DOI:
10.1039/C6RA13060G
(Paper)
RSC Adv., 2016,
6, 62263-62269
Hematite homojunctions without foreign element doping for efficient and stable overall water splitting†
Received
19th May 2016
, Accepted 20th June 2016
First published on 22nd June 2016
Abstract
A new strategy to prepare a hematite homojunction photoanode is proposed in this work. Hematite films with different Fermi levels are obtained by tuning the carrier concentrations in the films. This enables us to fabricate hematite homojunction photoelectrodes without foreign element doping by constructing bilayer films with different Fermi levels, and realize enhanced photoelectric conversion efficiency for highly efficient solar water splitting. The photocurrent density for the hematite homojunction is ∼1.1 mA cm−2 at 1.23 V vs. RHE, which is almost 5 times that of single type hematite photoanodes. What is more, with this homojunction configuration, the hematite homojunctions show a 15 times higher stability compared with high-donor-concentration Fe2O3 photoelectrodes for oxygen evolution. Most importantly, overall water splitting is realized and the photocell still holds 92.2% of the efficiency for water oxidation even after 73 hours.
Introduction
Due to the increasing global demand for energy, as well as the growing awareness of environment protection, searching for renewable energy has become attractive and urgent.1 Taking advantage of solar energy and conversion into clean energy sources such as CH4 (ref. 2) and H2 (ref. 3) has received lots of interest in recent years. Solar water splitting using semiconductor-based photoelectrochemical (PEC) devices offers a promising choice to produce clean energy.4,5 An improvement of the photoelectrode is directly related to the photoelectric conversion efficiency of the PEC system. Since oxygen vacancies are inevitably introduced into the oxide at a low concentration in the general material preparation process, most oxide semiconductors show n-type characteristics (Fermi level near the conduction band) and serve as photoanodes for oxygen evolution. These unintentionally introduced oxygen vacancies also play an important role in photocatalytic applications by serving as reaction sites6–8 or by affecting the light absorption9 of semiconductors.
Forming homojunctions offers an effective way to realize high charge separation efficiency via the construction of a large magnitude interfacial electric field within a semiconductor.10 Besides, as the compositions on both sides of the interface in the homojunction region are the same, the continuous band bending will facilitate the transport of photo-generated electron/hole carriers across the interface.10 However, literature about the application of homojunctions in PEC water splitting are limited except for p-Si/n-Si11 and W-BiVO4/BiVO4 (ref. 12), since the formation of homojunctions in films is much more difficult than that in powders. As previous examples of homojunction photoelectrodes are normally prepared by foreign element doping,11,12 the doped foreign elements will also function as recombination sites that can decrease the photo conversion efficiency to some extent.
The Fermi level of an n-type oxide semiconductor can be modulated near to the conduction band by increasing the carrier concentration in the semiconductor.9 This makes it possible to construct homojunction photoelectrodes within the same semiconductor possessing two different Fermi levels. In this work, we realized the construction of n–n hematite homojunction photoelectrodes without foreign element doping by controlling carrier concentrations. Hematite (α-Fe2O3) was studied as it is one of the most attractive candidates for PEC water oxidation due to its excellent visible light response and good stability, non-toxicity, and earth-abundance.13,14 Pulsed laser deposition (PLD) was used to prepare hematite homojunction photoelectrodes since it can effectively control the densities of oxygen vacancies during the deposition process,15,16 and ultimately change the donor densities of the hematite films. Considering the charge flow in the homojunction as well as the water oxidation reaction occurring on the photoelectrodes, hematite with a higher donor concentration (higher Fermi level) was prepared as the inner layer, while that with a lower donor concentration (lower Fermi level) was prepared as the outer layer. The PEC performances of hematite were remarkably enhanced due to the interfacial electric field formed in the homojunction. Besides, overall water splitting was realized by the hematite homojunction photoelectrode with H2
:
O2 = 2
:
1 even for more than 70 hours. The photocurrent density for the hematite homojunction is ∼1.1 mA cm−2 at 1.23 V versus the reversible hydrogen electrode (vs. RHE), which is almost 5 times that of single type hematite photoanodes. With this homojunction configuration, the hematite homojunctions showed a 15 times higher stability compared with the high-vacancy Fe2O3 photoelectrodes for oxygen evolution.
