutic agents
“Therapeutic agents” are a family of BPNs that uses a common active component. This includes polymeric drugs, polymer–drug conjugates, polymer–protein conjugates, polymeric micelles, polymeric non-viral vectors, and dendrimers.285,286 Polymer protein conjugates, using PEG as a polymer component, are currently the most advanced class of polymer therapeutics and are available in the market.287 They were developed to overcome the limitations of peptide protein and antibody-based drugs, such as a short plasma half-life, and poor stability and immunogenicity. The blending of nanotechnology and the multifunctional behaviour of polymers opens numerous doors for BPNs as therapeutic agents, generally for infected cells e.g. cancer cells. A correlation of the properties and potential uses of therapeutic agents is illustrated in Fig. 11.
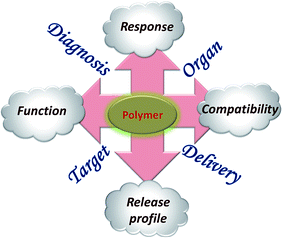 |
| Fig. 11 Schematic of polymeric material properties and potential therapeutic application. | |
In support of drug applications, polymer nanoparticles offer the advantage of controlled release, therapeutic impact, and targeted delivery. The targeting capabilities of nanoparticles depend on the particle size, surface charge, surface modification, and hydrophobicity.288 The active ingredients included in many formulations are usually hydrophobic, displaying poor water solubility, which prevents contact with cells and tissues.289 The multifunctional behaviour of macromolecules supports different therapeutic purposes, such as photodynamic therapy, based on the destruction of cancerous cells by laser-generated atomic oxygen. For this purpose, a special dye is used to generate the atomic oxygen because the cancer cells take more oxygen in comparison to a healthy cell. Consequently, a cancer cell is destroyed when exposed to laser radiation in the presence of dyes.290 Unfortunately, the remaining dye molecules migrate to the skin and the eyes, making the patient very sensitive to daylight exposure for several weeks. In order to avoid this side effect, the hydrophobic version of the dye molecule was enclosed inside porous BPNs.291,292 In this way the dye stayed trapped inside the nanoparticle without spreading, and its oxygen-generating ability was not affected because the pore size of BPN (∼1 nm) allowed the oxygen to freely diffuse out.
The clarity of the cornea (outermost covering of the eye), is critical for vision, and both acquired and genetic factors affect corneal transparency, resulting in loss of vision.293,294 Gene delivery to the cornea offers long-lasting management of corneal disorders and restoration of vision. Advances in nanotechnology have produced a wide range of BPNs for such biomedical applications.295,296
The encapsulation of tumor-associated antigens in BPNs is a promising approach to enhancing the efficiency of antigen delivery for anti-tumour vaccines. Head and neck squamous carcinoma (HNSCC) cell lines were initially used to generate tumor-associated antigens (TAA), containing a poly(lactic-co-glycolic acid) (PLGA) BPN to encapsulate efficiency and adequate kinetics release. The findings were adopted to entrap fresh tumour lysate from five patients with advanced HNSCC. This method may pave the way to a highly efficient cancer-cell elimination method with negligible toxicity. Efficient antigen (Ag) delivery is a major challenge for dendritic cell (DC)-based immunotherapy of solid-organ malignancies. The optimized formulation conditions ensure protein integrity and sustained delivery to representative tumors for 7–10 days, which is the probable life-span of an Ag-loaded DC. The extent of encapsulation of tumour antigens increases with increasing lysate concentration. Lyophilization of protein-containing lysates enables superior encapsulation and releases a spectrum of tumour antigens. The method of choice generates lysates for BPN fabrication, with increased protein encapsulation.297
Magnetic nanoparticles (MNPs) coated with temperature-sensitive polymers have also been attracting much attention as therapeutic agents because of their various potential applications in the fields of biotechnology and medicine. In particular, temperature-sensitive coated MNPs have been used extensively in controlled and targeted drug delivery.298,299 These nanocomposites are superior to the traditional stimuli-responsive systems, such as pH and temperature-sensitive polymers, due to the advantage of non-contact force (e.g. an external magnetic field). The external magnetic field is used to guide nanocomposites to a disease site and induce heat as a stimulus to control the polymer shell.300,301 The magnetic targeted carriers have been designed with dual functionality as imaging agents, as well as drug carriers. In general, these particles are capable of site-specific targeting, and controlled and sustained drug release with high biocompatibility, because of the reduction in systemic toxicity.302 Temperature-sensitive polymer PNIPAAm-coated MNPs are of particular interest, because of their stimuli-responsiveness and enhanced drug-loading ability. This BPN-based nanocomposite has more advantages when it is polymerized with acrylamide (AAm) and allylamine (AH). These co-polymer-based nanocomposites increase the lower critical solution temperature (LCST) above body temperature and provide amine groups for conjugation with bioactive molecules. Covalently coating MNPs with PNIPAAm and PNIPAAm-AAm-AH has also been studied.303 The PNIPAAm-AAm-AH-coated MNPs have a LCST above body temperature and functional groups on their surface for conjugation of biomolecules,304 which are suitable for in vitro characteristics of nanoparticles for site specific and controlled drug delivery applications. To manufacture the PNIPAAm-AAm-AH-coated MNPs, two synthetic steps were used. In the first step, metal nanoparticles were covalently bound with a silane coupling agent, vinyltrimethoxysilane (VTMS), to produce a template site for radical polymerization; in the second step, they were polymerized on the silicon layer around the MNPs via methylene-bis-acrylamide and ammonium persulfate as a cross-linking agent and an initiator, respectively.
4.7 Nutraceuticals
There is no universally accepted definition for the term nutraceuticals, but the American Nutraceutical Association in 1989 defined that a nutraceutical is any substance that is a food or a part of a food and provides medical or health benefits, including the prevention and treatment of disease. The term nutraceutical was coined by Stephen L. DeFelice. It is applied to products ranging from isolated nutrients, dietary supplements, and herbal products, to specific diets and processed food, such as cereals, soup, and beverages.305 In addition, the term nutraceutical is also commonly used to denote approaches or properties i.e. ‘nutraceutical applications’, ‘nutraceutical techniques’, ‘nutraceutical value’, ‘nutraceutical effect’, ‘nutraceutical intervention’, and ‘nutraceutical strategy’. It is a composite form of nutrients and pharmaceuticals and is demonstrated to have a physiological benefit as well as protection against chronic disease or disorder306. The main areas of the use of nutraceuticals are based on preventing cancer, improving arterial health, and lowering the risk of cardiovascular disease. Nano-engineered polymers hold great promise to provide benefits, not just within food products, but also around food products. However, there is an urgent need for regulatory systems to capably manage any risks associated with nano-foods. Many polymeric nanostructures, like lipids, polysaccharides, and protein, are intensively studied for nutraceutical applications. Lipids are a large group of natural compounds, which includes fats, waxes, sterols, fat-soluble vitamins (such as vitamins A, D, E, and K), monoglycerides, diglycerides, phospholipids, and carotenoids. Molecules such as fatty acids and their derivatives (including tri-, di-, and mono-glycerides and phospholipids), and sterol-containing metabolites, such as cholesterol, are also grouped as lipids. The main biological functions of lipids include energy storage, structural components, and cell signaling.307 A nano-encapsulation using lipids allows protection of sensitive bioactive food ingredients from unfavorable environmental conditions, eradication of incompatibilities, solubilization, or masking of unpleasant tastes or odors.308,309 Nanoemulsions (also known as mini-emulsions or submicron emulsions) are nanoscale droplets of multiphase colloidal dispersions formed by the dispersion of one liquid in another immiscible liquid by physical mixing,310 which are used to encapsulate food ingredients for appropriate delivery. Some representative lipid nanoemulsions are listed in Table 6.311–319
Table 6 List of lipid nano-emulsions used in nutraceutical applications
Polymer used |
Types of emulsion |
Active ingredient |
Application |
Reference |
Lipids |
Nanosphere |
Amphotericin B |
Delivery |
311 |
Lycorine-oleic acid |
Nano-ionic complex![[thin space (1/6-em)]](https://www.rsc.org/images/entities/char_2009.gif) |
A549 cell lines |
Tumour |
312 |
Lipids |
Nano droplets |
Fluorescent dye |
Single-particle tracking |
313 |
Oils |
Nanoemulsion |
Carotenoids |
Delivery |
314 |
Phenylephrine |
Iodinated nano-emulsions |
Induced relaxations |
Aorta |
315 |
Liposomes |
Nanocarriers |
Retinyl palmitate |
Delivery |
316 |
Sodium oleate |
Nanoemulsion |
Tocotrienols |
Anticancer activity |
317 |
PEG |
Magnetic nanoparticles |
Folic acid (FA) |
Intracellular uptake |
318 |
Chitosan and ε-polylysine |
Multilayer emulsions |
Citral |
Storage |
319 |
Rostami et al. reviewed solid lipid nanoparticle-based polymeric nanocomposites for their forefront and potential applications.320 They stated that, due to the unique size-dependent properties of the solid, lipid nanoparticles offer the possibility to develop new therapeutics. Solid lipid nanoparticle-based platforms can deliver drugs into targeted and specific tissues or cells, even in adverse pathological conditions. Obsessive–compulsive disorder (OCD) is a debilitating mental illness, which has a significant impact on quality of life. First-line selective serotonin re-uptake inhibitor (SSRI) treatments for OCD have typically limited benefit to only 40–60% of patients, and are associated with a range of adverse side effects. Current preclinical research investigating nutraceuticals for OCD reveals encouraging novel activity in modulating the key pathways suggested to be involved in the pathogenesis of OCD (glutamatergic and serotonergic pathway dysregulation). Clinical research in this area is in its infancy, and further research into nutraceuticals is warranted in the light of promising preclinical data regarding their mechanism of action, with favorable side effect profiles in comparison to current available SSRI treatments. It is recommended that future clinical trials of nutraceutical treatments for OCD utilize randomized placebo-controlled study designs and considerably superior sample sizes in order to properly test for efficacy.321 Colon cancer is a worldwide health problem and the second most dangerous type of cancer. The modern diet and lifestyles with high meat consumption and excessive alcohol intake, along with limited physical activity, have led to an increasing mortality rate as a result of colon cancer. Therefore, there is a need to focus on and develop novel and environmentally benign drug therapies for colon cancer. Currently, nutraceuticals play an increasingly important role in the treatment of various chronic diseases, such as colon cancer, diabetes, and Alzheimer's disease. Nutraceuticals are derived from various natural sources, such as medicinal plants, marine organisms, vegetables and fruits. Nutraceuticals have shown their potential to reduce the risk of colon cancer and slow its progression. These dietary substances target different molecular aspects for colon cancer development.322
5. Clinical advances
The decisive goal of the research and development of BPNs is its successful translation from bench to bedside, as presented in Fig. 12.
