DOI:
10.1039/C6RA15373A
(Paper)
RSC Adv., 2016,
6, 76360-76369
Low generation anionic dendrimers modulate islet amyloid polypeptide self-assembly and inhibit pancreatic β-cell toxicity†
Received
13th June 2016
, Accepted 24th July 2016
First published on 5th August 2016
Abstract
The deposition of the islet amyloid polypeptide (IAPP) as insoluble amyloid fibrils in the pancreatic islets is associated with type II diabetes. Recent studies have revealed that pre-fibrillar proteospecies and/or the amyloidogenic process mediate β-cell degeneration whereas amyloid fibrils are poorly cytotoxic. Thus, therapeutic strategies that aim at preventing β-cell death associated with amyloid deposition should either sequester prefibrillar species and/or modulate the initial steps of fibrillization. In this view, low generation flexible dendritic scaffolds harboring 4 to 16 hydroxyl, amine, carboxylate or sulfate functional groups were designed and evaluated for their effects on IAPP self-assembly and cytotoxicity. Whereas neutral polyhydroxylated and polycationic dendrimers did not affect the kinetics of amyloid assembly, carboxylated dendrimers accelerated IAPP fibrillization proportionally to surface group density. Interestingly, as revealed by thioflavin T fluorescence, circular dichroism spectroscopy and atomic force microscopy, the G0 sulfated dendrimer inhibited amyloid formation by maintaining the peptide in a random coil conformation. In contrast, G1 sulfated dendrimers potentiated IAPP self-assembly into long amyloid fibrils by a scaffold-based mechanism. Anionic dendrimers attenuated IAPP-induced toxicity on pancreatic β-cells. Our results indicate that sulfated dendrimers can alter the fibrillization pathway of IAPP and inhibit its proteotoxicity, either by accelerating amyloid formation or by trapping the peptide in a non-aggregating and non-toxic state. This study offers novel mechanistic insights for the design of a nanomolecular scaffold to manipulate the self-assembly of natively disordered amyloidogenic peptides.
1 Introduction
Numerous human diseases are associated with the deposition and accumulation of insoluble protein aggregates within the extracellular space, including Alzheimer's disease and several amyloidoses.1 Protein aggregation is a consequence of the self-assembly of a polypeptide, often into a quaternary structure rich in cross-β-sheets, known as amyloids fibrils. Over 30 endogenous proteins have been identified as precursors of amyloids whose depositions are linked to cellular degeneration.2 Owing to the causative link between amyloid deposition and the pathological states, the identification of molecules that can interfere with the amyloidogenic process is an area of extensive research.1 Therapeutic strategies to arrest protein aggregation include; (i) stabilization of the protein in its native state, (ii) destabilization of amyloid cross-β-sheets quaternary structure and (iii) inhibition of oligomerization.3 For instance, stabilization of transthyretin (TTR) quaternary native state led to the discovery of the first molecule used in the clinics to treat an amyloid disease.4 However, this rational approach is challenging to apply to intrinsically disordered polypeptides (IDPs), such as the β-amyloid peptide (Aβ), the islet amyloid polypeptide (IAPP) and α-synuclein, as the amyloidogenic precursors exhibit a wide conformational ensemble.5 Nonetheless, molecules harboring several hydrophilic and/or charged chemical groups at their surface have been reported to modulate IDP self-assembly. For example, the green tea polyphenol (−)-epigallocatechin gallate (EGCG), which is known to inhibit the aggregation of Aβ,6 α-synuclein,7 IAPP8 and the tau protein,9 exhibits a high density of hydroxyl groups. Besides, sulfated glycosaminoglycans (GAGs) are known to accelerate fibril formation and to inhibit the toxicity of several amyloidogenic IDPs, including Aβ10 and IAPP.11,12 Nonetheless, the relationships between the nature and density of hydrophilic functional groups with the effects on IDPs self-assembly have been poorly addressed so far.
Over the last decade, a few studies have reported that dendrimers can modulate amyloid formation from amyloidogenic IDPs, such as Aβ-peptide derivatives, prion protein fragments and IAPP, by either accelerating or preventing self-assembly.13,14 Dendrimers consist of globular polymers built on a central core scaffold surrounded by layers of branched monomers.15 These scaffolds, which represent a unique class of nanostructures that can be designed with various sizes, shapes and surface chemistries, have recently received attention as potential anti-amyloidogenic agents.13,16 For instance, cationic poly(propyleneimine) dendrimers decorated with maltose were shown to convert toxic Aβ species into nonfibrillar aggregates and to reduce amyloid cytotoxicity on PC12 and SH-SY5Y cells.17 Similarly, gallic acid–triethylene glycol dendrimers decorated with morpholine groups have been shown to reduce the toxicity of Aβ peptide fragment by accelerating the conversion of cytotoxic oligomeric species into fibrils.18 Particularly, owing to their flexible nature and to the high density of chemical functionalities that can be attached to the dendritic core, these nanostructures are perfectly suited to target natively unfolded polypeptides.
IAPP is a 37-residue glucomodulatory hormone (Fig. 1A) that is co-expressed and co-secreted with insulin by the pancreatic β-cells.19 In patients afflicted with type-2 diabetes (DM-2), this unusual aggregation-prone peptide forms amyloids that deposit in the pancreas, leading to the degeneration of the islets of Langerhans.19 IAPP amyloidogenesis observed in the pancreas is known to exacerbate pancreatic β-cells death; accelerating the progression of the physiopathology. When applied to isolated pancreatic cells in culture, non-fibrillar IAPP induces cell death,20–22 although clear mechanistic details remain elusive. Interestingly, amyloid fibrils show low toxicity and as for other amyloidogenic polypeptides, oligomeric species are considered as the most proteotoxic species of the self-assembly cascade.11,23 There is currently no therapeutic strategy to prevent amyloid deposition observed in pancreatic islets of diabetic patients. Thus, the discovery of compounds that modulate IAPP amyloidogenesis and prevent β-pancreatic cells death represents an important research goal. However, targeting an IDP amyloidogenic peptide that exhibits a wide conformational ensemble, such as IAPP, is challenging and requires the design of novel and flexible molecular scaffolds.