Experimental section
Preparation of high-vacancy Fe2O3 (HV-Fe2O3) and low-vacancy Fe2O3 (LV-Fe2O3) and HV/LV-Fe2O3 homojunction films by PLD
Both the HV-Fe2O3 and LV-Fe2O3 were prepared by PLD (ST-PLD; Pascal Co. Ltd, Japan) method using Fe2O3 pellets as targets and ITO as a substrate. It should be noted here that the two types of hematite layers were prepared with different targets. The HV-Fe2O3 target (defined as Target 1, black in color) was purchased from Hefei Kejing Co. Ltd, China. The LV-Fe2O3 target (defined as Target 2, dark red in color) was made by heating hematite powders at 900 °C for 24 hours.
The temperature for deposition was 550 °C. The film preparation chamber of the PLD machine was firstly evacuated to 0.001 Pa. Then for the preparation of HV-Fe2O3, no gas was introduced into the chamber with the target directly irradiated by the laser for different amounts of time. However, for the preparation of LV-Fe2O3, pure O2 was introduced into the chamber until the pressure became 4 Pa, then the laser began to irradiate for different amounts of time. After the laser irradiation, the film was kept at the same temperature for 30 min as a post-heat treatment.
For the preparation of the HV/LV-Fe2O3 homojunction films: firstly, the chamber of the PLD machine was evacuated to 0.001 Pa. Then the target was irradiated in a pure vacuum to produce HV-Fe2O3. After that, pure O2 was introduced into the chamber until the pressure became 4 Pa. Subsequently, the target was irradiated again to produce LV-Fe2O3. Finally, the film was kept at the same temperature for 30 min as a post-heat treatment. In all of these steps, the temperature of the substrate was 550 °C.
Characterization
XRD (RIGAKU Rint-2000 X-ray diffractometer) was used to test the phase of the hematite films. Raman spectroscopy (NRS-1000, Jasco Corp. Japan) was used to characterize the Raman scattering spectra of the samples. Field-emission scanning electron microscopy (SEM) (JSM-6701 F, JEOL) and atomic force microscopy (AFM) (Nanocute H, Japan) were used to characterize the surface morphology, thickness and roughness of the samples. Optical absorption properties of the Fe2O3 films were measured by a UV-vis spectrophotometer (UV-2500PC; Shimadzu Co., Japan). X-ray photoelectron spectroscopy (XPS) experiments were performed in a Theta probe (Thermo Fisher) using monochromated Al Kα X-rays at hν = 1486.6 eV.
Photoelectrochemical performances
Photoelectrochemical measurements were performed by an electrochemical work station (ALS/CH model 650A). Pt was used as the counter electrode while Ag/AgCl was used as the reference electrode. Air mass 1.5G solar simulation (WXS-80C-3 AM 1.5G) was utilized as the light source without using any filter. The solution during PEC characterization was 1 M NaOH aqueous solution. All the tests were held in a three-electrode system.
Water splitting
Hydrogen and oxygen generation over HV/LV-Fe2O3 homojunction photoanodes were tested in a water splitting system. A 1 cm2 HV/LV-Fe2O3 film was deposited on a 2 × 1 cm2 ITO substrate. A 1 M NaOH solution (180 ml) was put in a quartz glass cell as the source for water splitting. Then this glass cell was connected to the water splitting system with a closed gas circulation system. Before starting the water splitting, the reaction system was evacuated. The generated H2 and O2 were analyzed in situ with a TCD gas chromatograph (Shimadzu GC-8AIT, argon carrier). AM 1.5G solar simulation (WXS-80C-3 AM 1.5G) was utilized as the light source, and the external bias was 1.23 V vs. RHE.