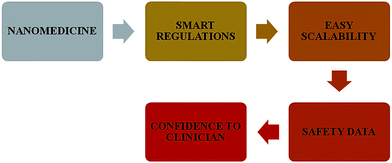 |
| Fig. 12 Schematic of transfer sequence of nanomedicine from bench to bedside. | |
However, there are significant obstacles and challenges in bringing nanomedical products to the market.323,324 The significant obstacles and challenges are: (1) scalability, (2) reproducibility, (3) lack of knowledge about the interaction between nanosystems and living tissue in vivo (e.g. behaviour as colloids in blood and interstitial fluid, receptor targeting of ligand-conjugated particles, and toxicity), (4) nanotherapeutic optimization for maximum therapeutic potential, (5) the reluctance to invest in nanotechnology, and (6) unpredictability of regulatory and safety guidelines. Furthermore, BPNs are rationally designed for a specific application to treat a specific disease. This includes the development of reasonably simple, inexpensive, and scalable protocols to prepare such BPNs, and control over the biological behaviour and pharmacological properties. In general, the real use of nanomedicine is an essential part of personalized medicine, which requires the development of multiple regulatory guidelines and appropriate training and education.325,326 The creation of an “industrial culture” during the preparation of nanomedicines, with standardized testing and characterization, is another challenge. Multiple hurdles appear before they can reach the clinics, starting from the detailed characterization and the successful manufacture of these complex constructs. Other than the standard criteria for acceptable safety and efficacy of the desirable pharmaceutical characteristics, which are applicable to most drugs, the ideal nanoparticle system or nanomedicine to be utilized for therapeutic purposes may embody the following features: critical components and their interactions, identification of key characteristics and their relation to performance, ability to replicate the characteristics, ease of production, ability to target or accumulate in the desired site of action by overcoming the restrictive biological barriers, good in-use stability, and easy storage.
The literature indicates that BPN-based medicines are currently under development to target the most common diseases,327 but nanomedicine has wide applications over most of the healthcare sector. The first generation of anti-cancer medicines are based on their “passive” delivery into tumours, drugs (such as Doxil®) and diagnostic agents such as SPION (superparamagnetic iron oxide nanoparticles)-based contrast agents being approved.328 Furthermore, despite a clear understanding that effective anti-cancer nanomedicines should specifically recognize the diseased cells, and provide an imaging signal from the affected zone to effectively deliver drugs in this specific zone, a clear set of uniform requirements for such preparation is missing from different perspectives (Table 7).
Table 7 List of different types of requisite perspectives for nanomedicine with regard to commercialization
Industrial |
Physician/pharmacist |
Health care system |
Patient perspective |
Improved utilization of pharmaceuticals |
More effective and less toxic therapeutic interventions |
Rational prescribing |
Reducing the frequency of dosage |
Reformulation by using innovative technology |
Personalized therapy |
Overall reduction in healthcare costs |
Minimally invasive method of administration |
Maximizing the return of R&D investments |
Simplified therapeutic procedures |
Improving the quality of healthcare services |
Improved therapeutic outcomes |
Providing targeted drug performance |
Reducing adverse effects |
Accelerating the healing process |
Improved quality of life of patients |
Improved patient compliance |
Improved medical/pharmaceutical care |
The rational design of such systems has yet to suggest clear guidelines for clinical translation. Another unresolved issue for the translation of BPN-based medicine is the role of nanoparticle shape and architecture in their biological behaviour and therapeutic properties. In past decades, the regulatory authorities have approved many BPN-based products for clinical use i.e. liposomes (e.g. DaunoXome®), polymer-coated liposomes (Doxil®/Caelyx®), polymeric drugs (Copaxone®), antibodies (Herceptin®, Avastin™) and antibody conjugates (Mylotarg®), polymer–protein conjugates (Oncaspar®, Neulasta®), and albumin-bound paclitaxel (Abraxane™). However, transfer from bench to clinic, including industrial scale-up synthesis, validation and regulation, and evaluation of safety and efficacy, is still a point of rigorous research. A conjugate called PK1 was a tumor-targeting polymeric prodrug to enter clinical trials in 1994. In PK1, doxorubicin is conjugated to the prototypic polymeric drug carrier, PHPMA, poly(N-(2-(hydroxypropyl)methacrylamide)), through an enzymatically cleavable tetrapeptide spacer. PK1 primarily improved the therapeutic index of doxorubicin by attenuating its cardiotoxicity. This is exemplified by the remarkably high maximum tolerated dose observed for PK1 in clinical trials, being 45 times higher than that determined for free doxorubicin.329 Abraxane is another polymeric nanoparticle-based product from American Pharmaceutical Partners, Inc., and American Bioscience, Inc. (ABI), which was approved in 2005 and is a nanoformulation of paclitaxel with albumin. This product is free of toxic solvents like Cremophor EL, which was used until now to solubilize paclitaxel in order to administer it intravenously to the patient. Many other BPN-based drugs are in the race for development and commercialization. New and exciting research data indicates that the future perspectives of biodegradable polymeric nanoparticles in nanomedicine are very promising and need to be widely commercialized. Table 8 lists some approved medicines for routine human use in the past decades, with many more currently in clinical developments.330–338
Table 8 List of recently approved nanomedicines based on polymeric nanoparticles
PNP |
Name |
Drugs |
Approval year |
Treatment |
References |
Liposomes |
Doxil/Caelyx |
Doxorubicin |
FDA (1995) |
Late-stage ovarian cancer |
330 |
Liposomes |
DaunoXome |
Doxorubicin |
FDA (1996) |
Advanced HIV-associated Kaposi's sarcoma |
331 |
Polymeric micelles |
Oncaspar |
PEG-L-asparaginase |
FDA (2006) |
Acute lymphoblastic leukemia |
332 |
Polymeric micelles |
Genexol-PM |
Paclitaxel |
South-Korea (2006) |
Metastatic breast cancer |
333 |
Conjugate |
SMANCS |
Neocarzinostatin |
Japan (1993) |
Hepatocellular carcinoma |
334 |
Polylactic acid (PLA) |
|
Dexamethasone |
|
Slow drug release for up to 50 h |
335 |
PEGylated non complex |
|
Plasmid DNA |
|
Gene therapy |
90 |
PLGA nanoparticles |
|
Docetaxel |
|
MDR of the cancer cells |
336 |
Liposome |
|
Docetaxel |
|
Cancer |
337 |
Polymeric drugs |
Copaxone |
Glu, Ala, Tyr copolymer |
Market |
Multiple sclerosis-MS |
338 |
Several studies also exploit size effects for tagging biomolecules, or use bioconjugates for labeling various cellular parts, and nano-ceramic materials for tissue engineering and orthopedics. However, the enhanced catalytic properties of surface nano-ceramics with noble metals like platinum are used to destroy dangerous toxins and other hazardous organic materials, and need to be commercialized.339–341
6. Biocompatibility and safety issues
The high reactivity of BPNs, due to their large surface-to-volume ratio, is a latent risk for all new nanosystems, which must be carefully considered as a major issue with respect to toxicology. The current protocols for new chemicals and pharmaceutical materials seem to be sufficient for these types of materials. Each new nanoparticle system has to be investigated carefully with respect to its potential side effects within human physiology and the environment. Fortunately, the public and scientific awareness of nanotechnology is high, and there is an increasing intensity of discussion on these ethical and societal issues. The clinical advances in nanotechnology also require a number of regulatory guidelines to ensure the appropriate use of new medical devices and drugs originating from nanoscience.342–346 The potential of molecular diagnostics and analysis based on nanotechnology and nanomedicine also deserves attention. This is not only related to toxicological aspects but also the improvement in the quality of life in the cases of severe diseases, cost-effective treatment of patients, artificial extension of our natural senses, and neural–electronic interface systems, which might only be available for a limited number of people. The toxicological risk to human health also includes effects during interaction with medical devices. According to the definition of the EU Medical Devices Directive, “medical devices” comprise tools for (i) diagnosis, monitoring, treatment, or alleviation of or compensation for an injury or handicap; (ii) investigation, replacement, or modification of the anatomy or of a physiological process and (iii) control of conception, which does not achieve its principal intended action in or on the human body by pharmacological, immunological, or metabolic means, but which may be assisted in its function by such means. If one considers nanoparticles as “medical devices”, these could have a broad range of applications in the human body347,348 and should be biocompatible. However, a drawback is their potential toxicity and incompatibility, which may result in disorders such as inflammation, immunological reaction, or cancer. The mechanisms of those effects are not yet well studied. However, recent literature indicates that inhalation of nanoparticles with a size below 100 nm from air pollution can lead to the induction of pulmonary inflammation. It has been demonstrated that, in this case, the individual expression of glutathione S-transferase (GST) determines the extent of the inflammation. Interestingly, the physiological task of GST is the detoxification of reactive oxygen species, thus indicating the generation of reactive oxygen species on the particle surfaces.349,350
Comparable data were obtained in relation to the inhalation of single-walled carbon nanotubes (SWCNTs). SWCNTs have been shown to elicit inflammation in the lungs of mice, while they cause small, focal interstitial fibrotic lesions in rat alveoli;351,352 a discussion on the risks of carbon nanotubes was recently received. It is further reported that this kind of nanoparticle acts, according to their needle-like structure, in the same way as asbestos, thus indicating the same risk for handlers. In an investigation by Pulskamp and co-workers in 2007, highly purified carbon nanotubes seemed not to possess short-term toxicity and can be considered as biocompatible with cardiomyocytes in culture. However, the evidence of long-term negative effects suggested physical rather than chemical interactions.353
The cytotoxicity of several kinds of nanoparticles was reviewed by Lewinski et al. in 2008. The discussion related to manifold interactions between foreign bodies and cells.354 Nanoparticles used for oral drug delivery have been found to accumulate in the liver, and excessive immune responses may cause permanent damage.355 The accumulation of nanoparticles in cells is also well documented in the case of pulmonary fibrosis caused by asbestos fibers (asbestosis) and silicosis, a disease that comes from breathing in silica or quartz dust.356–358 Nanoparticles used for drug delivery are exposed to biomolecules in the lungs and gastrointestinal tract, or to the endothelial barrier. The contact may result in the uptake of nanoparticles through endocytosis (mediated by receptors), membrane penetration in the case of hydrophobic particles, or by transmembrane channels, in the case of small nanoparticles below 5 nm.359 A strategy to prevent cellular internalization and uncontrolled cytotoxicity is to use nanoparticles less than 100 nm in diameter, with surfaces modified by hydrophilic polymers.360 In an aqueous environment, different types of biomolecules and chemicals, such as pesticides, adsorb nanoparticles. An example of the interaction of nanoparticles with biomolecules is the binding of C60 fullerenes to antibodies. Recent reports attribute the cytotoxic effect of C60 fullerene to lipid peroxidation. In organisms, all extracellular proteins, such as complement proteins or antibodies, can adsorb onto nanoparticles. During adsorption, the proteins possibly change their 3D conformation as a consequence of their reactivity, thereby resulting in an auto-immune response.361,362 To investigate the potential risks of nanotechnology, tools and methods have been developed and adapted to perform high-throughput and standardized testing of the interaction between nanoparticles, e.g. biological barriers. An established method of proving the integrity of biological barriers is the measurement of the transepithelial electrical resistance (TER). This method was adopted for determining the toxicity of nanoparticles and was developed for routine application.363–368 These few examples demonstrate that the effects of nanotechnology on human health could be double-edged, similar to conventional drug exposure, but possibly based on completely different mechanisms. Many of the investigated systems so far appear to exhibit relatively few short-term risks. Nevertheless, since all new technologies may bear hidden risks, systematic risk assessment in parallel to technological development has to be done to keep the potential hazards as low as possible.
