DOI:
10.1039/C6RA15247C
(Paper)
RSC Adv., 2016,
6, 80213-80220
An in situ self-catalytic hybrid cyanate ester resin and its self-catalytic polymerization behavior†
Received
12th June 2016
, Accepted 16th August 2016
First published on 17th August 2016
Introduction
Novolac cyanate ester (NCE) resins are well-known thermosetting resin with high thermal properties, which can be fully cured to form a triazine network. The polymerized NCE polymers exhibit excellent thermal stability, high strength, low dielectric constants over a wide range of frequencies and temperatures, high glass transition temperature, low water absorption.1–5 These polymers have already shown potential applications as printed circuit boards (PCBs) substrates, heat-resistant materials and matrix of advanced composites in aerospace and astronautics.6–9 However, it is difficult to polymerize completely due to the network structure limiting the trimerization of –OCN after gel point. Beside, in order to make the curing reaction more completely, more time or higher temperature is needed for the NCE system, but which is prone to producing amount of internal stress and defect in the cured NCE. These disadvantages considerably limit the application of NCE system in a variety of fields.10 In view of this, great efforts have been devoted to solving this problem. Up to now, the method of catalytic polymerization mostly was used to reducing the curing temperature and time, and several curing catalysts were developed. The polymerization catalysts mainly contain active hydrogen-containing compound (nonylphenol, diamino diphenyl sulfone, catechol, H3PO4, etc.) and transition metal salt (zinc octoate, manganese octoate, naphthenates, and acetylacetonates).11 But these catalysts would still remain in the polymerized NCE after curing, which can influence the heat resistance and the dielectrical property. In addition, these additional small-molecule catalysts also could induce internal stress and defect during polymerization process, and make the cured NCE with great brittleness.10 Therefore, it is interesting to develop a NCE resin with self-catalytic feature, which could polymerize with less stress and defect at lower temperature and shorter time.
The Si–N and Si–O bonds have high bond energy and good flexibility, and the materials composed of them could have improved heat resistance and better toughness.12,13 Thus, the molecular structure containing the flexible Si–N or Si–O with catalytic effect is hopeful to be introduced into the NCE and form self-catalytic NCE. Polysilazane is a polymer composed of alternative Si–N bond in molecular backbone, which can be used as Si–C–N ceramic precursor and high performance coatings.14–16 The Si–N bond exhibit high active to –NCO which could be inserted into the Si–N bond and form urea groups.17,18 Based on this reaction, Bauer proposed the reaction between Si–N and –OCN, and confirmed the formation of cyanamide group.19 In view of this, the Si–N could be introduced into NCE by the reaction of polysilazane and –OCN, and form the –O–Si–NH–C
N. The N–H of –O–Si–NH–C
N is expected to produce catalytic effect for the cyclotrimerization of –OCN, meanwhile the –O–Si–NH– could reduce the internal stress and defect from curing process.
Therefore, in this paper, we report a self-catalytic hybrid NCE resin which was prepared by the reaction of methylvinylcyclotrisilazane (MVSZ) and novolac cyanate ester (NCE). The synthesis and structure of hybrid resins were examined by Fourier transform-infrared spectroscopy (FT-IR) and hydrogen nuclear magnetic resonance spectroscopy (1H-NMR). The polymerization process of hybrid resins, thermal stability and thermomechanical properties of the polymerized hybrid resins were investigated by differential scanning calorimetry (DSC), FT-IR, thermogravimetric analysis (TGA), dynamic mechanical analysis (DMA).
Experimental
Materials and synthesis
Dichloromethylvinylsilane and dichloromethane were purchased from Shanghai Titan Scientific Co. Ltd. and distilled before use. Ammonia was purchased from Shanghai Jie Meng Chemical Co., Ltd. and dried before use.
Synthesis of NCE. Novolac Cyanate Ester (NCE) was synthesized by the procedures described in the literatures.1 A brown viscous liquid (NCE) was obtained in 82% yield. FT-IR data (cm−1): 2265 (–OCN), 2924 and 3050 (–C–H in aromatic ring). GPC data (solution in THF): 377 (Mn), 493 (Mw), 1.3 (Mw/Mn). Viscosity (mPa s): 620 (60 °C).
Synthesis of MVSZ. The MVSZ was synthesized through the reaction of dichloromethylvinylsilane and ammonia according to procedures previously reported.20,21 A colourless liquid (MVSZ) was obtained in 85% yield. FT-IR data (cm−1) shown in Fig. 10S:† 3300 (N–H), 1635 (–CH
CH2), 930 (Si–N). NMR data (solution in CDCl3) shown in Fig. 11S:† δ (1H, ppm) = 0.18–0.3 (brs, NH), 5.7–6.4 (m, CH2
CH–). δ (29Si, ppm) = −14.7 ([SiMeViNH]3), −17.7 ([SiMeViNH]4).