Results and discussion
Characterization of high-vacancy Fe2O3 (HV-Fe2O3) and low-vacancy Fe2O3 (LV-Fe2O3)
The high-vacancy Fe2O3 (HV-Fe2O3) and low-vacancy Fe2O3 (LV-Fe2O3) were prepared by pulsed laser deposition with a controllable gas atmosphere. XRD patterns confirm that both HV-Fe2O3 and LV-Fe2O3 have a pure phase of hematite (Fig. S1†). Raman spectra (Fig. 1a) of both HV-Fe2O3 and LV-Fe2O3 exhibit seven lines typical for hematite at about 226 (A1g), 245 (Eg), 293 (Eg), 298 (Eg), 412 (Eg), 500 (A1g) and 612 (Eg) cm−1.17 It should be noted that a mode (Eu) at 660 cm−1 appeared in our results which is attributed to the existence of oxygen vacancies in the materials. The peak at 660 cm−1 in HV-Fe2O3 is much more obvious than that in LV-Fe2O3, indicating there are more oxygen vacancies in HV-Fe2O3.18,19 XPS was also used to characterize the surface state of the hematite films. Both Fe2+ and Fe3+ ions can be detected in the XPS spectra of Fe (2p) for HV-Fe2O3 and LV-Fe2O3 as shown in Fig. S2.† Plus, in the XPS spectra for O (1s) (Fig. 1b), peaks at 530.1 eV, 531.8 eV and 532.4 eV commonly correspond to the oxygen present in the lattice, adsorbed OH− and adsorbed water respectively.20–23 It has been previously reported that adsorbed OH− develops with the increasing loss of oxygen.23 From the O (1s) spectra for HV-Fe2O3 and LV-Fe2O3 (Fig. 1b), it is observed that the percentage of adsorbed OH− on oxygen vacancies is 29.5% in LV-Fe2O3, while that in HV-Fe2O3 is 37.9%. The 8.4% higher adsorbed OH− on the surface of HV-Fe2O3 indicates that more oxygen vacancies exist in the samples of HV-Fe2O3.
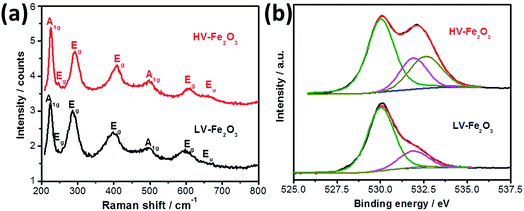 |
| Fig. 1 (a) Raman spectra, (b) XPS spectra of O (1s) of single HV-Fe2O3 and single LV-Fe2O3 films respectively. | |
Meanwhile, absorption spectra for the samples are shown in Fig. 2a. The absorption edges for both HV-Fe2O3 and LV-Fe2O3 are the same, about 590 nm, meaning that the band gaps for the two types of hematite are the same. The absorption after 600 nm in HV-Fe2O3 confirms the existence of oxygen vacancies. A higher amount of oxygen vacancies may increase the donor concentration in the semiconductor. As a result, HV-Fe2O3 is expected to possess larger donor densities. To get further insight into the carrier concentration of both types of hematite films, Mott–Schottky analysis was used.24 The slope for HV-Fe2O3 is smaller as shown in Fig. 2b, indicating that the carrier (electron) concentration in HV-Fe2O3 is higher than in LV-Fe2O3. As HV-Fe2O3 has more carriers, its Fermi level is considered to be nearer to its conduction band and higher than the Fermi level of LV-Fe2O3. From the above results, two types of hematite with different Fermi levels were successfully obtained by controlling the amount of donor densities during the preparation process. In addition, when they connect with each other, a homojunction forms at the interface between them.
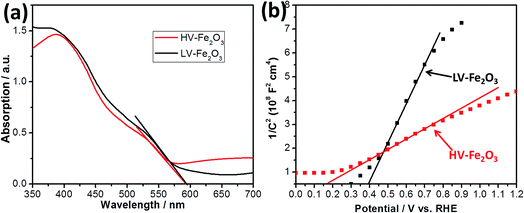 |
| Fig. 2 (a) UV-vis absorption spectra and (b) Mott–Schottky plots at 1 kHz of single HV-Fe2O3 and single LV-Fe2O3 films respectively. | |
The surfaces of HV-Fe2O3 and LV-Fe2O3 were similar, with a flat and smooth morphology (Fig. S3†). As reported in our previous studies, the deposition rates for the Fe based oxide materials were almost the same (3.5 nm min−1),13,17 so the HV-Fe2O3 and LV-Fe2O3 films had nearly the same thickness during the same deposition time as shown in Fig. S4.† The photocurrent density for single HV-Fe2O3 increased gradually with the increasing thickness and it reached the highest density at 70 nm. Then when the thickness exceeded 70 nm, it decreased at a much lower rate due to the increased electron/hole recombination efficiency in the film (Fig. S5†). A similar relationship existed for LV-Fe2O3 between the photocurrent density and the film thickness (Fig. S6†). As a result, both HV-Fe2O3 and LV-Fe2O3 have the highest photocurrent density when the film thickness was about 70 nm according to their linear sweep voltammetry curves. The photocurrent densities at 1.23 V vs. RHE for HV-Fe2O3 and LV-Fe2O3 are summarized in Fig. 3a. HV-Fe2O3 has a little higher photocurrent density compared with LV-Fe2O3. This is due to the higher concentration of carriers existing in HV-Fe2O3 as illustrated in Fig. 1b which offers more electron–hole pairs under light irradiation conditions.