7. Conclusion and future perspectives
The present review described biodegradable polymer nanostructures, along with their possible modification, in order to optimise their properties for therapeutic applications. The various synthetic routes developed during the course of research findings are compiled, along with their significant parameters. Furthermore, the importance of BPNs in biomedical sciences is discussed under different directions, such as drug delivery, implants, diagnosis, tissue engineering, biosensing, cosmetics, therapeutic agents, and nutraceuticals. The successes and hurdles in the commercialization of BPN-based medicine have been presented, along with future challenges to be encountered by the scientific community for human society. Future advances in the implementation of BPNs in therapeutics would include the capability to scale-up without sacrificing precise control. The development of efficient methods for harvesting BPNs, along with ethical issues, needs to be prioritized. Further computational models also need to be integrated to elucidate the effects on the degradation profile, and intra- and extra-cellular trafficking of polymeric nanoparticles for increased commercialization.
Acknowledgements
The authors are thankful to Dr K. Gopalaiah, Department of Chemistry, Delhi University, Delhi-110007 for scientific discussions. The author, Sudheesh K. Shukla also acknowledges the Global Excellence and Stature (GES) Fellowship, Department of Applied Chemistry, University of Johannesburg, South Africa.
References
- C. C. Chu, Biodegradable polymeric biomaterials: an updated overview, Biomedical Engineering Fundamentals, ed. J. D. Bronzino and D. R. Peterson, CRC Press, 2015, pp. 32/1–32/38 Search PubMed.
- T. Gianluca, A. Luigi and C. Costagliola, Biodegradable polymer medical device, PCT Int. Appl., WO 2016088054 A1 20160609, 2016.
- T. B. Goudoulas, Recent Pat. Nanomed., 2012, 2, 52 CrossRef CAS.
- J. M. Lehn, Angew. Chem., Int. Ed., 1988, 27, 89 CrossRef.
- M. Elsabahy and K. L. Woo, Chem. Soc. Rev., 2012, 41, 2545 RSC.
- S. Kango, S. Kalia, A. Celli, J. Njuguna, Y. Habib and R. Kumar, Prog. Polym. Sci., 2013, 38, 1232 CrossRef CAS.
- A. Tiwari, A. K. Mishra, H. Kobayashi and A. P. F. Turner, Intelligent Nanomaterials, WILEY-Scrivener Publishing LLC, USA, 2012 Search PubMed.
- R. Subbiah, M. Veerapandian and K. S. Yun, Curr. Med. Chem., 2010, 17, 4559 CrossRef CAS PubMed.
- K. E. Drexler, Nanotechnology: From Feynman to Funding, Bulletin of Science, Technology and Society, 2014, 24, 21 CrossRef.
- A. M. Waldron, D. Spencer and C. A. Batt, J. Nanopart. Res., 2006, 8, 569 CrossRef.
- A. Klug, Angew. Chem., Int. Ed., 1983, 22, 565 CrossRef.
- J. P. Rao and K. E. Geckeler, Prog. Polym. Sci., 2011, 36, 887 CrossRef CAS.
- J. M. Lehn, Supramolecular Polymer Chemistry: Scope and Perspectives, New York, 2000 Search PubMed.
- S. A. Jadhav, Adv. Mater. Lett., 2014, 1, 20 Search PubMed.
- A. Tiwari, M. Ramalingam, H. Kobayashi and A. P. F. Turner, Biomedical Materials and Diagnostic Devices, WILEY-Scrivener Publishing LLC, USA, 2012 Search PubMed.
- S. K. Shukla, Adv. Mater. Res., 2014, 1, 2 Search PubMed.
- R. Dong, Y. Zhou, X. Huang, X. Zhu, Y. Li and J. Shen, Adv. Mater., 2015, 27, 498 CrossRef CAS PubMed.
- T. A. Doll, S. Raman, R. Dey and P. Burkhard, J. R. Soc., Interface, 2013, 10, 20120740 CrossRef PubMed.
- S. M. Moghimi, A. C. Hunter and J. C. Murray, FASEB J., 2005, 19, 311 CrossRef CAS PubMed.
- F. T. Zohora and A. Y. M. A. Azim, Eur. Sci. J., 2014, 10, 186 Search PubMed.
- B. Guo, L. Glavas and A. Albertsson, Prog. Polym. Sci., 2013, 38, 1263 CrossRef CAS.
- B. Clotilde, X. Sala, M. Eduardo, J. G. J. L. Lebouille, H. E. A. Henriette and M. G. Meesters, J. Appl. Polym. Sci., 2016, 133, 31 Search PubMed.
- K. Li and B. Liu, Chem. Soc. Rev., 2014, 43, 6570 RSC.
- H. Xu, L. Cui, N. Tong and H. Gu, J. Am. Chem. Soc., 2006, 128, 15582 CrossRef CAS PubMed.
- D. N. Ghista, Biomedical Science, Engineering and Technology, In Tech Open Science, 2012 Search PubMed.
- D. Kundu, C. Hazra, A. Chattrjee, A. Chaudhary and S. Mishra, Polym. Degrad. Stab., 2014, 107, 37 CrossRef CAS.
- A. Tiwari and H. Kobayash, Responsive Materials and Methods: State-of-the-Art Stimuli-Responsive Materials and Their Applications, WILEY-Scrivener Publishing LLC, USA, 2013 Search PubMed.
- C. A. Serra, B. Cortese, I. U. Khan, N. Anton, M. H. J. M. de Croon, V. Hessel, T. Ono and T. Vandamme, Macromol. React. Eng., 2013, 7, 414 CrossRef CAS.
- A. Tiwari, Advanced Healthcare Materials, WILEY-Scrivener Publishing LLC, USA, 2013 Search PubMed.
- R. P. Singh and J. W. Choi, Adv. Mater. Lett., 2010, 1, 83 CrossRef CAS.
- S. Gulia and R. Kakkar, Adv. Mater. Lett., 2013, 4, 876 CAS.
- A. Tiwari and A. Tiwari, Engineered Nanomaterials, CRC Press, USA, 2013 Search PubMed.
- X. Yang, H. Tian, C. T. Ho and Q. J. Huang, J. Agric. Food Chem., 2012, 60, 402 CrossRef CAS PubMed.
- M. Athar and A. Das, Adv. Mater., 2014, 1, 25 Search PubMed.
- B. Mandal, H. Bhattacharjee, N. Mittal, H. Sah, P. Balabathula, L. A. Thoma and G. C. Wood, Nanomedicine, 2013, 9, 474 CAS.
- Z. S. Haidar, Polymer, 2010, 2, 323 CAS.
- B. Haley and E. Frenkel, Urol. Oncol., 2008, 26, 57 CrossRef CAS PubMed.
- S. Tenzer, D. Docter, J. Kuharev, A. Musyanovych, V. Fetz, R. Hecht, F. Schlenk, D. Fischer, K. Kiouptsi, C. Reinhardt, K. Landfester, H. Schild, M. Maskos, S. K. Knauer and R. H. Stauber, Nat. Nanotechnol., 2013, 8, 772–781 CrossRef CAS PubMed.
- S. Zanganeh, R. Spitler, M. Erfanzadeh, A. M. Alkilany and M. Mahmoudi, Int. J. Biochem. Cell Biol., 2016, 75, 143–147 CrossRef CAS PubMed.
- S. Palchetti, D. Pozzi, M. Mahmoudi and G. Caracciolo, J. Mater. Chem. B, 2016, 4, 4376 RSC.
- B. A. Allo, D. O. Costa, S. J. Dixon, K. Mequanint and A. S. Rizkalla, J. Funct. Biomater., 2012, 3, 432 CrossRef CAS PubMed.
- I. Vroman and L. Tighzert, Materials, 2009, 2, 307 CrossRef CAS.
- S. M. Kim, S. J. Park, K. B. Gu and C.-H. Kim, Polymer, 2015, 39, 831 Search PubMed.
- J. Li, R. M. Stayshich and T. Y. Meyer, J. Am. Chem. Soc., 2011, 133, 6910 CrossRef CAS PubMed.
- J. Li, S. N. Rothstein, S. R. Little, H. M. Edenborn and T. Y. Meyer, J. Am. Chem. Soc., 2012, 134, 16352 CrossRef CAS PubMed.
- N. Angelova and G. Yordanov, Colloids Surf., A, 2014, 452, 73 CrossRef CAS.
- V. Ganesan, N. A. Kumar and V. Pryamitsyn, Macromolecules, 2012, 45, 6281 CrossRef CAS.
- A. C. Balazs, T. Emrick and T. P. Russell, Science, 2006, 314, 1107 CrossRef CAS PubMed.
- Z. S. Haidar, R. C. Hamdy and M. Tabrizian, Biotechnol. Lett., 2009, 31, 1817 CrossRef CAS PubMed.
- Z. S. Haidar, R. C. Hamdy and M. Tabrizian, Biotechnol. Lett., 2009, 31, 1825 CrossRef CAS PubMed.
- Y. Y. Yang, Y. Wang, R. Powell and P. Chan, Clin. Exp. Pharmacol. Physiol., 2006, 33, 557 CrossRef CAS PubMed.
- V. Viviana, B. Francesca, D. Santis, F. Ciccarella, G. Giannelli, G. Leporatti and L. Stefano, Curr. Pharm. Des., 2012, 18, 4155 CrossRef.
- W. Xu, X. He, M. Zhong, X. Hu and Y. Xiao, RSC Adv., 2015, 5, 3157 RSC.
- S. Inagaki, Y. Fukushima and K. Kuroda, J. Chem. Soc., Chem. Commun., 1993, 680 RSC.
- N. Sakata, T. Aoki, G. Yoshimatsu, H. Tsuchiya, T. Hata, Y. Katayose, S. Egawa and M. Unno, Diabetes/Metab. Res. Rev., 2014, 30, 1 CrossRef PubMed.
- J. H. Park, M. Ye and K. Park, Molecules, 2005, 10, 146 CrossRef CAS PubMed.
- K. T. Nguyen, J. Nanomed. Nanotechnol., 2011, 2, 5 Search PubMed.