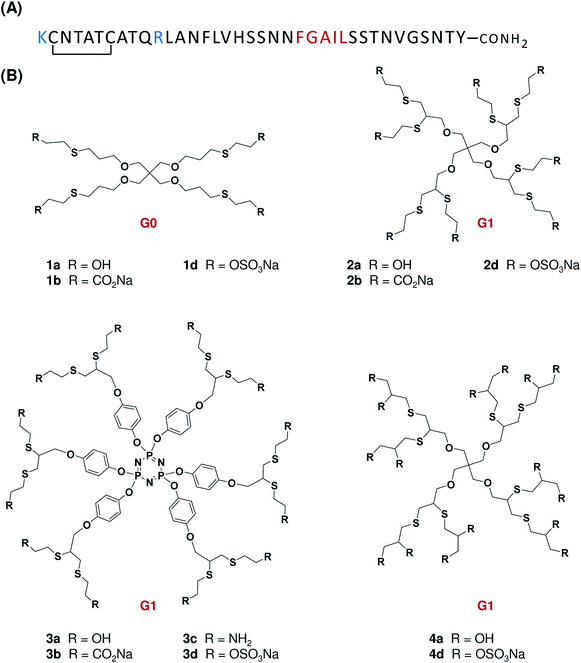 |
| Fig. 1 (A) Primary structure of human IAPP. Positively charged residues are indicated in blue whereas the amyloidogenic domain is represented in red. IAPP contains a disulfide bond between Cys-2 and Cys-7 and a C-α-amidated C-terminus. (B) Chemical structures of surface low generation dendrimers used in the present study. The generation level (G) is indicated in red. | |
Whereas the effects of large neutral or cationic dendrimers on IDPs self-assembly have been recently reported, nothing is known about how low generation anionic dendrimers can modulate fibrillization of amyloidogenic IDPs. Moreover, although derivatives densely functionalized with hydrophilic and/or charged groups are known to modulate the amyloidogenic process, mechanistic insights about the relationship between the chemical nature, orientation and density of functional units are still scarce. In this context, we investigated the structure–activity relationships (SAR) of low generation polyhydroxylated, polyaminated, polycarboxylated and polysulfated dendrimers harboring 4 to 16 peripheral groups. The present study shows a unique effect of sulfated dendrimers on amyloidogenesis that reside in the charge density and indicates that IAPP-induced cytotoxicity can be efficiently inhibited with anionic dendrimers. Our results offer key indications regarding the nature of the proteotoxic species and expose novel mechanistic insights for the design of molecular identities to manipulate the self-assembly of amyloidogenic IDPs.
2 Materials and methods
2.1 Dendrimers synthesis, purification and characterization
Synthetic routes towards low generation flexible dendrimer scaffolds and approaches for their purification and characterization are described in details in the ESI.†
2.2 Peptide synthesis and IAPP sample preparation
IAPP was synthesised by solid phase peptide synthesis and purified by preparative reversed-phase high performance liquid chromatography (RP-HPLC). Disulfide bond formation between Cys-2 and Cys-7 was achieved by dimethyl sulfoxide (DMSO) oxidation.24 These methods are described in details in the ESI.† Aliquots of monomerized IAPP were prepared by dissolving the pure lyophilized peptide in 100% hexafluoro-2-propanol (HFIP) to a concentration of 1 mg mL−1. The solution was sonicated for 30 min and filtered through a 0.22 μm hydrophilic polypropylene filter before being lyophilized. Lyophilized IAPP was resolubilized in HFIP at 1 mg mL−1, sonicated for 30 min and the solution was aliquoted and lyophilized to remove HFIP. Samples were kept dried at −80 °C until used.
2.3 Amyloid formation monitored by thioflavin T fluorescence
IAPP solutions were prepared by dissolving monomerized IAPP at a concentration of 25 μM (2× of final concentration) in 20 mM Tris, pH 7.4, containing 40 μM ThT immediately before final dilution and measurement. Dendrimers were solubilized in either 20 mM Tris buffer (polycarboxylate, polysulfate and polyamine) or in MeOH (polyhydroxyl) at 10× and 100×, respectively. For studies with OH-substituted dendrimers, MeOH concentration was kept below 1% (v/v) and control reaction contained the same percentage of MeOH. Assays were performed at 37 °C without stirring in sealed black-wall, clear-bottom 96-well non-binding surface plates (Corning) with a total volume of 100 μL per well. ThT fluorescence was measured every 10 min over the course of 20 h, using an Infinite M1000 fluorescence plate reader (TECAN). The fluorescence, with excitation at 440 nm and emission at 485 nm, was measured from the bottom of the well. For each experiment, control reactions (without IAPP) were carried. Data obtained from triplicate wells were averaged, corrected by subtracting the corresponding control reaction and plotted as fluorescence vs. time. Data of time-dependence of ThT fluorescence were fitted to a sigmoidal growth model where T50 is the time required to reach half of the fluorescence intensity, k is the apparent first-order constant and Ymax and Y0 are, respectively, the maximum and initial fluorescence values:
The lag time, the time before detectable amyloid formation occurs, as predicted by means of the nucleation dependent polymerization model, is described as T50 − 2/k. Data represents the average of at least two independent experiments performed in triplicate.
2.4 Circular dichroism spectroscopy
IAPP was dissolved in 20 mM Tris, pH 7.4 and mixed with an equivalent volume of 2× of dendrimers prepared in the corresponding buffer, and the samples were incorporated into a 2 mm path length quartz cell. Far-UV CD spectra were recorded from 189 to 260 nm using a J-815 CD spectropolarimeter (Jasco) at 25 °C. The wavelength step was set at 0.5 nm with an average time of 10 s per scan at each wavelength step. Each collected spectrum was background subtracted with the corresponding IAPP-free sample. Each spectrum represents the average of at least two independent experiments.
2.5 Atomic force microscopy
AFM was performed with a MultiMode 8 atomic force microscope controlled by Nanoscope V electronics (Bruker, Billerica, MA) equipped with a 100 μm scanner (J-scanner). IAPP (50 μM) was solubilized in absence or in presence of functionalized dendrimers in 20 mM Tris–HCl pH 7.4. The solution was incubated for 20 h at 25 °C before being diluted by a factor of 10 in acetic acid 1%. 20 μL of IAPP sample were deposited onto a freshly cleaved mica and incubated for 2 min at room temperature. The mica was washed twice with deionized water and air-dried for 24 h. AFM images were recorded in tapping mode using silicon probes (Bruker). Line scan frequency was set at 0.2 Hz. For quantification analysis, the width and length of at least 50 individual fibrils per experiment were determined and plotted as a frequency distribution using the Prism 6.0 software. Data (height and length) of at least three individual experiments were averaged and were expressed as the mean ± standard deviation.