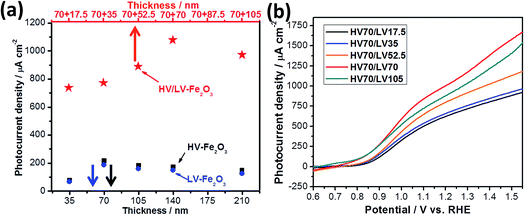 |
| Fig. 3 (a) Comparison of photocurrent densities for single HV-Fe2O3, single LV-Fe2O3 and HV/LV-Fe2O3 homojunctions at 1.23 V vs. RHE respectively. (b) Linear sweep voltammetry curves for HV/LV-Fe2O3 homojunctions with different thicknesses. The thickness of the inner HV-Fe2O3 layer was 70 nm. The thicknesses of the outer LV-Fe2O3 layers were 17.5, 35, 52.5, 70 and 105 nm respectively. The light source is AM 1.5G illumination. | |
PEC performances of hematite homojunction photoanodes (HV/LV-Fe2O3)
The two types of hematite (HV-Fe2O3 and LV-Fe2O3) with different Fermi levels were combined into homojunctions to obtain enhanced PEC performances for hematite. HV-Fe2O3 was firstly prepared on ITO, and LV-Fe2O3 was subsequently deposited on the surface of the HV-Fe2O3. The thickness of the inner HV-Fe2O3 layer was fixed at 70 nm, as it showed the best PEC performance with this thickness. Linear sweep voltammetry curves (LSV) according to the thickness of the outer layer (LV-Fe2O3) were obtained (Fig. 3b) to find the best match of HV-Fe2O3 and LV-Fe2O3. The onset potential was the lowest when the thickness of the LV-Fe2O3 reached about 70 nm. The photocurrent densities with different thicknesses of the hematite homojunction photoanodes (HV/LV-Fe2O3) are summarized in Fig. 3a (star points). It is clear that when the thicknesses of the homojunction photoanodes reached 140 nm (70 nm for the HV-Fe2O3 layer and 70 nm for the LV-Fe2O3 layer), the photoanodes performed with the highest photocurrent densities. As a result, the best design for the hematite homojunctions was fixed at 70 nm for the inside HV-Fe2O3 layer and 70 nm for the outside LV-Fe2O3 layer. The cross view for the best HV/LV-Fe2O3 homojunction photoanode is shown in Fig. 4a while the inset in Fig. 4a is a picture illustration for the structure of HV/LV-Fe2O3 homojunction photoanodes. The surface morphology investigated by Atomic Force Microscopy (AFM) is shown in Fig. 4b. The roughness was about 4.6 nm, indicating that the obtained photoanodes had a quite flat and smooth morphology, which was similar in both the single HV-Fe2O3 and single LV-Fe2O3 films (Fig. S3†). Raman spectra of the HV/LV-Fe2O3 homojunction (Fig. S7a†) show that the homojunction is composed of pure hematite with a small amount of oxygen vacancies observed (Eu mode). The UV-vis absorption (Fig. S7b†) is a little stronger than the single layer hematite film with a slight absorption after 600 nm which is attributed to the existence of oxygen vacancies.