- J. Panyam and V. Labhasetwar, Adv. Drug Delivery Rev., 2012, 64, 61 CrossRef.
- R. A. Ramli, W. A. Laftah and S. Hashim, RSC Adv., 2013, 3, 15543 RSC.
- G. R. Hendrickson, M. H. Smith, A. B. South and L. A. Lyon, Adv. Funct. Mater., 2010, 20(11), 1697 CrossRef CAS.
- Y. Gao, A. Ahiabu and M. Serpe, ACS Appl. Mater. Interfaces, 2014, 6, 13749 CAS.
- J. J. Gallagher, A. A. Hillmyer and T. M. Reineke, Macromolecules, 2014, 47, 498 CrossRef CAS.
- F. Zhang, J. Ma, Q. Xu, J. Zhou, D. Simion, G. Carmen, J. Wang and Y. Li, ACS Appl. Mater. Interfaces, 2016, 8, 11739 Search PubMed.
- V. L. Dimonie, E. S. Daniels, O. L. Shaffer and M. S. El-Aasser, Control of Particle Morphology, in Emulsion Polymerization and Emulsion Polymers, ed. P. Lovell and M. S. El-Aasser, John Wiley and Sons, West Sussex England, 1997, p. 294 Search PubMed.
- L. Liu, et al., Theranostics, 2012, 2, 705 CrossRef CAS PubMed.
- N. Sounderya and Y. Zhang, Recent Pat. Biomed. Eng., 2008, 1, 34 CrossRef CAS.
- J. L. Markman, A. Rekechenetskiy, E. Holler and J. Y. Ljubimova, Adv. Drug Delivery Rev., 2113, 65, 1866 CrossRef PubMed.
- I. P. Kaur and H. Singh, J. Controlled Release, 2014, 184, 36 CrossRef CAS PubMed.
- R. G. Chaudhuri and S. Paria, Chem. Rev., 2012, 112, 2373 CrossRef PubMed.
- Z. Sun, Q. Zhou and L. S. Fan, Langmuir, 2013, 29, 12520 CrossRef CAS PubMed.
- S. K. Shukla, S. R. Deshpande, S. K. Shukla and A. Tiwari, Talanta, 2012, 99, 283 CrossRef CAS PubMed.
- C. Zandonella, Nature, 2003, 423, 10 CrossRef CAS PubMed.
- J. S. Haidar, R. C. Hamdy and M. Tabrizian, Biomaterials, 2008, 29, 1207 CrossRef PubMed.
- C. Burda, X. Chen, R. Narayana and M. A. El-Sayed, Chem. Rev., 2005, 105, 1025 CrossRef CAS PubMed.
- S. Balakrishnan, M. J. Bonder and G. C. Hadjipanayis, J. Magn. Magn. Mater., 2009, 321, 117 CrossRef CAS.
- M. V. Salgueiri and M. A. Correa-Duarte, Adv. Mater., 2007, 19, 4131 CrossRef.
- A. Tiwari and M. Prabaharan, J. Biomater. Sci., Polym. Ed., 2010, 21, 937 CrossRef CAS PubMed.
- N. Pazyar, A. Feily and R. Yaghoobi, Indian J. Dermatol., 2013, 58, 157 Search PubMed.
- L. Chen and M. Subirade, Biomaterials, 2005, 26, 6041 CrossRef CAS PubMed.
- A. Akbarzadeh, R. Rezaei-Sadabady, S. Davaran, S. W. Joo, N. Zarghami, Y. Hanifehpour, M. Samiei, M. Kouhi and N.-K. Kazem, Nanoscale Res. Lett., 2013, 8, 102 CrossRef PubMed.
- S. Vemuri and C. T. Rhodes, Pharm. Acta Helv., 1995, 70, 95 CrossRef CAS PubMed.
- G. Storm and D. J. A. Crommelin, Pharm. Sci. Technol. Today, 1998, 1, 19 CrossRef CAS.
- L. Lesoin, O. Boutin, C. Crampon and E. Badens, Colloids Surf., 2011, A377, 1 CrossRef.
- B. Sun, D. S. Lim, J. S. Kuo, C. L. Kuyper and D. T. Chiu, Langmuir, 2004, 20, 9437 CrossRef CAS PubMed.
- S. Verma, S. Dent, B. J. Chow, D. Rayson and T. Safra, Cancer Treat. Rev., 2008, 34, 391 CrossRef CAS PubMed.
- B. Klajnert, L. Peng and V. Cena, Dendrimers in Biomedical Applications, RSC Publishing, 2013 Search PubMed.
- Z. Symon, et al., Cancer, 1999, 86, 72 CrossRef CAS PubMed.
- A. T. Perez, G. H. Domenech, C. Frankel and C. L. Vogel, Cancer Invest., 2002, 20, 22 CrossRef CAS PubMed.
- J. A. O'Shaughnessy, Clin. Breast Cancer, 2003, 4, 318 CrossRef.
- M. P. Murrell, R. Voituriez, J. F. Joanny, P. Nassoy, C. Sykes and M. T. L. Gardel, Nat. Phys., 2014, 10, 163 CrossRef CAS.
- H. L. Crampton and E. F. Simanek, Polym. Int., 2007, 56, 489 CrossRef CAS PubMed.
- B. Klajnert and M. Bryszewska, Acta Biochimca Acta, 2001, 48, 199 CAS.
- S. Ghosh, M. Dutta, S. Sahu, D. Fujita and A. Bandyopadhyay, Adv. Funct. Mater., 2014, 24, 1338 CrossRef.
- D. A. Tomalia, H. Baker, J. R. Dewald, M. Hall, G. Kallos, S. Martin, J. Roeck, J. Ryder and P. Smith, Macromolecules, 1986, 19, 2466 CrossRef CAS.
- L. C. du Toit, V. Pillay and Y. E. Choonara, Adv. Drug Delivery Rev., 2010, 62, 532 CrossRef CAS PubMed.
- R. Rupp, S. L. Rosenthal and L. R. Stanberry, Int. J. Nanomed., 2007, 2, 561 CAS.
- D. Tyssen, S. A. Henderson, A. Johnson, J. Sterjovski, K. Moore, J. La, M. Zanin, S. Sonza, P. M. Karellas, A. Robert and J. D. Freitas Jr, Nanomedicine, 2005, 1, 2 Search PubMed.
- J. F. Kukowska-Latallo, A. U. Bielinska, J. Johnson, R. Spindler, D. A. Tomalia and J. R. Baker, Proc. Natl. Acad. Sci. U. S. A., 1996, 93, 4897 CrossRef CAS.
- R. X. Zhuo, B. Du and Z. R. Lu, J. Controlled Release, 1999, 57, 249 CrossRef CAS PubMed.
- S. M. Moghimi, A. C. Hunter and J. C. Murray, FASEB J., 2005, 19, 311 CrossRef CAS PubMed.
- V. Torchilin, Expert Opin. Drug Delivery, 2008, 5, 1003 CrossRef CAS PubMed.
- W. W. Cheng and T. M. Allen, Expert Opin. Drug Delivery, 2010, 7, 461 CrossRef CAS PubMed.
- O. C. Farokhzad, J. Cheng, B. A. Teply, I. Sherifi, S. Jon, P. W. Kantoff, J. P. Richie and R. Langer, Proc. Natl. Acad. Sci. U. S. A., 2006, 103, 6315 CrossRef CAS PubMed.
- M. Oba, Y. Vachutinsky, K. Miyata, M. R. Kano, S. Ikeda, N. Nishiyama, K. Itaka, K. Miyazono, H. Koyama and K. Kataoka, Mol. Pharm., 2010, 7, 501 CrossRef CAS PubMed.
- Y. Miura, T. Takenaka, K. Toh, S. Wu, H. Nishihara, M. R. Kano, Y. Ino, T. Nomoto, Y. Matsumoto, H. Koyama, H. Cabral, N. Nishiyama and K. Kataoka, ACS Nano, 2013, 7, 8583 CrossRef CAS PubMed.
- Y. Bae, W. D. Jang, N. Nishiyama, S. Fukushima and K. Kataoka, Mol. BioSyst., 2005, 1, 242 RSC.
- T. M. Allen, Nat. Rev. Cancer, 2002, 2, 750 CrossRef CAS PubMed.
- V. P. Torchilin, Pharmaceut. Res., 2007, 24, 1 CrossRef CAS PubMed.
- R. Liu, Water-Insoluble Drug Formulation, CRC Press, Boca Raton, FL, USA, 2nd edn, 2008, p. 669 Search PubMed.
- M. Sharma, R. Sharma and D. K. Jain, Scientifica, 2016, 2016, 8525679 CrossRef PubMed.
- S. Movassaghian, O. M. Merkel and V. P. Torchilin, Wiley Interdiscip. Rev.: Nanomed. Nanobiotechnol., 2015, 7, 691 CrossRef CAS PubMed.
- S. kawabe, M. Seki and H. Tabata, Appl. Phys. Lett., 2016, 108, 081103 CrossRef.
- J. L. Markman, A. Rekechenetskiy, A. E. Holler and J. Y. Ljubimova, Adv. Drug Delivery Rev., 2013, 65, 1866 CrossRef CAS PubMed.
- C. M. Hu and L. Zhang, Biochem. Pharmacol., 2012, 83, 1104 CrossRef CAS PubMed.
- K. Cho, X. Wang, S. Nie, Z. G. Chen and D. M. Shin, Clin. Cancer Res., 2008, 14, 1310 CrossRef CAS PubMed.
- A. Srivastava, T. Yadav, S. Sharma, A. Nayak, A. Kumari and N. Mishra, J. Biosci. Med., 2016, 4, 69 Search PubMed.
- G. Mattheolabakis, B. Rigas and P. P. Constantinides, Nanomedicine, 2012, 7, 1577 CrossRef CAS PubMed.
- G. Ma, C. Zhang, L. Zhang, H. Sun, C. Song, C. Wang and D. Kong, J. Mater. Sci.: Mater. Med., 2016, 27, 17 CrossRef PubMed.
- N. Kang, M.-E. Perron, R. E. Prud’homme, Y. Zhang, G. Gaucher and J.-C. Leroux, J. Nanosci. Lett., 2005, 5, 315 CrossRef CAS PubMed.
- S. P. Vyas and R. K. Khar, Introduction to parenteral drug delivery, in Targeted and controlled drug delivery, ed. S. P. Vyas and R. K. Khar, CBS Publishers & Distributors, New Delhi, 2002, pp. 3–37 Search PubMed.
- K. Ita, Pharmaceutics, 2016, 8, 9 CrossRef PubMed.
- M. S. Umashankar, R. K. Sachdeva and M. Gulati, Nanomedicine, 2010, 6, 419 CAS.
- S. S. Jain, P. S. Jagtap, N. M. Dand, K. R. Jadhav and V. J. Kadam, J. Appl. Pharm. Sci., 2012, 2, 184 Search PubMed.