Preparation of hybrid NCE resins. Hybrid NCE resins were prepared by reaction of MVSZ and NCE as the Scheme 1(a) shown. NCE (198, 196, 190 g, respectively) was added into the 1 L three necked round-bottomed flask while stirring mechanically. The NCE was heated to 65 °C and the viscosity was controlled below 600 mPa s. Then, MVSZ (2, 4, 10 g, respectively) was added into the NCE resin and controlled the reaction temperature below 70 °C. These mixtures were mechanically stirred until no gas emitting, and then the temperature was decreased to ambient temperature. After that, the system pressure was reduced by vacuum pump until no bubble can be found. A viscous liquid (hybrid NCE resins) was obtained in nearly 98% yield. Three kinds of hybrid NCE resins were named Si–NCE-1, Si–NCE-2 and Si–NCE-5 where 1, 2 and 5 respectively stand for mass fraction of MVSZ as 1%, 2% and 5%. FT-IR data (cm−1): 2260 (–OCN), 2200 (–NHCN), 3300–3400 (N–H), 2924 and 3050 (–C–H in aromatic ring), 1640 (–CH
CH2), 930 (Si–N). NMR data (solution in CDCl3): δ (1H, ppm) = 0.18–0.36 (brs, NH), 5.7–6.2 (m, CH2
CH–).
 |
| Scheme 1 (a) Schematic illustration of the synthesis of hybrid NCE resins, (b) the sketch of model reaction between B–Cl and chlorotrimethylsilane. | |
Preparation of polymerized hybrid NCE resins. The hybrid NCE resins were thermally polymerized in an air convection oven according to the cure schedule: 120 °C for 2 h, 150 °C for 1 h, 180 °C for 1 h, and finally 220 °C for 1 h. The cured resins were ejected from the molds then cut and polished according the dimension requirements for the property measurements.
Instrumentation
Fourier transform infrared (FT-IR) spectra were obtained using a Nicolet 550 spectrometer. 1H-NMR analyses were performed on a BRUKER AVANCE 400 (400 MHz) instrument, using tetramethylsilane (TMS) as an external standard in CDCl3 solution. Differential scanning calorimetric analyses (DSC) were performed on a NETZSCH 200 PC module. Thermogravimetric analyses (TGA) were performed on a TA Instruments SDT Q600 analyzer. All thermal analyses were conducted under nitrogen atmosphere at a heating rate of 10 °C min−1. Dynamic mechanical analysis (DMA) was performed with a TA DMA 2980 Dynamic Mechanical Analyzer with a sample size of 45 mm × 6 mm × 3 mm. The storage modulus E′ and tan
δ were determined as the sample was subjected to the temperature scan mode at a heating rate of 10 °C min−1 at a frequency of 1 Hz under nitrogen atmosphere.
Results and discussion
Preparation of hybrid NCE resins
Hybrid NCE resins were prepared through the reaction between Si–NH of methylvinylcyclotrisilazane (MVSZ) and –OCN of novolac cyanate ester (NCE) with different mass ratio. Three kinds of obtained hybrid NCE resins were named Si–NCE-1, Si–NCE-2 and Si–NCE-5 according to their mass fraction of MVSZ. Experimentally, the obtained hybrid NCE resins were a brown viscous liquid and soluble in a variety of common solvents such as THF, toluene, dioxane, DMF, acetone, etc.
The formation mechanism of hybrid resins is important to figure out the preparation of hybrid NCE resin and their structure, and the reaction process between Si–NH and –OCN will be discussed according to the electronic properties of the reactive groups. The O–C bond in –OCN is prone to be polarized due to the higher electronegativity of the oxygen and nitrogen atom than carbon atom, and exhibit a negative partial charge on the oxygen atom and a positive partial charge on the carbon atom. Also, a negative partial charge on the nitrogen atom in Si–NH can be believed. According to the electronic property of the –OCN and Si–NH, Bauer proposed that the carbon atom with positive charge tend to attack the nitrogen atom, and the nitrile group would be transferred to the nitrogen atom through the cleavage of O–C and N–H bond as the route 2 of Scheme 1(a) shown.19 After that, the nitrile-substituted MVSZ and NCE containing a phenolic hydroxyl group are produced, the phenolic hydroxyl group is extremely easy to cleave the Si–N bond, and the hybrid resins containing –O–Si–NH–C
N is obtained.22 However, to our curiosity, the route 2 describing the reaction of C–O with N–H prior to Si–N arouse our doubt. To figure out the confusion, the B–Cl bond with vacant p orbital for boron atom and high electronegativity for chlorine atom were selected to simulate the property of C–O bond. Hexamethyldisilazane is used to be a model compound to provide Si–N–H. Meanwhile, the model reaction is constructed through the reaction of B–Cl and Si–N–H as Scheme 1(b) shown. According to the previous report, this reaction could produce B–NH and chlorotrimethylsilane, and no HCl can be found.23 Thus, the correct of the route 2 still need to be discussed. In view of this, we propose that the reaction should occur as route 1 of Scheme 1(a) shown, the nitrile group would be transferred to the nitrogen atom and the oxygen atom attached to silicon atom by the cleavage of O–C and Si–N bond, and finally the hybrid resins are obtained. Therefore, the prepared reaction of hybrid NCE could be assumed as route 1. Based on the discussion of the prepared reaction, the obtained hybrid NCE resins should contain cyanamide groups, N–H and Si–CH
CH2 groups. In order to confirm the proposed structure, FT-IR and 1H-NMR were carried out.