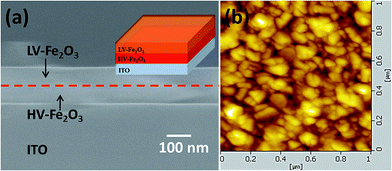 |
| Fig. 4 (a) SEM for the cross view of HV/LV-Fe2O3 homojunction photoanodes. (b) AFM for the top view of HV/LV-Fe2O3 homojunction photoanodes. | |
A comparison of PEC performances for single HV-Fe2O3, single LV-Fe2O3 and HV/LV-Fe2O3 homojunction photoanodes are shown in Fig. 5. The onset potential for single HV-Fe2O3 and LV-Fe2O3 was relatively high, about 1.08 V vs. RHE, while that of HV/LV-Fe2O3 was only 0.86 V vs. RHE (Fig. 5a). The detailed photocurrent density at 1.23 V vs. RHE is illustrated in Fig. 5b. The photocurrent densities for single HV-Fe2O3 and LV-Fe2O3 were 217 μA cm−2 and 189 μA cm−2 respectively. Interestingly, the photocurrent density for HV/LV-Fe2O3 was ∼1070 μA cm−2, which was about 5 times that of single HV-Fe2O3 and LV-Fe2O3. The improvement of the photocurrent density is much more obvious for the as-prepared HV/LV-Fe2O3 homojunction (∼5 times improvement) than the previously reported Mg–Fe2O3/Fe2O3 p–n junction (1.3 times improvement).26 Besides, the photocurrent density at 1.23 V vs. RHE for the HV/LV-Fe2O3 homojunction is ∼2 times that of the Mg–Fe2O3/Fe2O3 homojunction, indicating that the hematite homojunction formed by oxygen vacancy control is more effective than that formed by doping with Mg element.
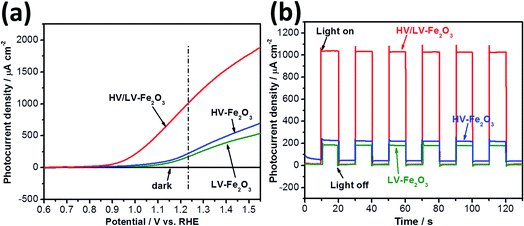 |
| Fig. 5 (a) Linear sweep voltammetry curves, (b) current–time curves for single HV-Fe2O3 layer, single LV-Fe2O3 and HV/LV-Fe2O3 homojunction photoanodes respectively. | |
Mechanism discussion
The greatly improved photocurrent density confirms that a homojunction really exists in the HV/LV-Fe2O3 photoanodes. To gain further insight into the charge-transfer process, electrochemical impedance spectra (EIS) were collected at 1.23 V vs. RHE in 1 M NaOH solution under light illumination (Fig. 6a).14 The radius of the arcs of the Nyquist plots for the HV/LV-Fe2O3 homojunctions was much smaller than both the single HV-Fe2O3 and LV-Fe2O3, indicating that the HV/LV-Fe2O3 homojunctions had a higher charge transfer efficiency under light illumination. Besides, open circuit photovoltage (OCP) curves of the photoanodes in the dark and under AM 1.5G illumination were measured in the same solution as shown in Fig. 6b. A nearly two-times larger OCP is observed for the HV/LV-Fe2O3 homojunction sample than the single type of hematite, indicating an enlarged band bending at the photoanode/electrolyte interface, which represents the enhanced electron–hole separation.25
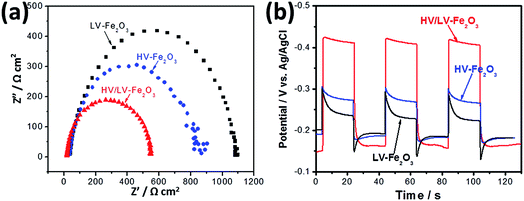 |
| Fig. 6 (a) Electrochemical impedance spectra curves and (b) open circuit potentials for single HV-Fe2O3 layer, single LV-Fe2O3 and HV/LV-Fe2O3 homojunction photoanodes respectively. | |
The energy diagrams of single HV-Fe2O3, single LV-Fe2O3 and the HV/LV-Fe2O3 homojunction are shown in Fig. 7 as well as the expected charge flow of the homojunction photoanode. Since the HV-Fe2O3 and LV-Fe2O3 had the same phases (Fig. S1† and 1a) and band gaps (Fig. 2a), it is considered that the positions of their conduction band and valence band were the same before contact. As the density of oxygen vacancies in HV-Fe2O3 was higher than that in LV-Fe2O3, the carrier concentration in these two types of hematite was different as well as the Fermi level positions. HV-Fe2O3 with a higher oxygen vacancy density (Fig. 2b) possessed a higher Fermi level position while LV-Fe2O3 with a lower oxygen vacancy density possessed a lower Fermi level position. When the two types of hematite contacted with each other, a homojunction was formed with an electric field between the interfaces. Under the influence of the electric field, holes were transferred from HV-Fe2O3 to LV-Fe2O3 and were consumed in the water oxidation reaction (Fig. S10†). At the same time, electrons were transferred from LV-Fe2O3 to HV-Fe2O3 under the influence of the built-in electric field at the homojunction, and then were transferred to the Pt cathode through the circuit for water reduction. Therefore, more holes will be accumulated on the surface of LV-Fe2O3 and be involved in the water oxidation reaction due to the electronic field formed at the homojunction. The homojunction made the charge transfer efficiency (Fig. 6a) and charge separation efficiency (Fig. 6b) in the photoanodes much higher than the single type of hematite and a dramatically enhanced photocurrent density occurred as shown in the current–time curves (Fig. 5b). Besides, as the holes were mainly accumulated on the surface of the LV-Fe2O3, the inner layer with high oxygen vacancy can be stabilized and protected by the outer layer with low oxygen vacancy during the anodic oxidation for oxygen evolution at the semiconductor/liquid interface.