- G. Chen, I. Roy, C. Yang and P. N. Prasad, Chem. Rev., 2016, 116, 2826 CrossRef CAS PubMed.
- J. K. Kaushik and R. Bhat, J. Biol. Chem., 2003, 278, 26458 CrossRef CAS PubMed.
- A. K. Cherian, A. C. Rana and S. K. Jain, Drug Dev. Ind. Pharm., 2000, 26, 459 CrossRef CAS PubMed.
- T. Tiwari, S. Khan, N. Rao, A. Joshi and B. K. Dubey, World J. Pharm. Pharm. Sci., 2012, 1, 250 CAS.
- S. Kommineni, S. Ahmad, P. Vengala and C. V. S. Subramanyam, Drug Dev. Ind. Pharm., 2012, 38, 577 CrossRef CAS PubMed.
- E. Larraneta, M. T. C. McCrudden, A. J. Courtenay and R. F. Donnely, Pharmaceut. Res., 2016, 33, 1055 CrossRef CAS PubMed.
- K. E. Geckeler and J. Stirn, Naturwissenschaften, 1993, 80, 487 CrossRef CAS PubMed.
- J. Yun, F. Cai, F. J. Lee and W. J. Pichler, Asia Pacific Allergy, 2016, 6, 77 CrossRef PubMed.
- H. Singh and M. Kumar, Indian Pat. Appl., IN 2012DE01249 A 20151030, 2015.
- S. Rangelov and A. Pispas, Polymer and Polymer-Hybrid Nanoparticles: From Synthesis to Biomedical Applications, CRC Press, 2013 Search PubMed.
- M. F. Zambaux, F. Bonneaux, R. Gref, P. Maincent, E. Dellacherie, M. J. Alonso, P. Labrude and C. J. Vigneron, J. Controlled Release, 1998, 50, 31 CrossRef CAS PubMed.
- Y. Haggag, Y. Abdel-Wahab, O. Ojo, M. Osman, S. El-Gizawy, M. El-Tanani, A. Faheem and P. McCarron, Int. J. Pharm., 2016, 499, 236–246 CrossRef CAS PubMed.
- W. Wang, S. Chen, L. Zhang, X. Wu, J. Wang, J.-F. Chen and Y. Le, Mater. Sci. Eng., C, 2015, 46, 514–520 CrossRef CAS PubMed.
- A. Manikandan and M. Sathiyabama, Int. J. Biol. Macromol., 2016, 84, 58–61 CrossRef CAS PubMed.
- S. K. Motwani, S. Chopra, S. Talegaonkar, K. Kohli, F. J. Ahmad and R. K. Khar, Eur. J. Pharm. Biopharm., 2008, 68, 513–525 CAS.
- Y. N. Konan, R. Gurny and E. Allemann, Int. J. Pharm., 2002, 233, 239 CrossRef CAS PubMed.
- H. J. Jeon, Y. I. Jeong, M. K. Jang, Y. H. Park and J. W. Nah, Int. J. Pharm., 2000, 207, 99 CrossRef CAS PubMed.
- A. S. Faheem, A. M. B. Nasser, A. K. Muzafar, A. Santosh, S. K. Myung and H. K. Kim, J. Mater. Sci.: Mater. Med., 2009, 20, 821 CrossRef PubMed.
- S. Hornig and T. Heinze, Biomacromolecules, 2008, 9, 1487 CrossRef CAS PubMed.
- A. G. Luque-Alcaraz, J. Lizardi-Mendoza, F. M. Goycoolea, I. Higuera-Ciapara and W. Argüelles-Monal, RSC Adv., 2016, 6, 59250 RSC.
- R. S. Soumya, S. Ghosh and E. T. Abraham, Int. J. Biol. Macromol., 2010, 46, 267 CrossRef CAS PubMed.
- M. J. Meziani, P. Pathak, W. Wang, T. Desai, A. Patil and Y. P. Sun, Ind. Eng. Chem. Res., 2005, 44, 4594 CrossRef CAS.
- L. A. Fielding, J. K. Hillier, M. J. Burchell and S. P. Armes, Chem. Commun., 2015, 51, 16886 RSC.
- B. Liu, Z. Fu, M. Zhang and H. Zhang, Colloid Polym. Sci., 2016, 294, 787 CAS.
- C. M. Miller and M. S. El-Aasser, Recent Advances in Polymeric Dispersions, NATO ASI Series E: Applied Science, 335, Kluwer Academic Publishers, Dordrecht, 1997 Search PubMed.
- K. E. Geckeler and H. Nishide, Advanced nanomaterials, Wiley-VCH Publishers, Weinheim, Germany, 2010 Search PubMed.
- M. Guardingo, P. G. Monje, F. Novio, E. Bellido, F. Busque, G. Molnar, A. Bousseksou and D. R. Molina, ACS Nano, 2016, 10, 3206 CrossRef CAS PubMed.
- Y. Chen, H. Liu, Z. Zhang and S. Wang, Eur. Polym. J., 2007, 43, 2848 CrossRef CAS.
- J. P. Rao and K. E. Geckeler, Prog. Polym. Sci., 2011, 36, 887 CrossRef CAS.
- T. Kozlecki, I. Polowczyk and W. Sawinski, J. Nanopart., 2016, 2016, 8203260 Search PubMed.
- C. Vauthier and K. Bouchemal, Pharmaceut. Res., 2009, 26, 1025 CrossRef CAS PubMed.
- E. B. Souto, N. P. Aditya and R. S. R. Murthy, Pharmazie, 2011, 66, 1 Search PubMed.
- E. Locatelli and M. C. Franchini, J. Nanopart. Res., 2012, 14, 1316 CrossRef.
- D. J. Bharali, S. K. Sahoo, S. Mozumdar, A. Maitra and A. J. Colloid, Interface Sci., 2003, 258, 415 CrossRef CAS.
- S. Abraham, C. S. Ha, C. A. Batt and I. Kim, J. Polym. Sci., Part A: Polym. Chem., 2007, 45, 3570 CrossRef CAS.
- G. Salzano, R. Riehle, G. Navarro, F. Perche, G. De Rosa and V. P. Torchilin, Cancer Lett., 2014, 343, 224 CrossRef CAS PubMed.
- A. Tiwari, and A. Tiwari, Nanomaterials in Drug Delivery, Imaging and Tissue Engineering, WILEY-Scrivener Publishing LLC, USA, 2014 Search PubMed.
- V. K. Mourya, N. N. Inamdar and A. Tiwari, Adv. Mater. Lett., 2011, 1, 11 CrossRef.
- G. A. Hughes, Nanomedicine, 2005, 1, 22 CAS.
- P. Roberto and L.-S. Turng, J. Appl. Polym. Sci., 2015, 132, 42889 Search PubMed.
- B. Hu, Y. Ting, X. Yang, W. Tang, X. Zeng and Q. Huang, Chem. Commun., 2012, 48, 2421 RSC.
- Available online at: http://www.fda.gov/ScienceResearch/SpecialTopics/Nanotechnology/ucm273325.htm.
- H. M. Mansour, C. W. Park and R. Bawa, Design and development of approved nanopharmaceutical products, in Handbook of Clinical Nanomedicine: From Bench to Bedside, Pan Stanford Publishing, Singapore, 2014 Search PubMed.
- R. Bawa, Nanotechnology Law & Business, 2008, 5, 135 Search PubMed.
- O. Yuichi, T. Akihiro and N. Koji, Adv. Polym. Sci., 2012, 247, 65–114 Search PubMed.
- J. V. Gonzalez-Aramundiz, M. V. Lozano, A. Sousa-Herves, E. Fernandez-Megia and N. Csaba, Expert Opin. Drug Delivery, 2012, 9, 183–201 CrossRef CAS PubMed.
- M. Edgar, B. M. Isabel and C.-G. Catherina, Int. J. Nanomed., 2012, 8, 3071–3091 Search PubMed.
- S. Saranya and K. V. Radha, Polym.-Plast. Technol. Eng., 2014, 53, 1636 CrossRef CAS.
- S. K. Pandey, C. Haldar, D. K. Patel and P. Maiti, Biodegradable Polymers for Potential Delivery Systems for Therapeutics, Adv. Polym. Sci., 2013, 254, 169–202 CrossRef CAS , in Multifaceted Development and Application of Biopolymers for Biology, Biomedicine and Nanotechnology.
- J. A. Khan, R. K. Kainthan, M. Ganguli, J. N. Kizhakkedathu, Y. Singh and S. Maiti, Biomacromolecules, 2006, 7, 1386 CrossRef CAS PubMed.
- G. S. Kwon, Crit. Rev. Ther. Drug Carrier Syst., 2003, 20, 357 CrossRef CAS PubMed.
- B. Dubertret, P. Skourides, D. Norris, V. Noireaux, A. H. Brivanlou and A. Libchaber, Science, 2002, 298, 1759 CrossRef CAS PubMed.
- P. Alexandridis and T. A. Hatton, Colloids Surf., A, 1995, 96, 1 CrossRef CAS.
- D. G. Abebe, R. Kandil, T. Kraus, M. Elsayed, T. Fujiwara and O. M. Merkel, Protocol Non-Viral Gene Delivery Vectors, Methods Mol. Biol., 2016, 1445, 175 Search PubMed.
- K. H. Bae, S. Choi, S. Y. Park, Y. Lee and T. G. Park, Langmuir, 2006, 22, 6380 CrossRef CAS PubMed.
- A. Marin, A. H. Sun, G. A. Husseini, W. G. Pitt, D. A. Christensen and N. Y. Rapoport, J. Controlled Release, 2002, 84, 39 CrossRef CAS PubMed.
- A. D. Tagalakis, G. D. Kenny, A. S. Bienemann, D. McCarthy, M. M. Munye, H. Taylor, M. J. Wyatt, M. F. Lythgoe, E. A. White and S. L. Hart, J. Controlled Release, 2014, 174, 177 CrossRef CAS PubMed.
- G. Gaucher, R. H. Marchessault and J. C. Leroux, J. Controlled Release, 2010, 143, 2 CrossRef CAS PubMed.
- P. F. Gu, H. Xu, B. W. Sui, J. X. Gou, L. K. Meng, F. Sun, X. J. Wang, N. Qi, Y. Zhang, H. B. He and X. Tang, Int. J. Nanomed., 2012, 7, 109 CrossRef CAS PubMed.
- A. Gessner, R. Waicz, A. Lieske, B. Paulke, K. Mader and R. H. Muller, Int. J. Pharm., 2000, 196, 245 CrossRef CAS PubMed.
- J. Molpeceres, M. Chacon, M. Guzman, L. Berges and M. A. Aberturas, Int. J. Pharm., 1999, 18, 101 CrossRef.
- M. A. Radwan, I. Y. Zaghloul and Z. H. Aly, Eur. J. Pharm. Sci., 1999, 8, 95 CrossRef CAS PubMed.