FT-IR spectra of the hybrid NCE resins are shown in Fig. 1. The FT-IR spectra of NCE appear in Fig. 1 for comparison. The absorption band at 2260 cm−1 is the –O–C
N stretch vibration. Also, the significant peaks assigned to the characteristic absorption of triazine ring could be observed at 1380 cm−1 and 1560 cm−1, and their intensity increase with the addition of MVSZ. The appearance of new bands at around 3300–3400 cm−1 and 2200 cm−1 confirms the conversion of –O–C
N into a cyanamide group (–NH–C
N) during preparation of hybrid resins. The appearance of bands at 1640 cm−1 and 930 cm−1 confirms the existence of Si–CH
CH2 and Si–N bond. In addition, it can be seen that the intensity of the characteristic absorption of cyanamide group, N–H, Si–CH
CH2 and Si–N bond exhibit an increasing trend with the addition of MVSZ. These structural information indicate that the obtained hybrid NCE resins are consistent with designed structures as Scheme 1 shown. The chemical structures of the synthesized hybrid NCE resins were further determined by 1H-NMR (Fig. 2). Two new resonances located at 0.18–0.36 ppm and 5.7–6.2 ppm were observed, which are attributed to the protons of –NH–C
N and Si–CH
CH2 and become obvious from Si–NCE-1 to Si–NCE-5. Also, it could be found that the characteristic peak at around 0 ppm assignable to Si–CH3 become more obvious with the addition of MVSZ. These characteristic structures further confirms the designed structure of hybrid resins. Therefore, it is concluded that the catalytic group, –O–Si–NH–C
N, was formed and its contents become more with the increase of reactants, MVSZ.
 |
| Fig. 1 FT-IR spectra of the hybrid NCE resins. | |
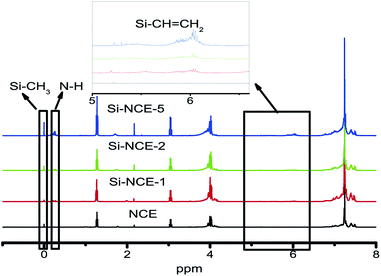 |
| Fig. 2 1H-NMR spectra of the hybrid NCE resins in CDCl3. | |
Catalytic polymerization behavior of hybrid NCE resins
Polymerization reaction of hybrid NCE resins. The process of polymerization reaction is important to investigate the self-catalytic behavior, the in situ FT-IR was utilized to reveal the chemical reaction of hybrid NCE resins from 100 °C to 300 °C with a heating rate of 5 °C min−1, and the spectra was recorded every five minutes. The FT-IR spectra of the NCE and Si–NCE-5 at each cure cycle are shown in Fig. 3. The intensity of the characteristic absorption peaks at 2265 cm−1 assigned to typical –OCN stretches weaken gradually with the temperature increasing and vanish at 300 °C for Si–NCE-5, but still can be found at 300 °C for NCE. This could be better understood by the changes of conversion for –OCN during the process from Fig. 3(a) and (b). It clearly can be found from Fig. 3(c) and (d) that the conversion of –OCN for Si–NCE-5 (92.1% after 55 min) is higher than that of NCE with 43.1% after 55 min, which suggest the self-catalytic behaviour for Si–NCE-5. Meanwhile, the triazine derived from trimerization of –OCN at 1563 and 1361 cm−1 appear gradually,24 and become apparent from 175 °C for Si–NCE-5 and 225 °C for NCE. This result confirm the self-catalytic effect of Si–NCE-5. In addition, for Si–NCE-5, the bands at 1640 cm−1 and 2200 cm−1 corresponding to the Si–CH
CH2 and –NH–C
N also decrease gradually with the temperature increasing, and disappeared after 200 °C for –NH–C
N. It is also noteworthy that the width of the characteristic peak of hybrid NCE resins at 1361 cm−1 is wider than NCE, and become wider with the content of –NH–C
N increasing shown in Fig. 3(b), 1S and 2S.† This can be caused by the asymmetric triazine ring from trimerization of –NH–C
N and –OCN.19 Therefore, it can be concluded that the self-polymerization of hybrid NCE resins mainly contains cyclotrimerization of –OCN and trimerization between NH–C
N and –OCN, and the polymerization can occur at lower temperature by catalytic effect from N–H of –O–Si–NH–C
N.