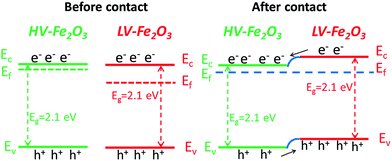 |
| Fig. 7 Energy diagram of single HV-Fe2O3, single LV-Fe2O3 and HV/LV-Fe2O3 homojunction photoanodes respectively and the speculated charge flow in the homojunction photoanode. | |
Incident photo-to-current efficiency (IPCE) (Fig. S8†) was used to determine the light usage efficiency of the HV/LV-Fe2O3 homojunction photoanodes. It is observed that light with a wavelength below 600 nm can make some contribution to the photocurrent density according to the IPCE curve. The IPCE increased gradually as light wavelength decreased and it reached the highest efficiency at 400 nm of about 29.0%.
Long-time water splitting performance of hematite homojunction photoanodes (HV/LV-Fe2O3)
Long-time water splitting experiments were carried out using air mass 1.5G (AM 1.5G) as the light source with a 1.23 V vs. RHE (Fig. 8a) external bias in 1 M NaOH solution. The corresponding current–time curves are shown in Fig. 8b. The dashed line in Fig. 8a is the theoretical amount of oxygen gas calculated from the current–time curves. It is observed that the dashed line is almost the same as the oxygen evolution dots, indicating that the anodic photocurrent generated by the HV/LV-Fe2O3 homojunction photoanode is the exclusive result of O2 evolution from water splitting. It should be noted that although there are a number of reports about improving PEC water splitting performances by increasing the density of oxygen vacancies in semiconductors,27–31 the function of these oxygen vacancies are mainly to influence the light absorption properties of the materials.28 Oxygen vacancies in most of the literature are generally produced near to the surface since they are generated by a reductive post-treatment process after the formation of the photoelectrodes.27,29 As a result, they are very unstable and readily consumed during the anodic oxidation at the semiconductor/liquid interface.27 Finally, water splitting is generally observed with oxygen vacancy consumption with an oxygen evolution rate less than half that of hydrogen.28 However, in our work, the ratio of the H2 and O2 evolution rates was nearly 2
:
1, implying that the oxygen vacancies were not involved in the water oxidation reaction and overall water splitting occurred in this HV/LV-Fe2O3–Pt PEC system. Moreover, the HV/LV-Fe2O3 homojunction photoanode (Fig. 8b) showed a 15 times higher stability compared with single HV-Fe2O3 (Fig. S9†). This also confirmed that the inner HV-Fe2O3 is successfully protected by the homojunction and the outer LV-Fe2O3. Water splitting rates in this system were very high, which were about 22.9 μmol h−1 for H2 evolution and 12.6 μmol h−1 for O2 evolution respectively. A faradaic efficiency of nearly 100% was achieved by comparing the ideal oxygen evolution curve (dashed line) and the actual oxygen evolution curve. The gas evolution amounts for HV/LV-Fe2O3 increased almost linearly with time, accumulating for 73 hours, and the photocurrent still held 92.2% of the reaction rates even after 73 hours water splitting.