- R. A. Jain, Biomaterials, 2000, 21, 2475 CrossRef CAS PubMed.
- O. Kayser, C. Olbrich, V. Yardley, A. F. Kiderlen and S. L. Croft, Int. J. Pharm., 2003, 254, 73 CrossRef CAS PubMed.
- B. E. Rabinow, Nat. Rev. Drug Discovery, 2004, 3, 785 CrossRef CAS PubMed.
- A. Sabnis, A. S. Wadajkar, P. Aswath and K. T. Nguyen, Nanomedicine, 2009, 15, 305 Search PubMed.
- M. Mastrogiacomo, A. Papadimitropoulos, A. Cedola, F. Peyrin, P. Giannoni, S. G. Pearce, M. Alini, C. Giannini, A. Guagliardi and R. Cancedda, Biomaterials, 2007, 28, 1376 CrossRef CAS PubMed.
- N. Sahai and M. Anseau, Biomaterials, 2005, 26, 5763 CrossRef CAS PubMed.
- G. Chan and D. J. Mooney, Trends Biotechnol., 2008, 26, 382 CrossRef CAS PubMed.
- S. Wang, N. Mamedova, N. A. Kotov, W. Chen and J. Studer, J. Nanosci. Lett., 2002, 2, 817 CrossRef CAS.
- S. Haidar, R. C. Hamdy and M. Tabrizian, Biomaterials, 2008, 29, 1207 CrossRef PubMed.
- N. Nishiyama, Y. Matsumura and K. Kataoka, Cancer Sci., 2016, 107, 867 CrossRef CAS PubMed.
- J. J. Panda, A. Mishra, A. Basu and V. S. Chauhan, Biomacromolecules, 2008, 9, 2244 CrossRef CAS PubMed.
- S. R. Devasani, A. Dev, S. Rathod and G. Deshmukh, Pharmaceut. Bio. Evaluations, 2016, 3, 60 Search PubMed.
- S. K. Shukla, Nidhi, Sudha, Pooja, Namrata, Charu, Akshay, Silvi, Manisha, Rizwana, A. Bharadvaja, G. C. Dubey and A. Tiwari, Adv. Mater. Lett., 2013, 4, 714 CAS.
- R. P. Singh, S. Pal and S. A. Ali, Adv. Mater. Lett., 2014, 5, 24 CAS.
- A. Martien, S. Cohen, W. T. S. Huck, J. Genzer, M. Müller, C. Ober, M. Stamm, G. B. Sukhorukov, I. Szleifer, V. V. Tsukruk, M. Urban, F. Winnik, S. Zauscher, I. Luzinov and S. Minko, Nat. Mater., 2010, 9, 101 CrossRef PubMed.
- Q. Zhao and B. Li, Nanomedicine, 2008, 4, 302 CAS.
- Y. Y. Luo, X. Y. Xiong, Y. Tian, Z.-L. Li, Y. C. Gong and P. Yu, Drug Delivery, 2016, 23(6), 1882–1891 CAS.
- S. Behzadi, et al., Colloids Surf., B, 2104, 123, 143–149 CrossRef PubMed.
- A. Cifuentes-Rius, H. de Puig, J. C. Kah, S. Borros and K. Hamad-Schifferli, ACS Nano, 2013, 7, 10066 CrossRef CAS PubMed.
- G. Baier, D. Baumann, J. M. Siebert, A. Musyanovych, V. Mailaender and K. Landfester, Biomacromolecules, 2012, 13, 2704 CrossRef CAS PubMed.
- B. Kang, P. Okwieka, S. Schçttler, S. Winzen, J. Langhanki, K. Mohr, T. Opatz, V. Mailnder, K. Landfester and F. R. Wurm, Angew. Chem., Int. Ed., 2015, 54, 7436 CrossRef CAS PubMed.
- M. L. Martins, M. J. Saeki, M. T. F. Telling, J. P. R. L. L. Parra, S. Landsgesell, R. I. Smith and H. N. Bordallo, J. Alloys Compd., 2014, 584, 514 CrossRef CAS.
- C. Wiraja, C. D. Yeo, M. S. K. Chong and C. Xu, Small, 2016, 12(10), 1342 CrossRef CAS PubMed.
- Y. Xu, D. W. Piston and C. H. Johnson, Proc. Natl. Acad. Sci. U. S. A., 1999, 96, 151 CrossRef CAS.
- M. K. So, C. Xu, A. M. Loening, S. S. Gambhir and J. Rao, Nat. Biotechnol., 2006, 24, 339 CrossRef CAS PubMed.
- S. Hirsh, Pediatr. Endocrinol. Rev., 2016, 13, 714 Search PubMed.
- J. C. Pickup, Z. L. Zhi, F. Khan, T. Saxl and D. J. Birch, Diabetes/Metab. Res. Rev., 2008, 24, 604 CrossRef CAS PubMed.
- J. Wang, Talanta, 2008, 75, 636 CrossRef CAS PubMed.
- J. Wang and M. Musameh, Analyst, 2003, 128, 1382 RSC.
- R. M. Ramesan and C. P. Sharma, Expert Rev. Med. Devices, 2009, 6, 665 CrossRef CAS PubMed.
- P. Scodeller, V. Flexer, R. Szamocki, E. J. Calvo, N. Tognalli, H. Troiani and A. Fainstein, J. Am. Chem. Soc., 2008, 130, 12690 CrossRef CAS PubMed.
- Y. Li, M. Zhao, J. Chen, S. Fan, J. Liang, L. Ding and S. Chen, Sens. Actuators, B, 2016, 232, 750 CrossRef CAS.
- A. Tiwari and S. Shukla, eXPRESS Polym. Lett., 2009, 3, 553 CrossRef CAS.
- K. Abu-Rabeah and R. S. Marks, Sens. Actuators, B, 2009, 136, 516 CrossRef CAS.
- K.-J. Huang, J. Li, Y.-Y. Wu and Y.-M. Liu, Bioelectrochemistry, 2103, 90, 18 CrossRef PubMed.
- A. Singh, G. Sinsinbar, M. Choudhary, V. Kumar, R. Pasricha, H. N. Verma, S. P. Singh and K. Arora, Sens. Actuators, B, 2013, 185, 675 CrossRef CAS.
- J. Xiaa, X. Caoa, Z. Wanga, M. Yang, F. Zhang, B. Lua, F. Lia, L. Xia, Y. Li and Y. Xia, Sens. Actuators, B, 2016, 225, 305 CrossRef.
- B. Derkus, E. Emregul, K. C. Emregul and C. Yucesanb, Sens. Actuators, B, 2014, 192, 294 CrossRef CAS.
- S. Pandey, G. K. Goswami and K. K. Nanda, Int. J. Biol. Macromol., 2012, 51, 583 CrossRef CAS PubMed.
- K. Khun, Z. H. Ibupoto, J. Lu, M. S. AlSalhi, M. Atif, A. A. Ansari and M. Willander, Sens. Actuators, B, 2012, 173, 698 CrossRef CAS.
- Y. Wang, W. Wei, J. Zeng, X. Liu and X. Zeng, Microchim. Acta, 2008, 160, 253 CrossRef CAS.
- Y.-C. Tsai, S.-Y. Chen and C.-A. Lee, Sens. Actuators, B, 2008, 135, 96 CrossRef CAS.
- S. K. Shukla, O. Parlak, S. K. Shukla, S. Mishra, A. P. F. Turner and A. Tiwari, Ind. Eng. Chem. Res., 2014, 53, 8509 CrossRef CAS.
- H. Dai, Y. Chi, X. Wu, Y. Wang, M. Wei and G. Chen, Biosens. Bioelectron., 2010, 25, 1414 CrossRef CAS PubMed.
- D. Zhuang, F. Wen, Y. Cui, T. Tan and J. Yang, J. Polym. Sci., Part A: Polym. Chem., 2016, 54, 1929 CrossRef CAS.
- J. W. Lim and I. J. Kang, Bull. Korean Chem. Soc., 2014, 35, 25 CrossRef CAS.
- E. Emregul, O. Kocabay, B. Derkus, T. Yumak, K. C. Emregul, A. Sınag and K. Polat, Bioelectrochemistry, 2013, 90, 8 CrossRef CAS PubMed.
- X. Chen, Theranostics, 2011, 1, 1 CrossRef PubMed.
- M. J. Hajipour, S. Laurent, A. Aghaie, F. Rezaee and M. Mahmoudi, Biomater. Sci., 2014, 2, 1210–1221 RSC; M. J. Hajipour, J. Raheb, O. Akhavan, S. Arjmand, O. Mashinchian, M. Rahman, M. Abdolahad, V. Serpooshan, S. Laurent and M. Mahmoudi, Nanoscale, 2015, 7, 8978 RSC.
- T. H. Kim, S. Lee and X. Chen, Expert Rev. Mol. Diagn., 2013, 13, 257 CrossRef CAS PubMed.
- K. Y. Choi, E. J. Jeon and H. Y. Yoon, et al., Biomaterials, 2012, 33, 6186 CrossRef CAS PubMed.
- K. Y. Choi, E. J. Jeon and H. Y. Yoon, et al., Biomaterials, 2012, 33, 6186 CrossRef CAS PubMed.
- M. S. Muthu, D. T. Leong, L. Mei and S. S. Feng, Theranostics, 2014, 4, 660 CrossRef CAS PubMed.
- Y. E. Lukianova-Hleb and O. D. Lapotko, Theranostics, 2014, 4, 761 CrossRef PubMed.
- J. Agulla, D. Brea, F. Campos, T. Sobrino, B. Argibay, W. Al-Soufi, M. Blancol, J. Castillo and P. Ramos-Cabrer, Theranostics, 2014, 4, 90 CrossRef CAS PubMed.
- A. Topete, M. Alatorre-Meda, P. Iglesias, E. M. Villar-Alvarez, S. Barbosa, J. A. Costoya, P. Taboada and V. Mosquera, ACS Nano, 2014, 8, 2725 CrossRef CAS PubMed.
- M. K. So, C. Xu, A. M. Loening, S. S. Gambhir and J. Rao, Nat. Biotechnol., 2006, 24, 339 CrossRef CAS PubMed.
- J. R. Lai, Y. W. Chang, H. C. Yen, N. Y. Yuan, M. Y. Liao and C. Y. Hsu, J. Appl. Phys., 2010, 107, 09B318 CrossRef.
- K. Hu, J. Li, Y. Shen, W. Lu, X. Gao, Q. Zhang and X. Jiang, J. Controlled Release, 2009, 134, 55 CrossRef CAS PubMed.
- N. Ahmed, H. Fessi and A. Elaissari, Drug Discovery Today, 2012, 17, 928 CrossRef CAS PubMed.
- J. F. Lovell, C. S. Jin, E. Huynh, H. Jin, C. Kim, J. L. Rubinstein, W. C. Chan, W. Cao, L. V. Wang and G. Zheng, Nat. Mater., 2011, 10, 324 CrossRef CAS PubMed.