 |
| Fig. 3 (a) FT-IR spectra of NCE resins at different temperature with 5 °C min−1, (b) FT-IR spectra of Si–NCE-5 at different temperature with 5 °C min−1, (c) the curve of conversion of group (–OCN) versus time for NCE, (d) the curve of conversion of group (–OCN) versus time for Si–NCE-5. | |
Self-catalytic behavior of hybrid NCE resins. To determine the self-catalytic behavior of the hybrid NCE resins, differential scanning calorimetry (DSC) were used for analyzing the polymerization behavior of the hybrid NCE resins under different treated temperature. The hybrid resins were treated according to the procedure: 120 °C/2 h, 150 °C/1 h and 180 °C/1 h, and the test samples of DSC were selected at different temperature respectively. The DSC curves of the hybrid NCE resins at different treatment temperatures are shown in Fig. 4 and the feature data are collected in Table 1. It is obvious that the hybrid NCE resins exhibit lower initial polymerized temperature (Ti), exotherm peaking temperature (Tp) and polymerized heat (ΔH) than NCE with 219.6 °C (Ti), 249.9 °C (Tp) and 611.2 J g−1 (ΔH), which indicate that the existence of –O–Si–NH–C
N have excellent catalytic effect for the polymerization reaction. Also, the Ti and Tp of untreated hybrid NCE resins decrease with the temperature, and become more obvious with its contents increasing. Besides, it can be observed that the ΔH exhibit the same tendency as Ti and Tp with the increase of contents of –O–Si–NH–C
N for untreated hybrid NCE resins from Fig. 4 and Table 1. This can be explained that the prepolymerization of –O–C
N occurred during the preparation of hybrid NCE resins, which could be confirmed by the formation of triazine ring from the FT-IR spectra (shown in Fig. 1). The prepolymerization could release some polymerized heat which increase with the increase of reactants (MVSZ), and could avoid the insecurity from the explosive polymerization of –O–C
N.25,26
 |
| Fig. 4 DSC thermogram (10 °C min−1 in N2) of hybrid NCE resins polymerized at different treatment temperatures: (a) untreated hybrid NCE resins, (b) hybrid NCE resins treated after 120 °C for 2 h, (c) hybrid NCE resins treated after 120 °C for 2 h and 150 °C for 1 h, (d) hybrid NCE resins treated after 120 °C for 2 h, 150 °C for 1 h and 180 °C for 1 h. | |
Table 1 The thermal data of hybrid NCE resins polymerized at different treatment temperature
Sample |
Untreated |
120 °C for 2 h |
150 °C for 1 h |
180 °C for 1 h |
Ti (°C) |
Tp (°C) |
ΔH (J g−1) |
Ti (°C) |
Tp (°C) |
ΔH (J g−1) |
Ti (°C) |
Tp (°C) |
ΔH (J g−1) |
Ti (°C) |
Tp (°C) |
ΔH (J g−1) |
NCE |
219.6 |
249.9 |
611.2 |
216.5 |
248.3 |
580.0 |
200.6 |
241.2 |
547.6 |
224.8 |
273.2 |
442.7 |
Si–NCE-1 |
180.5 |
243.4 |
591.9 |
178.4 |
222.4 |
492.8 |
182.9 |
236.2 |
322.2 |
191.9 |
239.6 |
260.7 |
Si–NCE-2 |
176.5 |
227.4 |
572.3 |
168.6 |
214.8 |
286.7 |
174.3 |
231.1 |
276.5 |
193.4 |
238.2 |
194.8 |
Si–NCE-5 |
162.2 |
201.8 |
558.5 |
172.5 |
222.1 |
179.4 |
175.6 |
225.7 |
176.9 |
204.8 |
258.6 |
48.2 |
The Fig. 4 and Table 1 also show that the Ti and Tp of Si–NCE-1 and Si–NCE-2 both increase at first, and then decrease with the increase of the treatment temperature. This results could be ascribed to the change of the contents of –O–Si–NH– relative to –O–C
N and the limit of formed network structures during the polymerized process. The contents of –O–C
N decrease with the treatment temperature increasing due to the cyclotrimerization consuming –O–C
N, which lead to the relative contents of catalytic structures (–O–Si–NH–) for the treated sample at 120 °C more than the untreated and exhibit higher active and lower Ti and Tp. With the treatment temperature further increasing to 150 °C, the cross-linked network structures were formed and the –O–Si–NH– and –O–C
N were limited in these network structures, which cause the polymerized reaction being restricted and needing higher temperature to overcome the limitation from network structures. Therefore, the polymerized reaction of hybrid NCE resins can be divided into two steps: at the beginning stage, the polymerized reaction is dominated by catalytic effect of –O–Si–NH–, and at the second stage, the polymerized reaction depend on the conformational transition to overcome the limit of network structures at higher curing temperature. However, the Si–NCE-5 shows a different tendency from Si–NCE-1 and Si–NCE-2 as Fig. 4 and Table 1 demonstrated, and the Ti and Tp of Si–NCE-5 increase with the treatment temperature increasing. This could be explained that the cross-linked network structures could be formed for the Si–NCE-5 treated at 120 °C, and the polymerized reaction is dominated by the conformational transition after the treatment temperature exceeding 120 °C.