2.6 Cell toxicity assay
Rat INS-1 β-pancreatic cells were seeded in black wall clear bottom 96-well plates (TC treated) at a density of 30
000 cells per well (100 μL per well) in RPMI-1640 medium supplemented with 10% FBS, 2 mM L-glutamine, 100 U mL−1 penicillin, 100 μg mL−1 streptomycin, 10 mM HEPES, 1 mM sodium pyruvate, 50 μM β-mercaptoethanol. INS-1 cells were provided by Pr Alain Fournier, INRS-Institut-Armand, Canada. After 48 h incubation at 37 °C in a 5% CO2 incubator, cells were treated by directly adding 50 μL of IAPP solutions at 3× of final concentration in 20 mM Tris, pH 7.4, (with or without dendrimers) that have been pre-incubated, or not, for 16 h at room temperature. The cells were incubated for 24 h and cell viability was measured by the resazurin reduction assay. Cell viability (in % of control) was calculated from the ratio of the fluorescence of the treated sample to the control cells (non-treated). All compounds were individually tested using the above protocol for their cytotoxicity at concentrations of 5 and 50 μM. Data (in %) of at least three individual experiments performed in triplicate were averaged and were expressed as the mean ± S.E.M. Evaluation of the results was made using the Student's t-test and statistical difference (between control and dendrimers-treated or between IAPP-treated and IAPP-treated + dendrimers) was established at P < 0.05. Statistical analysis was performed using Prism 6.0 software.
3 Results and discussion
3.1 Synthesis of low generation dendrimers
To address the effect of dendrimers on IAPP amyloidogenesis and to obtain key SAR information regarding the nature and density of surface groups and the molecular scaffold, a small library of flexible low generation (G0, G1) neutral, cationic and anionic dendrimers were prepared (Fig. 1B). A divergent synthesis was designed for the construction of polyhydroxylated, polyaminated, polycarboxylated and polysulfated dendrimers harboring 4 to 16 peripheral groups. These molecules were readily synthesized using either thiol–ene or thiol–yne reactions around tetravalent and hexavalent core molecules.25–27 Experimental details regarding the synthesis of these compounds are included in the ESI (Fig. S1 to S37†).
3.2 Effect of low generation dendrimers on IAPP amyloid formation
We initially evaluated the effect of low generation functionalized dendrimers on the kinetics of fibril formation by measuring the fluorescence of the amyloid-specific dye thioflavin T (ThT). ThT is a small compound that exhibits a strong increase of its fluorescence quantum yield upon its specific binding to the cross-β-sheet quaternary structure of amyloid fibrils.28 IAPP amyloidogenesis is often described as a nucleation-dependent polymerization process that is characterized by a ThT-negative phase (lag-phase), in which the high-energy nucleus is formed, followed by a thermodynamically favorable elongation phase that is characterized by the rapid growth of the fibrils29 (Fig. 2A). In absence of dendrimers, the sigmoidal time course of IAPP fibrillization (12.5 μM; pH 7.4; quiescent condition; non-binding surface) displayed a lag time of 7.72 h and a T50 of 9.36 h (Table 1). The T50 corresponds to the time required to reach half of the maximum ThT fluorescence whereas the lag time is ascribed to the time before detectable amyloid formation occurs. In presence of 1 eq. of neutral polyhydroxylated dendrimers (compounds 1a to 4a) no significant changes in the rate of amyloid formation and final ThT fluorescence were detected (Table 1; Fig. S38†). This result suggests that the inhibitor effect of polyhydroxylated compounds on IAPP aggregation, such as EGCG8 and glucopyranosylgenistein,30 reside in their polyphenolic nature and/or in the spatial distribution of their hydroxyl groups rather than simply in their high density of hydroxyl groups. Similar results were observed for the cationic dendrimer decorated with 12 amine functions (compound 3c; Table 1; Fig. S39†). As cationic dendrimers are known for their inherent cell toxicity,13 we evaluated only one amine-functionalized dendrimer in this study.
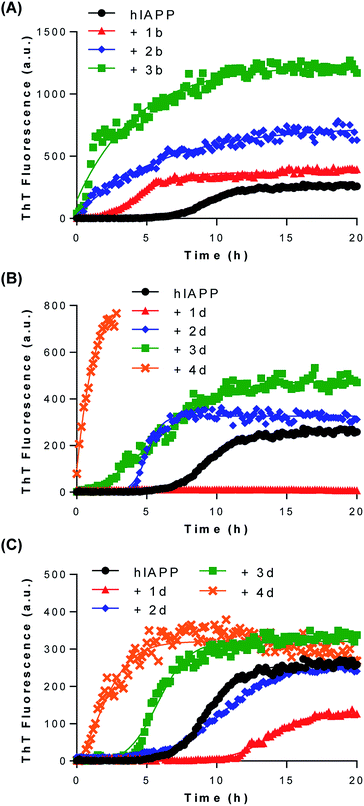 |
| Fig. 2 Effects of low generation dendrimers on IAPP amyloid fibril formation monitored by ThT fluorescence. IAPP (12.5 μM) was incubated in 20 mM Tris, pH 7.4, at 25 °C without agitation in the absence or in the presence of (A) 1 eq. of compounds 1b to 3b, (B) 1 eq. of compounds 1d to 4d or (C) 0.1 eq. of compounds 1d to 4d. (A–C) ThT fluorescence was measured every 10 min over the course of 20 h, with excitation at 440 nm and emission at 485 nm. | |
Table 1 Effects of low generation G0 and G1 dendrimers on IAPP amyloid formation
Compounda |
Rb |
Lag timec (h) |
T50d (h) |
Maximum intensitye (% of IAPP control) |
Concentration of IAPP was 12.5 μM and all compounds were tested at 1 eq. (12.5 μM), otherwise stated. Each data represents the mean from at least two individual experiments performed in triplicate. Number in ( ) corresponds to the number of surface groups attached on the dendrimer. Lag time corresponds to the time before detectable amyloid formation occurs, as predicted by means of the nucleation dependent polymerization model. T50 corresponds to the time required to reach half of the maximum ThT fluorescence. The maximum fluorescence of IAPP in absence of compound was set as 100. |