- J. A. K. Ekberg and J. A. Stjohn, Anat. Rec., 2014, 297, 121 CrossRef PubMed.
- S. Ratima, A. Jia, W. Y. Yeong and C. K. Chua, Macromol. Mater. Eng., 2015, 3, 858 Search PubMed.
- M. Antonella, T. S. Yi, K. K. Lynn, Y. Wang, G. Yike, G. David, P. Mariangela, B. Nitsa, L. Ann and D. R. Wilson, ACS Nano, 2015, 9, 1236 CrossRef PubMed.
- D. S. Kohane and R. Langer, Pediatr. Res., 2008, 63, 487 CrossRef CAS PubMed.
- V. Marx, Nature, 2015, 522, 373 CrossRef CAS PubMed.
- A. Khademhosseini, J. P. Vacanti and R. Langer, Sci. Am., 2009, 300, 64 CrossRef CAS PubMed.
- M. Goldberg, R. Langer and X. J. Jia, Biomater. Sci., 2007, 18, 241 CrossRef CAS.
- A. Simchi, E. Tamjid, F. Pishbin, A. R. Boccaccini, A. Simchi, E. Tamjid, F. Pishbin and A. R. Boccaccini, Nanomedicine, 2011, 17, 22 Search PubMed.
- N. Dilbaghi, H. Kaur, R. Kumar, P. Arora and S. Kumar, Adv. Mater. Lett., 2013, 4, 175 Search PubMed.
- L. E. Freed, V. N. Gordana, R. J. Biron, D. B. Eagles, D. C. Lesnoy, S. K. Barlow and R. G. Langer, Nat. Biotechnol., 1994, 12, 689 CrossRef CAS.
- C. Sharma, S. Gautam, A. K. Dinda and N. C. Mishra, Adv. Mater. Lett., 2011, 2, 90 CrossRef CAS.
- C. E. Misch and J. B. Suzuki, Tooth extraction, socket grafting, and barrier membrane bone regeneration, in Contemporary Implant Dentistry, Mosby, St Louis, MO, 3rd edn, 2007, pp. 870–904 Search PubMed.
- C. Shi, Y. Zhu, X. Ran, M. Wang, Y. Su and T. Cheng, J. Surg. Res., 2006, 133, 185 CrossRef CAS PubMed.
- A. Gutowska, B. Jeong and M. Jasionowski, Anat. Rec., 2001, 263, 342 CrossRef CAS PubMed.
- M. Zilberman and J. J. Elsner, J. Controlled Release, 2008, 130, 202 CrossRef CAS PubMed.
- H. C. Flemming and J. Wingender, Nat. Rev. Microbiol., 2010, 8, 623 CAS.
- I. O. Smith, X. H. Liu, L. A. Smith and P. X. Ma, Nanomedicine and Nanobiotechnology, 2009, 1, 226 CrossRef CAS PubMed.
- H. C. Wu, T. W. Wang, J. S. Sun, Y. H. Lee, M. H. Shen, Z. R. Tsai, C. Y. Chen and H. C. Hsu, Materials, 2016, 9, 198 CrossRef.
- R. L. Price, M. C. Waid and K. M. Haberstroch, Biomaterials, 2003, 24, 1877 CrossRef CAS PubMed.
- Z. A. Yao and H. G. Wu, Adv. Mater. Lett., 2010, 1, 67 CrossRef CAS.
- C. J. Chung, H. I. Lin, C. M. Chou, P. Y. Hsieh, C. H. Hsiao and Z. Y. Shi, Surf. Coat. Technol., 2009, 203, 1081 CrossRef CAS.
- X. Fenglan, L. Yubao, Y. Xiaoming, L. Hongbing and Z. Li, J. Mater. Sci.: Mater. Med., 2007, 18, 635 CrossRef PubMed.
- D. Shukla and B. I. Devi, J. Neurosci. Rural Pract., 2010, 1, 82 CrossRef PubMed.
- W. Shi, D. Nie, G. Jin, W. Chen, L. Xia, X. Wu, X. Su, X. Xu, L. Ni, X. Zhang, X. Zhang and J. Chen, Biomaterials, 2012, 33, 3119 CrossRef CAS PubMed.
- S. F. Badylak, D. Taylor and K. Uygun, Annu. Rev. Biomed. Eng., 2011, 13, 27 CrossRef CAS PubMed.
- V. N. Gordana, K. O. Lui, N. Tandon and K. R. Chien, Annu. Rev. Biomed. Eng., 2011, 13, 245 CrossRef PubMed.
- M. S. Muthu, D. T. Leong, L. Mei and S. S. Feng, Theranostics, 2014, 4, 660 CrossRef CAS PubMed.
- E. Mastrobattista, M. A. Vander Aa, W. E. Hennink and D. J. A. Crommelin, Nat. Rev., 2006, 5, 115 Search PubMed.
- U.S. Food and Drug Administration, Cosmetics Q&A: FDA's Authority, http://www.fda.gov/Cosmetics/ResourcesForYou/Consumers/Cosmetics%20QA/ucm%20135709.htm.
- M. H. Fulekar, Nanotechnology: Importance and Application, IK International Publishing House, New Delhi, India, 2010 Search PubMed.
- I. M. Martins, M. F. Barreiro, M. Coelho and A. E. Rodrigues, Microencapsulation of essential oils with biodegradable polymeric carriers for cosmetic applications, Chem. Eng. J, 2014, 245, 191–200 CrossRef CAS.
- RC Scientific and Technical Report, Nanomedicine: Drivers for development and possible Impacts. JRC European Commission 2008.
- D. D. Lasic, Applications of Liposomes, Handbook of Biological Physics, ed. R. Lipowsky and E. Sackmann, Elsevier, Amsterdam, 1995, vol. 1 Search PubMed.
- R. H. Müller, E. B. Souto and S. Gohla, SOFW Journal, 2004, 9, 36 Search PubMed.
- A. Ammala, Int. J. Cosmet. Sci., 2013, 35, 113 CrossRef CAS PubMed.
- R. Rautela and S. S. Cameotra, Environment and Sustainable Development, ed. M. H. Fulekar, B. Pathak and R. K. Kale, Springer, India, 2014 Search PubMed.
- R. H. Müller, R. H. M. Radtke and S. A. Wissing, Adv. Drug Delivery Rev., 2002, 54, S131 CrossRef.
- E. Gilbert, F. Pirot, V. Bertholle, L. Roussel, F. Falson and K. Padois, Int. J. Cosmet. Sci., 2013, 35, 208 CrossRef CAS PubMed.
- M. Ramalingam and A. Tiwari, Adv. Mater. Lett., 2010, 1, 179 CrossRef.
- P. K. Bowen, R. E. Shearier, S. Zhao, R. J. Guillory, F. Zhao, J. Goldman and W. Jaroslaw, Adv. Healthcare Mater., 2016, 5, 1121 CrossRef CAS PubMed.
- Y. Qazi, G. Wong, B. Monson, J. Stringham and B. K. Ambati, Brain Res. Bull., 2010, 81, 198 CrossRef PubMed.
- I. Roy, T. Y. Ohulchanskyy, H. F. Pudavar, B. J. Bergey, A. R. Oseroff, J. Morgan, T. J. Dougherty and P. N. Prasad, J. Am. Chem. Soc., 2003, 125, 7860 CrossRef CAS PubMed.
- S. Doppalapudi, A. Jain, J. A. Domb and W. Khan, Expert Opin. Drug Delivery, 2016, 13, 891 CAS.
- M. Ramalingam and A. Tiwari, Adv. Mater. Lett., 2010, 1, 179 CrossRef.
- Y. Qazi, G. Wong, B. Monson, J. Stringham and B. K. Ambati, Brain Res. Bull., 2010, 81, 198 CrossRef PubMed.
- R. Duncan and M. J. Vicent, Adv. Drug Delivery Rev., 2013, 65, 60 CrossRef CAS PubMed.
- J. Khandare and T. Minko, Prog. Polym. Sci., 2006, 31, 359 CrossRef CAS.
- J. M. Ellingford, J. Med. Genet., 2016, 1 Search PubMed.
- L. Zhang, F. X. Gu, J. M. Chan, A. Z. Wang, R. S. Langer and O. C. Farokhzad, Clin. Pharmacol. Ther., 2008, 83, 761 CrossRef CAS PubMed.
- I. Armentano, M. Dottori, E. Fortunati, S. Mattioli and J. M. Kenny, Polym. Degrad. Stab., 2010, 95, 2126 CrossRef CAS.
- S. Prasad, V. Cody, J. K. Saucier-Sawyer, W. M. Saltzman, C. T. Sasaki, R. L. Edelson, M. A. Birchall and D. J. Hanlon, Nanomedicine, 2011, 7, 1 CAS.
- S. S. Banerjee and D. H. Chen, Chem. Mater., 2007, 19, 6345 CrossRef CAS.
- T. K. Jain, M. K. Reddy, M. A. Morales and D. L. Leslie-Pelecky, Mol. Pharm., 2008, 5, 316 CrossRef CAS PubMed.
- J. J. Lai, J. M. Hoffman, M. Ebara, A. S. Hoffman, C. Estournès and A. Wattiaux, Langmuir, 2007, 23, 7385 CrossRef CAS PubMed.
- S. H. Hu, T. Y. Liu, D. M. Liu and S. Y. Chen, J. Controlled Release, 2007, 121, 181 CrossRef CAS PubMed.
- T. Y. Liu, S. H. Hu, K. H. Liu, D. M. Liu and S. Y. Chen, J. Controlled Release, 2008, 126, 228 CrossRef CAS PubMed.
- M. Rahimi, Y. M. Cheng, E. I. Meletis, R. C. Eberhart and K. Nguyen, J. Nanosci. Nanotechnol., 2009, 9, 4128 CrossRef CAS PubMed.
- M. Rahimi, S. Kilaru, S. Hajj, E. L. Ghida, A. Saleh and D. Rudkevich, J. Biomed. Nanotechnol., 2008, 4, 1 Search PubMed.
- S. De Felice, The nutraceutical revolution: fueling a powerful, new international market, 1989, http://www.fimdefelice.org, http://www.anajana.org Search PubMed.
- D. Santos, P. Pereira, S. H. Flores, R. O. Alessandro and R. C. Chiste, Trends Food Sci. Technol., 2016, 53, 23 CrossRef.
- J. Bernal, J. A. Mendiola, E. Ibáñez and A. Cifuentes, J. Pharm. Biomed. Anal., 2011, 55, 758 CrossRef CAS PubMed.
- X. Yang, H. Tian, C. T. Ho and Q. Huang, J. Agric. Food Chem., 2011, 59, 6113 CrossRef CAS PubMed.
- M. Fathi, M. R. Mozafari and M. Mohebbi, Trends Food Sci. Technol., 2012, 23, 13 CrossRef CAS.