The polymerization kinetic study of hybrid NCE can be performed with nonisothermal DSC scans at different heating rates, 2, 5, 10, 20 °C min−1 (shown in Fig. 5(a) and (b), and 4S and 5S†), to determine the apparent activation energy of the polymerization process. Also, the conversion of hybrid NCE during curing reaction at different heating rate were be shown in Fig. 5(c) and (d), and 6S and 7S† according to the nonisothermal DSC by the equation: dα/dT = (dH/dT)/ΔHt, where ΔHt is total reaction heat.27 The Kissinger method and self-catalytic mode have been used to determine the activation energy (Ea) and establish the kinetic equation based on these DSC results shown in Fig. 6(a), 8S and 9S.†28 The activation energies calculated from the slope of the Kissinger plots are about 93.83 kJ mol−1 for NCE, 89.21 kJ mol−1 for Si–NCE-1, 84.64 kJ mol−1 for Si–NCE-2 and 80.31 kJ mol−1 for Si–NCE-5 (as shown in Table 1S†). This indicate that the introduction of –NH–CN obviously decrease the activation energy of curing reaction and exhibit excellent self-catalytic behaviour. Also, the order of curing reaction (n and m) can be seen from Table 1S† as 2.42 and 1, 2.37 and 1, 2.31 and 1, 2.29 and 1 for NCE, Si–NCE-1, Si–NCE-2 and Si–NCE-5, respectively. The reduced n could give an information that the introduction of –NH–CN decrease the influence on curing reaction rate from the concentration of reactive group, –OCN, in local region of hybrid NCE. This can be explained that the –O–Si–NH– structure make the conformational transition of –NCO easier, overcome the limit from network structure and form helpful conformation to curing reaction. Moreover, the curing kinetic equation of hybrid NCE are established according to the obtained kinetic parameter (Table 1S†) as dα/dt = 1.45 × 109(1 − α)2.42α1.01
exp(−1.13 × 104/T), dα/dt = 1.77 × 109(1 − α)2.37α0.99
exp(−1.07 × 104/T), dα/dt = 8.58 × 108(1 − α)2.31α0.99
exp(−1.02 × 104/T), and dα/dt = 3.61 × 108(1 − α)2.29α0.98
exp(−9.66 × 103/T).