hIAPP |
— |
7.72 |
9.36 |
100 |
1a |
OH (4) |
7.58 |
9.06 |
109 |
2a |
OH (8) |
8.30 |
10.50 |
112 |
3a |
OH (12) |
6.68 |
7.95 |
102 |
4a |
OH (16) |
7.70 |
8.79 |
103 |
1b |
COOH (4) |
2.83 |
4.22 |
144 |
2b |
COOH (8) |
<1 |
<1 |
287 |
3b |
COOH (12) |
N.D. |
N.D. |
480 |
3c |
NH2 (12) |
8.06 |
9.84 |
1.12 |
1d |
OSO3H (4) |
>20 |
>20 |
N.D. |
2d |
OSO3H (8) |
4.38 |
5.01 |
127 |
3d |
OSO3H (12) |
4.03 |
5.39 |
186 |
4d |
OSO3H (16) |
N.D. |
<0.5 |
>800 |
1d (0.1 eq.) |
OSO3H (4) |
12.18 |
13.77 |
49 |
2d (0.1 eq.) |
OSO3H (8) |
8.78 |
10.4 |
95 |
3d (0.1 eq.) |
OSO3H (12) |
3.98 |
5.78 |
125 |
4d (0.1 eq.) |
OSO3H (16) |
<1 |
1.36 |
126 |
Eprodisate |
SO3H (2) |
8.17 |
9.98 |
102 |
Eprodisate (5 eq.) |
SO3H (2) |
8.08 |
9.61 |
103 |
In contrast, dendrimers functionalized with carboxylate units showed strong accelerating effects on IAPP self-assembly. Incubation of IAPP in presence of 1 eq. of compounds 1b and 2b reduced the lag-phase to 2.83 h and to less than 1 h, respectively (Table 1; Fig. 2A). Dendrimer decorated with 12 carboxylate groups (3b) hastened IAPP fibrillogenesis to such an extent that the lag phase was abolished. As inferred from the observed final ThT fluorescence intensity, polycarboxylated G1 dendrimers (2b, 3b) increased the amount of fibrils formed and/or favor the formation of amyloids with better defined cross-β-sheets structure. We observed a direct correlation between the increase of fibrilization and the number of carboxylic units attached to the dendrimeric core. IAPP is a cationic peptide displaying a net charge of +3 at physiological pH (Fig. 1; N-terminus amine, Lys1 and Arg11), favoring its binding through electrostatic interactions to anionic groups. Accordingly, our results suggest that G1 carboxylated dendrimers potentiate IAPP amyloidogenesis by a scaffold-based mechanism, in which the carboxylate groups interact with the positively charged N-terminal domain of IAPP, increasing the local peptide concentration and favoring self-association of the 20–27 amyloidogenic domain into β-sheet-rich assemblies. This scaffold-based mechanism has been previously proposed for the accelerating effect of sulfated GAGs on the assembly of several amyloidogenic polypeptides.31–33
Astonishingly, the correlation between the density of negatively charged units and the acceleration effect on IAPP fibrillization observed for carboxylated dendrimers was not detected for their sulfated counterparts. In fact, when 1 eq. of G0 sulfated dendrimer (1d) was incubated with IAPP, no ThT positive signal was measured over the course of a 20 h assay (Fig. 2B), suggesting a total inhibition of the formation of cross-β-sheet rich assemblies. Thus, substituting the 4 carboxylate groups on the G0 dendritic scaffold by 4 sulfate units led to a compound (1d) that instead of potentiating amyloid assembly inhibits amyloid formation. As both groups are deprotonated under the conditions of the fibrillization assay (pH 7.4), this discrepancy is not related to the charge state of the G0 dendrimer, but most likely resides in their distinctive solubilization capacity. This result reinforces the notion that the sulfate group constitutes a key molecular identity for the design of amyloid assembly inhibitors.34 Anionic dendrimers decorated with 8, 12 or 16 sulfate units (2d, 3d, 4d, respectively) enhanced amyloid formation in a charge density dependent manner. Nonetheless, these effects were less pronounced compared to their carboxylated equivalents. As the sulfated dendrimers displayed interesting amyloid modulating properties when employed at 1 eq., i.e. acceleration or inhibition of IAPP amyloid formation, we evaluated the effects of compounds 1d to 4d at 0.1 eq. (1.25 μM). When used at 0.1 eq. to IAPP, compound 1d harboring 4 sulfate units still delayed the formation of ThT-positive species with a lag phase of over 12 hours (Fig. 2C). Similarly, the accelerating effects of compounds 2d, 3d and 4d on IAPP amyloid assembly were significantly reduced.
To assess the importance of the clustered nature of sulfated dendrimers, we evaluated the effects of eprodisate (propane-1,3-disulfonate) on IAPP amyloid formation. It has been reported that eprodisate, which was developed and tested into the clinics as an amyloid inhibitor,35 had no effect on proIAPP(1–48) aggregation.36 However, the effect of this compound on IAPP self-assembly has never been described in the literature. We observed that this disulfonated GAGs mimetic had no significant effects on IAPP amyloidogenesis when employed at 1 and 5 eq. (Table 1; Fig. S40†). Considering that 62.5 μM of eprodisate (5 eq.) correspond to a concentration of 125 μM sulfonate units whereas 12.5 μM of compound 1d (1 eq.) correspond to a concentration of 50 μM sulfate groups, this indicates that the inhibitory effect of compound 1d most likely resides in its multivalency rather than in the concentration of anionic groups in solution. Moreover, the high flexibility of the G0 dendrimeric scaffold as well as the distance between sulfated units could also make a key contribution to this anti-assembly effect. Interestingly, the sulfonated triphenyl methane derivative acid fuchsin, harboring 3 sulfonate groups, has been reported to be a potent inhibitor of IAPP fibrillization,34 supporting the key role of this chemical group for the inhibition of amyloid formation.