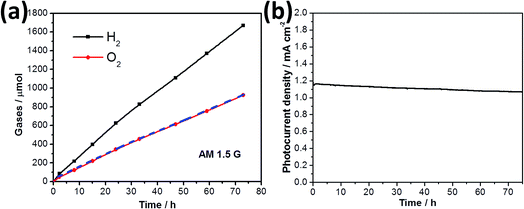 |
| Fig. 8 Time courses of gas evolution (a) and the corresponding current–time curve (b) for HV/LV-Fe2O3 homojunction photoanodes. The dashed line in (a) is the oxygen gas calculated from the current–time curves. | |
Conclusion
In summary, we developed a new strategy to prepare hematite homojunction photoanodes. By controlling the density of oxygen vacancies in hematite, two types of hematite films with different Fermi levels were obtained. Hematite homojunction photoanodes, HV/LV-Fe2O3, were successfully fabricated after combining the two types of hematite together. The hematite homojunction photoanode showed several times higher water splitting efficiency and stability. Most importantly, it still held 92.2% of the efficiency for water oxidation even after 73 hours. We believe that the method to tune the Fermi level of semiconductor films by controlling the oxygen vacancies can be extended to other metal oxide semiconductors such as TiO2 and ZnO et al., and that the formation of homojunction photoelectrodes within these metal oxide semiconductors will offer new opportunities to improve PEC water splitting efficiency.
Acknowledgements
This work received financial support from the World Premier International Research Center Initiative on Materials Nanoarchitectonics (WPI MANA), the Japanese Government (Monbukagakusho: MEXT) Scholarship, the National Basic Research Program of China (973 Program, 2014CB239301), and the Mitsubishi Foundation.
References
- H. Tong, S. Ouyang, Y. Bi, N. Umezawa, M. Oshikiri and J. Ye, Adv. Mater., 2012, 24, 229 CrossRef CAS PubMed
. - X. Meng, T. Wang, L. Liu, S. Ouyang, P. Li, H. Hu, T. Kako, H. Iwai, A. Tanaka and J. Ye, Angew. Chem., Int. Ed., 2014, 126, 11662 CrossRef
. - K. Chang, M. Li, T. Wang, S. Ouyang, P. Li, L. Liu and J. Ye, Adv. Energy Mater., 2015 DOI:10.1002/aenm.201402279
. - Z. Li, W. Luo, M. Zhang, J. Feng and Z. Zou, Energy Environ. Sci., 2013, 6, 347 Search PubMed
; J. Cao, J. Xing, Y. Zhang, H. Tong, Y. Bi, T. Kako, M. Takeguchi and J. Ye, Langmuir, 2013, 29, 3116 CrossRef CAS PubMed
. - S. C. Warren, K. Voitchovsky, H. Dotan, C. M. Leroy, M. Cornuz, F. Stellacci, C. Hebert, A. Rothschild and M. Grätzel, Nat. Mater., 2013, 12, 842 CrossRef CAS PubMed
; J. Cao, T. Kako, P. Li, S. Ouyang and J. Ye, Electrochem. Commun., 2011, 13, 275 CrossRef
. - I. Justicia, P. Ordejon, G. Canto, J. Mozos, L. J. Fraxedas, G. A. Battiston, R. Gerbasi and A. Figueras, Adv. Mater., 2002, 14, 1399 CrossRef CAS
. - S. Polarz, J. Strunk, V. Ischenko, M. W. E. van den Berg, O. Hinrichsen, M. Muhler and M. Driess, Angew. Chem., Int. Ed., 2006, 45, 2965 CrossRef CAS PubMed
. - R. Schaub, P. Thostrup, N. Lopez, E. Lægsgaard, I. Stensgaard, J. K. Nørskov and F. Besenbacher, Phys. Rev. Lett., 2001, 87, 266104 CrossRef CAS PubMed
. - I. S. Cho, M. C. Logar, H. Lee, L. Cai, F. B. Prinz and X. Zheng, Nano Lett., 2014, 14, 24 CrossRef CAS PubMed