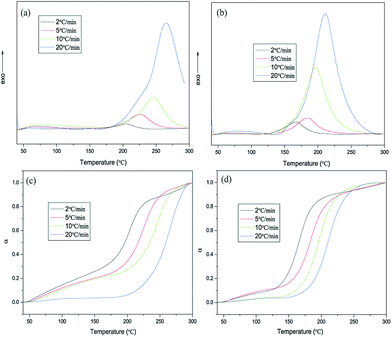 |
| Fig. 5 (a) DSC thermogram of NCE at different heating rate under N2, (b) DSC thermogram of Si–NCE-5 at different heating rate under N2, (c) the conversion of NCE versus temperature at different heating rate, (d) the conversion of Si–NCE-5 versus temperature at different heating rate. | |
 |
| Fig. 6 (a) Kissinger plot for determination of the activation energy of NCE and Si–NCE-5, (b) dependence of polymerized degree of hybrid NCE resins on the treatment temperature. | |
In order to examine the catalytic effect of polymerized process, polymerized degree, α, was defined as α = 1 − ΔHT/ΔHi, where ΔHT is the reaction heat at the treatment temperature, T, ΔHi is the total reaction heat of untreated samples.29 The dependence of the polymerized degree of hybrid NCE resins with the treatment temperature are shown in Fig. 6(b). The higher polymerized degree of hybrid NCE resins can be observed than NCE treated at 180 °C with 27.6%, especially for Si–NCE-5 treated at 120 °C with 67.9% and 180 °C with 91.4%. This further confirms the excellent catalytic effect of –O–Si–NH–. It also can be seen that the polymerized degree of Si–NCE-2 and Si–NCE-5 exhibit great change at 25–120 °C and 180 °C, and little change among 120–180 °C. This indicate that the –O–Si–NH– could catalyze the polymerization of hybrid NCE resin not only at low temperature but also high temperature. Two reason can be responsible for this results, (1) the catalytic effect from N–H in –O–Si–NH–, (2) lager bond angle of –O–Si–NH– prone to overcoming the limitation from network structures confirmed by lower relaxation temperature shown in Fig. 6(b). Therefore, according to polymerized process discussed last paragraph, the catalytic process can be described as Scheme 2 during polymerization reaction. At the initial stage of polymerization between ambient temperature and 120 °C, the N–H from –O–Si–NH– have large catalytic region due to high motility of –O–Si–NH–C
N (as the structure (a) shown in Scheme 2). With the treatment temperature increasing further, the asymmetric triazine ring is produced by the reaction of NH–C
N and –O–C
N, and the cross-linked network structures are formed, which limit the catalytic region of N–H and the conformational transition of –O–C
N. But, when the treatment temperature up to relaxation temperature of molecular structure, the limit from the network structures is overcame, and the –O–Si–NH– and left –O–C
N could form the conformation helpful to catalytic polymerization and produce some catalytic region (as the structure (b) shown in Scheme 2). Also, the result of DMA show that the –O–Si–NH– could effectively decrease the relaxation temperature, which ensure the catalytic effect during the polymerization process and further confirm the description as Scheme 2 shown. Therefore, it is concluded that the –O–Si–NH– could catalyze the polymerization not only at lower temperature but also higher temperature. In contrast, the polymerized degree of Si–NCE-1 exhibit different tendency, which is probably caused by the lower content of –O–Si–NH–C
N. Even this, the Si–NCE-1 also shows higher self-catalytic effect than NCE.
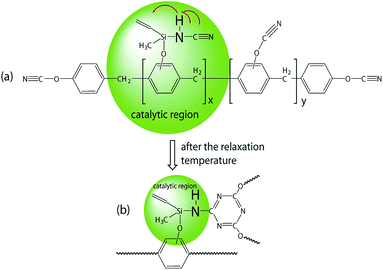 |
| Scheme 2 Schematic illustration of catalytic behavior of N–H during the polymerization process. | |
Thermal properties of cured hybrid NCE resins
Thermal stability of cured hybrid NCE resins. An important property of hybrid NCE resins, their thermal stability, has been investigated by TGA and the TGA thermogram of cured hybrid NCE resins is shown in Fig. 7(a). The thermal stability of the cured hybrid NCE resins was evaluated with its 5% weight loss temperature (Td5) and the residue yield at 1000 °C. It could be observed that the cured NCE, Si–NCE-1 and Si–NCE-2 and Si–NCE-5 give residue yield 62.2%, 62.1%, 63.5% and 61.8%, when heated to 1000 °C, the Td5 reach to 425 °C, 424 °C, 426 °C and 424 °C, respectively. Obviously, there are no big difference for the thermal stability between cured hybrid NCE resins and NCE, which indicate that the catalytic group, –O–Si–NH–C
N, has no great influence on the thermal stability of the cured resins. To understand the thermal decomposition process, DTG (derivative thermogravimetric analysis) curves are shown as Fig. 7(b). Two decomposition stages can be found at 380–500 °C and 520–620 °C, and the second stage become more and more obvious with the content of –O–Si–NH–C
N from NCE to Si–NCE-5. This indicate that a new heat resistance structure in hybrid NCE resins was probably formed during polymerization, and likely to be the asymmetric triazine rings. Therefore, the decomposition process probably can be described that symmetric triazine rings from trimerization of –OCN begin to decompose at 380–500 °C, and with the temperature increasing to 520 °C, the decomposition event occur for the asymmetric triazine rings from trimerization of NH–C
N and –OCN. Therefore, the appearance of the new heat resistance structure indicate introduction of NH–C
N could partly improve the decomposed temperature.