3.3 Modulation of amyloid assembly by low generation sulfated dendrimers monitored by atomic force microscopy
ThT fluorescence reports the formation of protein aggregates rich in cross-β-sheets quaternary structure and not the formation of amorphous aggregates and/or non-fibrillar species. Moreover, the strong inhibitory effect of compound 1d at 1 eq. on amyloid formation observed by ThT fluorescence could be related to the inhibition of dye binding to the amyloids, rather to a real effect on IAPP amyloidogenesis. For instance, it has been observed that the previously reported inhibitory effect of aspirin and ketoprofen on IAPP assembly resides in the interference with Congo Red binding and that these compounds have no effects on amyloid formation.37 Thus, we validated the results obtained with the ThT kinetics assays by monitoring the fibrillization process by atomic force microscopy (AFM). AFM analysis revealed the formation of amyloid fibrils with an average length of 476.5 ± 192.7 nm and an average diameter of 3.52 ± 1.15 nm when IAPP was incubated in absence of dendrimers for 20 hours (Fig. 3A). This result is consistent with previous studies showing that IAPP forms fibrils of around 300 to 1000 nm length and 3 to 10 nm height, when incubated in aqueous buffer at room temperature without agitation.36,38–40 When IAPP was incubated in presence of 1 eq. compound 1d, no fibrils were found on the mica (Fig. 3B). This data supports the inhibition of amyloid formation reported with the ThT assay. In sharp contrast, the presence of compound 3d in the aggregation mixture led to the massive formation of very long (1143.7 ± 252.7 μm) fibrils with an average height of around 5.85 ± 1.66 nm (Fig. 3C). This result shows that the dendrimer decorated with 12 sulfate units not only accelerated amyloid fibrils formation, but favored the formation of significantly longer fibrils. All controls (buffer alone, compounds 1d and 3d alone) did not show any aggregates after 20 hours incubation under the same conditions. AFM analysis performed with representative sulfated G0 and G1 dendrimers confirmed the results obtained with the ThT fluorescence assays and indicated that compound 1d does not only inhibit cross-β-sheets assembly, but it also prevents amorphous aggregation of IAPP.
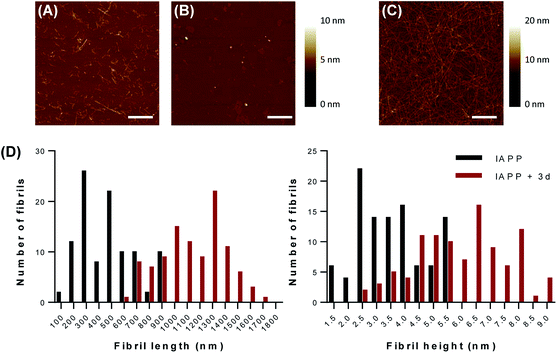 |
| Fig. 3 Low generation sulfated dendrimers modulate IAPP amyloid fibril formation. Representative atomic force microscopy (AFM) images of IAPP incubated for 20 hours in absence (A) or in presence of (B) 1 eq. of compound 1d or (C) 1 eq. of compound 3d. Images are 5 μm × 5 μm and scale bar represents 1 μm. (D) Distribution of height and length of IAPP amyloid fibrils prepared in absence and in presence of 1 eq. of compound 3d. All experiments were performed in 20 mM Tris, pH 7.4, at 25 °C without agitation in presence of 50 μM IAPP. | |
3.4 Modulation of IAPP conformational transition by low generation sulfated dendrimers
In its monomeric soluble structure, IAPP mainly adopts a random coil conformation, although it diverges from a pure disordered peptide by the transient appearance of helical segments.41 Amyloid formation of natively disordered peptide is usually accompanied by a transition of the polypeptide secondary structure from a random coil to a β-sheet-rich conformation, involving different conformational intermediates that could be either on- or off-pathway.40,42 Considering the promising results (ThT assay, AFM) obtained with the polysulfated dendrimers, we evaluated the capacity of these compounds to modulate IAPP conformational transition. The CD spectrum of freshly dissolved IAPP was characteristic of a random coil conformation with a single minimum around 202 nm (Fig. 4A). After 20 h incubation in absence of dendrimers, IAPP exhibited a CD spectrum with a single minimum at around 220 nm, characteristic of a β-sheets-rich secondary structure (Fig. 4C). Strikingly, when IAPP was incubated with the dendritic scaffold functionalized with 4 sulfate groups (1d), this conformational transition was inhibited and IAPP remained in a random coil conformation upon 20 h incubation. As anticipated from AFM analysis and ThT assay, incubation of IAPP in presence of compound 3d accelerated its conformational transition from a random coil to a β-sheet rich structure, supporting the pro-amyloidogenic effect of densely sulfated dendrimers. Moreover, this data shows that although the fibrils obtained without or with compound 3d are morphologically different, as observed by AFM, both amyloid preparations have a secondary structure rich in β-sheets.