- Z. Du, C. Wang, X. Tai, G. Wang and X. Liu, ACS Sustainable Chem. Eng., 2016, 4, 983 CrossRef CAS.
- H. Fukui, T. Koike, A. Saheki, S. Sonoke and J. Seki, Int. J. Pharm., 2003, 265, 37 CrossRef CAS PubMed.
- Y. Guo, X. Liu, X. Sun, Q. Zhang, T. Gong and Z. Zhang, Theranostics, 2012, 2, 1104 CrossRef CAS PubMed.
- V. N. Kilin, H. Anton, N. Anton, E. Steed, J. Vermot, T. F. Vandamme, Y. Mely and A. S. Klymchenko, Biomaterials, 2014, 35, 4950 CrossRef CAS PubMed.
- L. Salvia-Trujillo, C. Qian, O. Martín-Belloso and D. J. McClements, Food Chem., 2013, 141, 1472 CrossRef CAS PubMed.
- N. Anton, M. Atzenhoffer, F. Daubeuf, X. Li, V. B. Schini-Kerth, B. Delmotte, T. F. Vandamme and T. Chataigneau, Int. J. Pharm., 2013, 454, 43 CrossRef PubMed.
- B. Clares, A. C. Calpena, A. Parra, G. Abrego, H. Alvarado, J. F. Fangueiro and E. B. Souto, Int. J. Pharm., 2014, 473, 591 CrossRef CAS PubMed.
- L. Alaadin, M. A. Nehad, M. Abhita, S. P. Sylvester and N. Sami, J. Nanosci. Nanotechnol., 2014, 14, 4002 CrossRef.
- Y. Zhang and J. Zhang, J. Colloid Interface Sci., 2005, 283, 352 CrossRef CAS PubMed.
- X. Yang, H. Tian, C.-T. Ho and Q. Huang, J. Agric. Food Chem., 2012, 60, 402 CrossRef CAS PubMed.
- E. Rostami, S. Kashanian, A. H. Azandaryani, H. Faramarzi, J. Ezzati, N. Dolatabadi and K. Omidfar, Chem. Phys. Lipids, 2014, 181, 56 CrossRef CAS PubMed.
- D. A. Camfield, J. Sarris and M. Berk, Prog. Neuro-Psychopharmacol. Biol. Psychiatry, 2011, 35, 887 CrossRef PubMed.
- P. Kuppusamya, M. M. Yusoffa, G. P. Maniama, S. J. AriefIchwanb, I. Soundharrajanc and N. Govindan, Acta Pharm. Sin. B, 2014, 4, 173 CrossRef PubMed.
- G. Finch, H. Havel, M. Analoui, R. W. Barton, A. R. Diwan, M. Hennessy, V. Reddy, N. Sadrieh, L. Tamarkin, M. Wolfgang, B. Yerxa, B. Zolnik and M. Liu, AAPS J., 2014, 16, 698 CrossRef CAS PubMed.
- A. Hafner, J. Lovric, G. P. Lakos and I. Pepic, Int. J. Nanomed., 2014, 9, 1005 Search PubMed.
- W. C. Zamboni, V. Torchilin, A. Patri, J. Hrkach, S. Stern, R. Lee, A. Nel, N. J. Panaro and P. Grodzinski, Clin. Cancer Res., 2012, 18, 3229 CrossRef CAS PubMed.
- I. S. Vizirianakis, Nanomedicine, 2011, 7, 11 CAS.
- Y. Liu, M. Solomon and S. Achilefu, Med. Res. Rev., 2013, 33, 3 CrossRef CAS PubMed.
- V. Raffa, O. Vittorio, C. Riggio and A. Cuschieri, Minim. Invasive Ther. Allied Tech., 2010, 19, 127 CrossRef CAS PubMed.
- R. Duncan and M. J. Vicent, Adv. Drug Delivery Rev., 2013, 65, 60 CrossRef CAS PubMed.
- A. N. Gordon, J. T. Fleagle, D. Guthrie, D. E. Parkin, M. E. Gore and A. J. Lacave, J. Clin. Oncol., 2001, 19, 3312 CAS.
- FDA approves Dauno Xome as first-line therapy for Kaposi's sarcoma. Food and Drug Administration, J. Int. Assoc. Physicians AIDS Care, 1996, 2(5), 50–51.
- P. A. Dinndorf, J. Gootenberg, M. H. Cohen, P. Keegan and R. Pazdur, Oncologist, 2007, 12, 991 CrossRef CAS PubMed.
- C. Oerlemans, W. Bult, M. Bos, G. Storm, J. F. Nijsen and W. E. Hennink, Pharm. Res., 2010, 27, 2569 CrossRef CAS PubMed.
- H. Maeda, G. Y. Bharate and J. Daruwalla, Eur. J. Pharm. Biopharm., 2009, 71, 409 CrossRef CAS PubMed.
- A. Kumari, S. K. Yadav and S. C. Yadav, Colloids Surf., B, 2010, 75, 1 CrossRef CAS PubMed.
- H. Zhu, H. Chen, Z. Zeng, Z. Wang, Z. Zhang, Y. Wu, Y. Gao, J. Zhang, K. Liu, R. Liu, L. Cai, L. Mei and S. S. Feng, Biomaterials, 2014, 35, 2391 CrossRef CAS PubMed.
- L. Zhang, J. M. Chan, F. X. Gu, J. W. Rhee, A. Z. Wang, A. F. Radovic- Moreno, F. Alexis, R. Langer and O. C. Farokhzad, ACS Nano, 2008, 8, 1696 CrossRef PubMed.
- T. Lammers, W. E. Hennink and G. Storm, Br. J. Cancer, 2008, 99, 392 CrossRef CAS PubMed.
- A. Bathool, G. D. Vishakante, M. S. Khan and H. G. Shivakumar, Adv. Mater. Lett., 2012, 3, 466 CAS.
- V. Wagner, A. Dullaart, A. K. Bock and A. Zweck, Nat. Biotechnol., 2006, 24, 1211 CrossRef CAS PubMed.
- Integrated Biomaterials for Biomedical Technology, ed. M. Ramalingam, A. Tiwari, S. Ramakrishna and H. Kobayashi, Wiley-Scrivener, USA, 2012 Search PubMed.
- K. Riehemann, S. W. Schneider, T. A. Luger, B. Godin, M. O. Ferrari and H. Fuchs, Angew. Chem., Int. Ed., 2009, 48, 872 CrossRef CAS PubMed.
- M. Powell, M. Griffin and S. Tai, Environ. Manage., 2008, 42, 426 CrossRef PubMed.
- D. G. Rickerby, J. Nanosci. Nanotechnol., 2007, 7, 4618 CAS.
- J. S. Tsuji, A. D. Maynard, P. C. Howard, J. T. James, C. W. Lam, D. B. Warheit and A. B. Santamaria, Toxicol. Sci., 2006, 89, 42 CrossRef CAS PubMed.
- V. L. Colvin, Nat. Biotechnol., 2003, 21, 1166 CrossRef CAS PubMed.
- H. Wang, N. Eliaz, Z. Xiang, H. P. Hsu, M. Spector and L. W. Hobbs, Biomaterials, 2006, 27, 4192 CrossRef CAS PubMed.
- T. Furuzono, M. Masuda, M. Okada, S. Yasuda, H. Kadono, R. Tanaka and K. Miyatake, ASAIO J., 2006, 52, 315 CrossRef CAS PubMed.
- F. D. Gilliland, Y. F. Li, A. Saxon and D. Diaz-Sanchez, Lancet, 2004, 363, 119 CrossRef CAS.
- A. Nel, T. Xia, L. Madler and N. Li, Science, 2006, 311, 622 CrossRef CAS PubMed.
- A. A. Shvedova, E. R. Kisin and R. Mercer, et al., Am. J. Physiol.: Lung Cell. Mol. Physiol., 2005, 289, L698 CrossRef CAS PubMed.
- J. B. Mangum, E. A. Turpin, A. Antao-Menezes, M. F. Cesta, E. Bermudez and J. C. Bonner, Part. Fibre Toxicol., 2006, 3, 15 CrossRef PubMed.
- K. Pulskamp, S. Diabat and H. F. Krug, Toxicol. Lett., 2007, 168, 58 CrossRef CAS PubMed.
- N. Lewinski, V. Colvin and R. Drezek, Small, 2008, 4, 26 CrossRef CAS PubMed.
- P. Jani, G. W. Halbert, J. Langridge and A. T. Florence, J. Pharm. Pharmacol., 1990, 42, 821 CrossRef CAS PubMed.
- Y. Zhang, Z. Hu, M. Ye, Y. Pan, J. Chen, Y. Luo, Y. Zhang, L. He and J. Wang, Eur. J. Pharm. Biopharm., 2007, 66, 268 CrossRef CAS PubMed.
- M. E. Akerman, W. C. Chan, P. Laakkonen, S. N. Bhatia and E. Ruoslahti, Proc. Natl. Acad. Sci. U. S. A., 2002, 99, 12617 CrossRef CAS PubMed.
- S. Chong, K. S. Lee, M. J. Chung, J. Han, O. J. Kwon and T. S. Kim, Radiographics, 2006, 26, 59 CrossRef PubMed.
- P. H. Hoet, I. Bruske-Hohlfeld and O. V. Salata, J. Nanobiotechnol., 2004, 2, 12 CrossRef PubMed.
- A. K. Gupta and M. Gupta, Biomaterials, 2005, 26, 3995 CrossRef CAS PubMed.
- H. Benyamini, A. Shulman-Peleg, H. J. Wolfson, B. Belgorodsky, L. Fadeev and M. Gozin, Bioconjugate Chem., 2006, 17, 378 CrossRef CAS PubMed.
- S. Gurunathan and J. H. Kim, Int. J. Nanomed., 2016, 11, 1927 CrossRef PubMed.
- J. Wegener, D. Abrams, W. Willenbrink, H. J. Galla and A. Janshoff, Biotechniques, 2004, 37, 590 CAS.
- J. Wegener, D. Abrams, W. Willenbrink, H. J. Galla and A. Janshoff, Biotechniques, 2004, 37, 392 Search PubMed.
- J. Wegener, D. Abrams, W. Willenbrink, H. J. Galla and A. Janshoff, BioTechniques, 2004, 37, 348 Search PubMed.
- M. S. Bynoe, C. Viret, A. Yan and D. G. Kim, Fluids Barriers CNS, 2015, 12, 20 CrossRef PubMed.
- D. Hoheisel, T. Nitz, H. Franke, J. Wegener, A. Hakvoort, T. Tilling and H. J. Galla, Biochem. Biophys. Res. Commun., 1998, 244, 312 CrossRef CAS PubMed.
- K. Furusawa, T. Mizutani and N. Sasaki, Regenerative Therapy, 2016, 3, 82–89 CrossRef.
|
This journal is © The Royal Society of Chemistry 2016 |