 |
| Fig. 7 (a) TGA thermogram (10 °C min−1 in N2) of cured hybrid NCE resins. (b) DTG thermogram of cured hybrid NCE resins. | |
Thermomechanical property of cured hybrid NCE resins. The dynamic mechanical properties were measured for the cured hybrid NCE resins in order to find out the effect of introducing –O–Si–NH–C
N. Fig. 8 display the thermomechanical spectra for the storage modulus (E′) and tan
δ as a function of the temperature for hybrid NCE resins. Accordingly, Table 2 summarizes the obtained characteristic storage modulus (E′), and glass transition temperature (Tg). The data suggest that polymerized hybrid NCE resins have a higher E′ than NCE blew 150 °C. Impressively, the polymerized Si–NCE-1 shows an E′ value of 1731 MPa, 17% higher than NCE, but the Si–NCE-2 and Si–NCE-5 exhibit slight higher than NCE. This could be explained that the enhanced E′ for Si–NCE-1 is attributed to the increased cohesive energy density from π–π interaction between polarizable asymmetric triazine rings and aromatic rings,30,31 but for Si–NCE-2 and Si–NCE-5, the increase of flexible structures, –O–Si–NH–, limits the enhance of E′. As the temperature increase, E′ of the hybrid NCE resins and NCE decreases steadily due to the increased chain mobility as Fig. 6(a) shown and the hybrid NCE resins decrease more quickly than NCE, especially for Si–NCE-2 and Si–NCE-5, which can be ascribed to the increased chain mobility caused by –O–Si–NH–. In addition, it is interesting that the Tg and peak height of tan
δ show strong regularity and dependence with the content of –O–Si–NH– as Fig. 6(b) shown. The cured hybrid NCE resins exhibit lower Tg and higher peak height. This indicate that as the increase of –O–Si–NH–, the chain mobility become easier, and endow the hybrid NCE resins with decreased molecular chain relaxation temperature and higher damping energy dissipation, which will benefit the transformation of the potentially damageable vibration energy to heat and reduction of the internal stress produced by polymerized process.
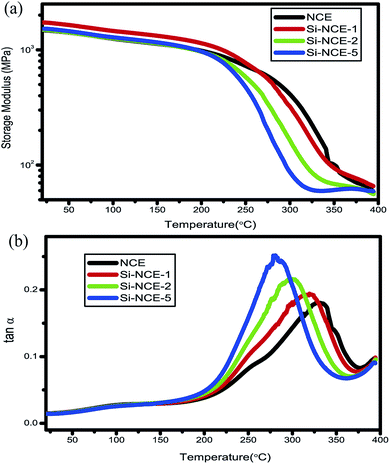 |
| Fig. 8 DMA spectra (5 °C min−1) of hybrid NCE resins polymerized after 220 °C: (a) the storage modulus (E′) against temperature, and (b) tan δ against temperature. | |
Table 2 The thermomechanical data of cured hybrid NCE resins
|
E′ at ambient temperature (MPa) |
Tg (°C) |
Peak height of tan δ |
NCE |
1480 |
331.1 |
0.14 |
Si–NCE-1 |
1731 |
319.4 |
0.16 |
Si–NCE-2 |
1502 |
299.1 |
0.17 |
Si–NCE-5 |
1526 |
280.5 |
0.20 |
Conclusions
A hybrid novolac cyanate ester (NCE) with self-catalytic function that incorporates –Si–NH–C
N group by the reaction between MVSZ and NCE could result in significant decrease in the polymerization temperature without any added initiator and catalyst. Its catalytic polymerization is dominated by the cyclotrimerization of –OCN and trimerization between NH–C
N and –OCN, and the asymmetric and symmetric triazine ring were formed. The investigation of polymerization process reveal that the N–H from O–Si–NH could catalyze the polymerization not only at lower temperature but also higher temperature even the limit from network structures, and endow the polymerized hybrid NCE with higher polymerized degree than NCE. The result from thermomechanical properties reveal that the catalytic effect of –O–Si–NH– could improve the storage modulus and reduce the internal stress produced by polymerization process. Therefore, the obtained hybrid NCE resins could be highly attractive for the industrial use, and the introduction of –Si–NH–C
N to produce the catalytic effect for cyanate ester system is a promising method for modifying disadvantages of cyanate ester during polymerization.
Acknowledgements
The authors gratefully acknowledge the support of the National Science Foundation of China (Grant No. 51103038).
Notes and references
- C. P. R. Nair, D. Mathew and K. N. Ninan, Adv. Polym. Sci., 2001, 155, 1 CrossRef CAS.
- M. S. Lakshmi and B. S. R. Reddy, Eur. Polym. J., 2003, 38, 795 CrossRef.
- G. Liang and M. Zhang, J. Appl. Polym. Sci., 2002, 85, 2377 CrossRef CAS.
- T. J. Wooster, S. Abrol, J. M. Hey and D. R. MacFarlane, Composites, Part A, 2004, 35, 75 CrossRef.