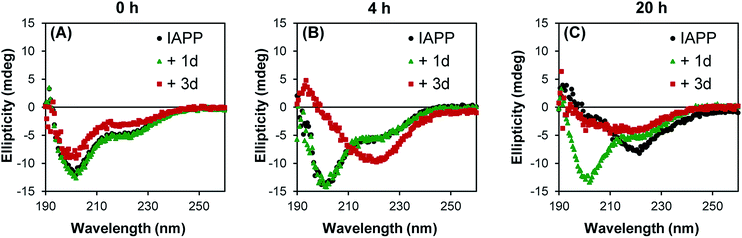 |
| Fig. 4 Conformational transition of IAPP modulated by low generation sulfonated dendrimers. Representative far-UV circular dichroism spectra of 50 μM IAPP incubated in absence (black) or in presence of 1 eq. of compound 1d (green) or 1 eq. of compound 3d (red) at time (A) 0 h, (B) 4 h and (C) 20 h. All experiments were performed in 20 mM Tris, pH 7.4, at 25 °C without agitation. | |
3.5 Anionic low generation dendrimers inhibit IAPP-induced pancreatic β-cells toxicity
All dendritic compounds were initially evaluated for their cytotoxicity towards pancreatic β-cells by means of the resazurin cell viability assay. Among all derivatives, only the cationic dendrimer decorated with amine units (3c), showed potent cytotoxicity at 5 and 50 μM, with cellular viability of 40 and 5% of control, respectively (Fig. 5A). All neutral and anionic G0 and G1 dendrimers did not affect the viability of pancreatic β-cells. Next, compounds were tested for their capacity to inhibit cell death induced by IAPP. It is known that IAPP induces death of pancreatic β-cells, although the exact molecular mechanisms of toxicity are still the matter of active debate.21,22 When cells were treated with freshly dissolved monomerized IAPP, a decrease of cellular viability upon 24 hours treatment was observed in a concentration-dependent manner, with cellular viability of approximatively 40% of non-treated cells at 50 μM (Fig. S41†). This cytotoxic effect is related to the aggregation propensity, as it has been shown that the non-amyloidogenic rodent IAPP is not cytotoxic at concentrations lower than 50 μM.40 We evaluated the toxicity of IAPP proteospecies generated upon 20 h incubation at 25 °C in absence or in presence of 0.1 (5 μM) and 1 eq. (50 μM) of functionalized dendritic scaffolds. As compound 3c was cytotoxic, it was not tested for its ability to protect pancreatic cells against IAPP toxicity. As anticipated from their absence of effects on IAPP amyloidogenesis, neutral polyhydroxylated dendrimers did not reduce, nor increase, the cytotoxicity of 50 μM IAPP on β-pancreatic cells (Fig. 5B). In contrast, polycarboxylated G1 dendrimers attenuated IAPP-induced cell death proportionally to the number of carboxylate units attached to the dendritic core. Actually, the protecting effect of polycarboxylated dendrimers was not dependent to the concentration of carboxylate groups present in solution, but was rather proportional to the density of substitution. For instance, 50 μM compound 1b, i.e. 200 μM of carboxylate groups, did not protect cells against IAPP effect whereas compound 3b at 5 μM, i.e. 60 μM of carboxylate units, totally inhibited the cytotoxic effect of IAPP. This observation is consistent with the results obtained with the ThT assay and suggests that compound 3b is protecting pancreatic cells by accelerating amyloid formation and/or by converting proteotoxic species (monomer, oligomer, and pre-fibrillar) into non-culprit fibrillary aggregates. A similar trend was observed for the polysulfated derivatives (Fig. 5B), whereas compound 3d (12 sulfate units) and 4d (16 sulfate units) increased the viability of IAPP-treated cells. Interestingly, compound 1b at a concentration 50 μM, which showed a clear inhibition of amyloid formation, as measured by ThT-fluorescence, AFM as well as CD spectroscopy, partially inhibited the toxicity of IAPP.
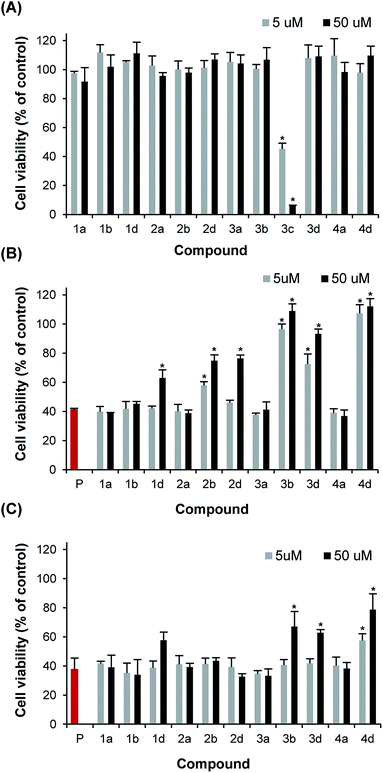 |
| Fig. 5 Inhibition of IAPP-induced cytotoxicity on β-pancreatic cells by low generation dendrimers. (A) Viability of INS-1 cells incubated for 24 h with 5 or 50 μM dendrimers. (B) Viability of INS-1 cells treated for 24 h with IAPP (50 μM; P) that has been pre-incubated for 20 h in absence (red) or in presence of 5 or 50 μM dendrimers. (C) Viability of INS-1 cells treated for 24 h with freshly dissolved IAPP (50 μM) in absence (red) or in presence of 5 or 50 μM dendrimers. * Statistically significant difference between (A) control vs. dendrimer treated or (B, C) IAPP-treated vs. dendrimer IAPP-treated (P < 0.05). | |
Next, we evaluated the effect of these dendritic scaffolds on IAPP-toxicity when they were co-applied with the peptide to the media of pancreatic cells, i.e. without 20 h pre-incubation. Under these conditions, only compounds 3b, 3c and 4b at 50 μM showed a significant reduction of IAPP-induced cell death (Fig. 5C). Taking into account that these compounds drastically accelerated IAPP assembly in vitro, they most likely reduced IAPP toxicity by sequestering IAPP proteospecies and/or by accelerating the amyloidogenic cascade to such an extent that the toxicity is reduced. Compound 1d appeared to protect partially pancreatic cells when added simultaneously to IAPP in the cell media at 50 μM, although this effect was not statistically significant. This result illustrates that a potent inhibitory effect on amyloid formation in a homogenous in vitro environment does not necessarily correlate with a strong capacity of this compound to protect cells against the toxicity of an amyloidogenic polypeptide. Accordingly, it is particularly important that the identification of amyloid modulators in vitro is also performed in milieu that recapitulates, as much as possible, the complex biological environment in which IAPP aggregation and toxicity occur,21 an avenue that we are currently exploring.
4 Conclusion
The self-assembly of the peptidic hormone IAPP in the pancreatic islets is associated with DM-2. While the correlation between amyloid deposition and the severity and progression of DM-2 has been well-established,43 there is currently no therapeutic approaches targeting this amyloidogenic cascade. Over the last two decades, several compounds, including aromatic-rich small molecules,8,30,34,44–46 N-methylated peptide derivatives,47 phosphonated molecular tweezers48 and sulfated glycosaminoglycans,11,12 were shown to modulate IAPP self-assembly in vitro and to reduce its cytotoxicity. Strikingly, although their scaffolds are structurally divergent, the majority of these molecules are densely functionalized with hydrophilic and/or charged groups on their surface. Nonetheless, the relationships between the nature/density of the chemical function and the effect on IAPP amyloid formation have never been explicitly addressed. This lack of knowledge particularly precludes the identification and optimization of amyloid modulators. Moreover, IAPP is a natively disordered polypeptide and needs to undergo transient secondary and quaternary conformational rearrangements to self-assemble intro fibrillary aggregates. These complex structural conversions are not completely understood and are still the matter of active research. Therefore, the rational design of compounds targeting pre-amyloid transient structural states is extremely challenging and molecular scaffolds with high conformational flexibility could demonstrate unique anti-amyloid assembly properties.