; J. Cao, Y. Zhang, L. Liu and J. Ye, Chem. Commun., 2013, 49, 3440 RSC
. - H. Li, Y. Zhou, W. Tu, J. Ye and Z. Zou, Adv. Funct. Mater., 2015, 25, 998 CrossRef CAS
. - S. W. Boettcher, E. L. Warren, M. C. Putnam, E. A. Santori, D. Turner-Evans, M. D. Kelzenberg, M. G. Walter, J. R. McKone, B. S. Brunschwig, H. A. Atwater and N. S. Lewis, J. Am. Chem. Soc., 2011, 133, 1216 CrossRef CAS PubMed
. - F. F. Abdi, L. H. Han, A. H. M. Smets, M. Zeman, B. Dam and R. vande Krol, Nat. Commun., 2013, 4, 2195 Search PubMed
. - Q. Yu, X. Meng, T. Wang, P. Li, L. Liu, K. Chang, G. Liu and J. Ye, Chem. Commun., 2015, 51, 3630 RSC
; J. Cao, L. Liu, A. Hashimoto and J. Ye, Electrochem. Commun., 2014, 48, 17 CrossRef CAS
. - Q. Yu, X. Meng, T. Wang, P. Li and J. Ye, Adv. Funct. Mater., 2015, 25, 2686 CrossRef CAS
. - Y. W. Li, Z. G. Hu, F. Y. Yue, P. X. Yang, Y. N. Qian, W. J. Cheng, X. M. Ma and J. H. Chu, J. Phys. D: Appl. Phys., 2008, 41, 215403 CrossRef
. - H. Lin, A. Rumaiz, K. Schulz, M. D. Wang, R. C. Rock, P. Huang and S. I. Shah, Mater. Sci. Eng., B, 2008, 151, 133 CrossRef CAS
. - J. Cao, T. Kako, N. Kikugawa and J. Ye, J. Phys. D: Appl. Phys., 2010, 43, 325101 CrossRef
. - I. V. Chernyshova, A. S. Hochella Jr and M. F. Madden, Phys. Chem. Chem. Phys., 2007, 9, 1736 RSC
. - N. D. Phu, D. T. Ngo, L. H. Hoang, N. H. Luong, N. Chau and N. H. Hai, J. Phys. D: Appl. Phys., 2011, 44, 345002 CrossRef
. - J. Gan, X. Lu, J. Wu, S. Xie, T. Zhai, M. Yu, Z. Zhang, Y. Mao, S. Wang, Y. Shen and Y. Tong, Sci. Rep., 2013, 3, 1021 CrossRef PubMed
. - X. Lu, Y. Zeng, M. Yu, T. Zhai, C. Liang, S. Xie, M. Balogun and Y. Tong, Adv. Mater., 2014, 26, 3148 CrossRef CAS PubMed
. - C. D. Wagner, in Practical Surface Analysis, Wiley, Chichester, 1990 Search PubMed
. - G. Tyuliev and S. Angelov, Appl. Surf. Sci., 1988, 32, 387 CrossRef
. - M. Grätzel, Nature, 2001, 414, 338 CrossRef PubMed
. - M. Zhong, T. Hisatomi, Y. Kuang, J. Zhao, M. Liu, A. Iwase, Q. Jia, H. Nishiyama, T. Minegishi, M. Nakabayashi, N. Shibata, R. Niishiro, C. Katayama, H. Shibano, M. Katayama, A. Kudo, T. Yamada and K. Domen, J. Am. Chem. Soc., 2015, 137, 5053 CrossRef CAS PubMed
. - Y. Lin, Y. Xu, M. T. Mayer, Z. I. Simpson, G. McMahon, S. Zhou and D. Wang, J. Am. Chem. Soc., 2012, 134, 5508 CrossRef CAS PubMed
. - C. Mao, F. Zuo, Y. Hou, X. Bu and P. Feng, Angew. Chem., Int. Ed., 2014, 53, 10485 CrossRef CAS PubMed
. - X. Chen, L. Liu, P. Y. Yu and S. S. Mao, Science, 2011, 331, 746 CrossRef CAS PubMed
. - G. Wang, H. Wang, Y. Ling, Y. Tang, X. Yang, R. C. Fitzmorris, C. Wang, J. Z. Zhang and Y. Li, Nano Lett., 2011, 11, 3026 CrossRef CAS PubMed
. - Q. Kang, J. Cao, Y. Zhang, L. Liu, H. Xu and J. Ye, J. Mater. Chem. A, 2013, 1, 5766 RSC
. - F. L. Wang, T. Wu, Z. Zhang, D. Borchardt and P. Feng, J. Am. Chem. Soc., 2010, 132, 11856 CrossRef PubMed
.
Footnote |
† Electronic supplementary information (ESI) available. See DOI: 10.1039/c6ra13060g |
|
This journal is © The Royal Society of Chemistry 2016 |