- A. J. Guenthner, G. R. Yandek, M. E. Wright, B. J. Petteys, R. Quintana, D. Connor, R. D. Gilardi and D. A. Marchant, Macromolecules, 2006, 39, 6046 CrossRef CAS.
- Z. Y. Zhang, R. Yu, F. Wang, J. Wang and Y. Jiao, J. Appl. Polym. Sci., 2011, 120, 3716 CrossRef CAS.
- G. Anuradha and M. Sarojadevi, J. Appl. Polym. Sci., 2008, 110, 938 CrossRef CAS.
- L. Yuan, S. Huang, A. Gu, G. Liang, F. Chen, Y. Hu and S. Nutt, Compos. Sci. Technol., 2013, 87, 111 CrossRef CAS.
- C. P. Sakthidharan, P. R. Sundararajan and M. Sarojadevi, RSC Adv., 2015, 5, 19666 RSC.
- O. Georjon, J. Galy and J.-P. Pascault, J. Appl. Polym. Sci., 1993, 49, 1441 CrossRef CAS.
- H. Wang, L. Yuan, G. Liang and A. Gu, RSC Adv., 2016, 6, 3290 RSC.
- F. S. Model, G. Redl and E. G. Rochow, J. Polym. Sci., Part A: Polym. Chem., 1966, 4, 639 CrossRef CAS.
- J. Parrick, Polymer International, ed. R. G. Jones, The Royal Society of Chemistry, Cambridge, 1st edn, 1997, vol. 42, p. 235 Search PubMed.
- S. H. Koo, S. G. Lee, H. Bong, Y. Cho, H. S. Lim and J. H. Cho, Polymer, 2014, 55, 2661 CrossRef CAS.
- R. Riedel, G. Mera, R. Hauser and A. Klonczynski, J. Ceram. Soc. Jpn., 2006, 114, 425 CrossRef CAS.
- P. Colomboo, G. Mera, R. Riedel and G. D. Soraru, J. Am. Ceram. Soc., 2010, 93, 1805 Search PubMed.
- N. Janakiraman and F. Aldinger, J. Eur. Ceram. Soc., 2009, 29, 163 CrossRef CAS.
- T. J. Cross, R. Raj, T. J. Cross, S. V. Prasad and D. R. Tallant, Int. J. Appl. Ceram. Technol., 2006, 3, 113 CrossRef CAS.
- M. Bauer, D. Decker, F. Richter and J. Gwiazda, US20120135217A1, 2012.
- D. Seyferth and R. Stewart, Appl. Organomet. Chem., 1997, 11, 813 CrossRef CAS.
- Q. Hu, H. Qi, F. Wang and Y. Zhu, Adv. Mater. Res., 2014, 1004–1005, 286 Search PubMed.
- Z. Guo, H. Li, W. Han and T. Zhao, J. Appl. Polym. Sci., 2013, 128, 3356 CrossRef CAS.
- R. Rye, T. Theodore, T. Borek, D. A. Linquist and D. A. R. T. Paine, J. Am. Ceram. Soc., 1990, 73, 1409 CrossRef CAS.
- X. Li, Y. Xia, W. Xu, Q. Ran and Y. Gu, Polym. Chem., 2012, 3, 1629 RSC.
- T. Iijima, T. Kaise and M. Tomoi, J. Appl. Polym. Sci., 2003, 88, 1 CrossRef CAS.
- M. F. Zeng, X. D. Sun and X. D. Yao, J. Appl. Polym. Sci., 2010, 115, 338 CrossRef CAS.
- H. Cui, K. Suganuma and H. Uchida, RSC Adv., 2015, 5, 2677 RSC.
- S. Ohashi, J. Kilbane, T. Heyl and H. Ishida, Macromolecules, 2015, 48, 8412 CrossRef CAS.
- J. Moosburger-will, M. Greisel, M. Sause, R. Horny and S. Horn, J. Appl. Polym. Sci., 2013, 130, 4338 CAS.
- B. G. Harvey, A. J. Guenthner, W. W. Lai, H. A. Meylemans, M. C. Davis, L. R. Cambrea, J. T. Reams and K. T. Lamison, Macromolecules, 2015, 48, 3173 CrossRef CAS.
- J. Wan, B. Gan, C. Li, M. Jon, Z. Li, X. Wang and D. Wang, J. Mater. Chem. A, 2015, 3, 21907 CAS.
Footnote |
† Electronic supplementary information (ESI) available. See DOI: 10.1039/c6ra15247c |
|
This journal is © The Royal Society of Chemistry 2016 |
Click here to see how this site uses Cookies. View our privacy policy here.