In this study, we evaluated the effects of low generation dendrimers decorated with 4 to 16 functional groups on IAPP amyloidogenesis and IAPP-induced pancreatic β-cells toxicity. These dendritic scaffolds are perfectly suited to probe how multi-homofunctionalized molecular templates can modulate the self-assembly of amyloidogenic IDPs. Particularly, these dendrimers were designed to be highly flexible in order to accommodate the wide conformational ensemble of native IAPP and its numerous transient conformations during the assembly process. Moreover, whereas the anti-amyloidogenic potential of large dendrimers was recently recognized for polypeptides related to Alzheimer and prion diseases,13,16,18,49,50 the effects of low generation dendrimers on IDP self-assembly and toxicity have never been assessed so far. We identified a G0 dendrimer decorated with 4 sulfate groups (1d) that efficiently inhibit IAPP amyloid assembly. Interestingly, compound 1d not only prevented fibrillization, but also inhibited IAPP conformational conversion. This indicates that compound 1d interacts with IAPP in its natively disordered secondary structure, preventing its aggregation and its conformational transition into β-sheets competent nucleus. The high flexibility of the G0 scaffold most likely accommodates the large ensemble of distances between the two positively charged residue side chains (Lys,1 Arg11). In turn, this electrostatic-mediated binding of IAPP to compound 1d prevents the interpeptide interactions that are driving amyloid assembly.
Increasing the number of sulfated units from 8 to 16 led to an acceleration of amyloid formation, most likely by a scaffold-based mechanism, as previously reported for highly sulfated GAGs.11,12,32 Whereas the sulfated G0 dendrimer decorated with 4 units (compound 1d) inhibited fibrillization, the G1 2d dendrimer (8 sulfate groups) potentiated IAPP self-assembly. These results offer a novel perspective regarding the relation between the number of sulfate groups on a flexible molecular scaffold and the effects on self-assembly. Over the last fifteen years, several studies have shown that pre-fibrillar intermediates are the most toxic species and that well-defined amyloid fibrils are poorly cytotoxic.51,52 The protective effect observed for polysulfated G1 dendrimers against IAPP-induced toxicity highlights that sequestering proteotoxic species of the amyloidogenic cascade through the acceleration of fibrillization constitute a therapeutic strategy to reduce β-cell loss that deserves further investigation.
References
- F. E. Cohen and J. W. Kelly, Nature, 2003, 426, 905–909 CrossRef CAS PubMed.
- F. Chiti and C. M. Dobson, Annu. Rev. Biochem., 2006, 75, 333–366 CrossRef CAS PubMed.
- C. Soto, Nat. Rev. Neurosci., 2003, 4, 49–60 CrossRef CAS PubMed.
- C. E. Bulawa, S. Connelly, M. Devit, L. Wang, C. Weigel, J. A. Fleming, J. Packman, E. T. Powers, R. L. Wiseman, T. R. Foss, I. A. Wilson, J. W. Kelly and R. Labaudiniere, Proc. Natl. Acad. Sci. U. S. A., 2012, 109, 9629–9634 CrossRef CAS PubMed.
- A. Abedini and D. P. Raleigh, Protein Eng., Des. Sel., 2009, 22, 453–459 CrossRef CAS PubMed.
- D. E. Ehrnhoefer, J. Bieschke, A. Boeddrich, M. Herbst, L. Masino, R. Lurz, S. Engemann, A. Pastore and E. E. Wanker, Nat. Struct. Mol. Biol., 2008, 15, 558–566 CAS.
- J. Bieschke, J. Russ, R. P. Friedrich, D. E. Ehrnhoefer, H. Wobst, K. Neugebauer and E. E. Wanker, Proc. Natl. Acad. Sci. U. S. A., 2010, 107, 7710–7715 CrossRef CAS PubMed.
- F. Meng, A. Abedini, A. Plesner, C. B. Verchere and D. P. Raleigh, Biochemistry, 2010, 49, 8127–8133 CrossRef CAS PubMed.
- H. J. Wobst, A. Sharma, M. I. Diamond, E. E. Wanker and J. Bieschke, FEBS Lett., 2014, 589, 77–83 CrossRef PubMed.
- J. McLaurin, T. Franklin, X. Zhang, J. Deng and P. E. Fraser, Eur. J. Biochem., 1999, 266, 1101–1110 CrossRef CAS PubMed.
- C. A. De Carufel, P. T. Nguyen, S. Sahnouni and S. Bourgault, Biopolymers, 2013, 100, 645–655 CrossRef CAS PubMed.
- S. Jha, S. M. Patil, J. Gibson, C. E. Nelson, N. N. Alder and A. T. Alexandrescu, J. Biol. Chem., 2011, 286, 22894–22904 CrossRef CAS PubMed.
- N. Benseny-Cases, O. Klementieva and J. Cladera, New J. Chem., 2012, 36, 211–216 RSC.
- E. N. Gurzov, B. Wang, E. H. Pilkington, P. Chen, A. Kakinen, W. J. Stanley, S. A. Litwak, E. G. Hanssen, T. P. Davis, F. Ding and P. C. Ke, Small, 2016, 12, 1615–1626 CrossRef CAS PubMed.
- R. Roy and T. C. Shiao, Chem. Soc. Rev., 2015, 44, 3924–3941 RSC.
- P. M. Heegaard, U. Boas and D. E. Otzen, Macromol. Biosci., 2007, 7, 1047–1059 CrossRef CAS PubMed.
- O. Klementieva, N. Benseny-Cases, A. Gella, D. Appelhans, B. Voit and J. Cladera, Biomacromolecules, 2011, 12, 3903–3909 CrossRef CAS PubMed.
- B. Klajnert, T. Wasiak, M. Ionov, M. Fernandez-Villamarin, A. Sousa-Herves, J. Correa, R. Riguera and E. Fernandez-Megia, Nanomedicine: Nanotechnology, Biology and Medicine, 2012, 8, 1372–1378 CrossRef CAS PubMed.
- P. Westermark, A. Andersson and G. T. Westermark, Physiol. Rev., 2011, 91, 795–826 CrossRef CAS PubMed.
- A. Kapurniotu, A. Schmauder and K. Tenidis, J. Mol. Biol., 2002, 315, 339–350 CrossRef CAS PubMed.
- P. T. Nguyen, N. Andraka, C. A. De Carufel and S. Bourgault, Diabetes Res., 2015, 2015, 515307 Search PubMed.
- A. Abedini and A. M. Schmidt, FEBS Lett., 2013, 587, 1119–1127 CrossRef CAS PubMed.
- P. Cao, P. Marek, H. Noor, V. Patsalo, L. H. Tu, H. Wang, A. Abedini and D. P. Raleigh, FEBS Lett., 2013, 587, 1106–1118 CrossRef CAS PubMed.
- A. Abedini, G. Singh and D. P. Raleigh, Anal. Biochem., 2006, 351, 181–186 CrossRef CAS PubMed.
- R. Sharma, N. Kottari, Y. M. Chabre, L. Abbassi, T. C. Shiao and R. Roy, Chem. Commun., 2014, 50, 13300–13303 RSC.
- R. Sharma, I. Zhang, L. Abbassi, R. Rej, D. Maysinger and R. Roy, Polym. Chem., 2015, 6, 1436–1444 RSC.
- R. Sharma, I. Zhang, T. C. Shiao, G. M. Pavan, D. Maysinger and R. Roy, Nanoscale, 2016, 8, 5106–5119 RSC.
- H. Naiki, K. Higuchi, M. Hosokawa and T. Takeda, Anal. Biochem., 1989, 177, 244–249 CrossRef CAS PubMed.
- J. A. Hebda and A. D. Miranker, Annu. Rev. Biophys., 2009, 38, 125–152 CrossRef CAS PubMed.
- A. R. Jesus, C. Dias, A. M. Matos, R. F. de Almeida, A. S. Viana, F. Marcelo, R. T. Ribeiro, M. P. Macedo, C. Airoldi, F. Nicotra, A. Martins, E. J. Cabrita, J. Jimenez-Barbero and A. P. Rauter, J. Med. Chem., 2014, 57, 9463–9472 CrossRef CAS PubMed.
- J. P. Solomon, S. Bourgault, E. T. Powers and J. W. Kelly, Biochemistry, 2011, 50, 2486–2498 CrossRef CAS PubMed.
- S. Bourgault, J. P. Solomon, N. Reixach and J. W. Kelly, Biochemistry, 2011, 50, 1001–1015 CrossRef CAS PubMed.
- N. Motamedi-Shad, E. Monsellier, S. Torrassa, A. Relini and F. Chiti, J. Biol. Chem., 2009, 284, 29921–29934 CrossRef CAS PubMed.
- F. Meng, A. Abedini, A. Plesner, C. T. Middleton, K. J. Potter, M. T. Zanni, C. B. Verchere and D. P. Raleigh, J. Mol. Biol., 2010, 400, 555–566 CrossRef CAS PubMed.
- P. S. Aisen, S. Gauthier, B. Vellas, R. Briand, D. Saumier, J. Laurin and D. Garceau, Curr. Alzheimer Res., 2007, 4, 473–478 CrossRef CAS PubMed.
- F. Meng and D. P. Raleigh, J. Mol. Biol., 2011, 406, 491–502 CrossRef CAS PubMed.
- L. H. Tu, H. Noor, P. Cao and D. P. Raleigh, ACS Chem. Biol., 2014, 9, 1632–1637 CrossRef CAS PubMed.
- D. Radovan, V. Smirnovas and R. Winter, Biochemistry, 2008, 47, 6352–6360 CrossRef CAS PubMed.
- S. Luca, W. M. Yau, R. Leapman and R. Tycko, Biochemistry, 2007, 46, 13505–13522 CrossRef CAS PubMed.
- C. A. De Carufel, N. Quittot, P. T. Nguyen and S. Bourgault, Angew. Chem., Int. Ed., 2015, 54, 14383–14387 CrossRef CAS PubMed.
- J. A. Williamson and A. D. Miranker, Protein Sci., 2007, 16, 110–117 CrossRef CAS PubMed.
- J. A. Williamson, J. P. Loria and A. D. Miranker, J. Mol. Biol., 2009, 393, 383–396 CrossRef CAS PubMed.
- P. Westermark, Upsala J. Med. Sci., 2011, 116, 81–89 CrossRef PubMed.
- T. Tomiyama, H. Kaneko, K. Kataoka, S. Asano and N. Endo, Biochem. J., 1997, 322(3), 859–865 CrossRef CAS PubMed.
- A. Hassanpour, C. A. De Carufel, S. Bourgault and P. Forgione, Chemistry, 2014, 20, 2522–2528 CrossRef CAS PubMed.
- B. Cheng, H. Gong, X. Li, Y. Sun, H. Chen, X. Zhang, Q. Wu, L. Zheng and K. Huang, Proteins, 2013, 81, 613–621 CrossRef CAS PubMed.
- L. M. Yan, M. Tatarek-Nossol, A. Velkova, A. Kazantzis and A. Kapurniotu, Proc. Natl. Acad. Sci. U. S. A., 2006, 103, 2046–2051 CrossRef CAS PubMed.
- D. H. Lopes, A. Attar, G. Nair, E. Y. Hayden, Z. Du, K. McDaniel, S. Dutt, K. Bravo-Rodriguez, S. Mittal, F. G. Klarner, C. Wang, E. Sanchez-Garcia, T. Schrader and G. Bitan, ACS Chem. Biol., 2015, 10, 1555–1569 CrossRef CAS PubMed.
- T. Wasiak, M. Ionov, K. Nieznanski, H. Nieznanska, O. Klementieva, M. Granell, J. Cladera, J. P. Majoral, A. M. Caminade and B. Klajnert, Mol. Pharmaceutics, 2012, 9, 458–469 CrossRef CAS PubMed.
- O. Klementieva, E. Aso, D. Filippini, N. Benseny-Cases, M. Carmona, S. Juves, D. Appelhans, J. Cladera and I. Ferrer, Biomacromolecules, 2013, 14, 3570–3580 CrossRef CAS PubMed.
- R. Kayed, E. Head, J. L. Thompson, T. M. McIntire, S. C. Milton, C. W. Cotman and C. G. Glabe, Science, 2003, 300, 486–489 CrossRef CAS PubMed.
- S. Bourgault, S. Choi, J. N. Buxbaum, J. W. Kelly, J. L. Price and N. Reixach, Biochem. Biophys. Res. Commun., 2011, 410, 707–713 CrossRef CAS PubMed.
Footnotes |
† Electronic supplementary information (ESI) available. See DOI: 10.1039/c6ra15373a |
‡ These authors contributed equally to this work. |
|
This journal is © The Royal Society of Chemistry 2016 |