DOI:
10.1039/C6RA12480A
(Paper)
RSC Adv., 2016,
6, 62825-62832
Design of a hierarchically structured hybrid material via in situ assembly of a silica aerogel into a wood cellular structure†
Received
13th May 2016
, Accepted 23rd June 2016
First published on 27th June 2016
Abstract
A novel hierarchically structured wood-templated silica aerogel hybrid material was synthesized through sol impregnation and in situ gelation within a Norway spruce wood scaffold. Following chemical modification and supercritical drying of the microstructured gel phase, the desired hybrid wood–aerogel composite was obtained. The developed method presents a new route for efficient incorporation of highly mesoporous low-density silica aerogel into cellular biomaterial structures without the need for pretreatments to access the complex cellular level network. In-depth characterization using microtomography and electron microscopy revealed that the mesoporous silica aerogel filled the wood lumina to a very large extent, whereas no silica was deposited inside the wood cell walls. The incorporation of the hydrophobic aerogel substructure within the wood microstructure resulted in an improved dimensional stability and reduced moisture retention of the hybrid material which may lead to improved durability at higher humidity. Furthermore, the super-insulating silica aerogel phase leads to lower thermal conductivity and total heat of combustion. This study is viewed as a proof of concept for the in situ preparation of natural bio template based hybrid aerogels, and will enable a new generation of multifunctional bioinspired materials with tailored physical properties and applications.
Introduction
Wood is a material of great historical and trend-setting significance, when sustainability, aesthetic and engineering properties such as high stiffness to weight ratio, vibro-acoustic properties and climate impact are considered. Wood based materials are widely used in interior architecture, construction, art and restoration, due to comfort and psychological perception1 as well as engineering related properties. At a material level, wood is a hierarchically structured cellular material, where a finely tuned nature-made architecture of biopolymer building blocks within the substructure of layered cell walls and their middle lamella determines the bulk properties and outstanding ability to stand internal and external forces.2 This unique, hierarchical architecture has been developed across multiple length scales, from bulk of the material to cellulose semi-crystalline microfibrils, while the morphological and compositional parameters determine the density, moisture uptake as well as thermal and mechanical properties of the material.3 Wood can be considered hybrid, where the cellulose semi-crystalline microfibrils represent the reinforcing media and are responsible for stiffness, in a matrix of hemicelluloses and lignin which contributes more in the viscoelasticity. Microfibrils and matrix are linked by hydrogen bonds and covalent chemical crosslinks, which are dictating the hygroscopic nature and dimensional instability of wood.4 From engineering perspective, dimensional instability, limited durability and poor fire behaviour are its most prominent shortcomings, which negatively influence reliability and its usage in building and construction sectors.
Traditional wood modification strategies employ various treatments with chemical additives, which most commonly target the alteration of volume fraction as well as degree and chemistry of crosslinking of the biopolymers within the bulk of the material. In other words, the modification of physical or mechanical properties of bulk wood is typically identified as (i) the controlled removal of biopolymer from the cell walls or (ii) incorporation of organic or inorganic compounds into the cell lumina or the layered cell wall structure. In general, the complexity of the partly closed pore structure of wood limits easy access of chemical reagents deep inside the material and makes its modification inherently challenging. One prominent example of controlled degradation of the biopolymers that make up the cell walls are chemical or fungal treatments which can be employed to alter permeability or vibro-mechanical properties of wood materials.5,6 Chemical modification methods typically rely on forming a covalent bond to the hydroxyl groups of the carbohydrate backbone or cross-links between hydroxyl groups to reduce polarity and thus moisture sorption properties.7–9 Ermeydan and coworkers have demonstrated a chemical modification of wood through polymerisation of monomers within the wood microstructure to improve its dimensional stability and hardness.10 In a similar manner, incorporation of metal oxides, borate and phosphorous compounds into the cell walls can lead to improved fire resistance and mechanical properties.11–16 However, due to difficulty in accessing the wood's inner structure by liquid chemical agents and their potential environmental emission hazards in case of fire exposure, up-scaling of wood modification strategies is becoming an active area of technical development worldwide.
In this work, we demonstrate the concept of in situ synthesis of silica aerogel inside the cellular microstructure of wood using Norway spruce as a bio-template. Silica aerogels are high surface area mesoporous low density materials with a typical nanoscale morphology consisting of a “pearl necklace”-like hierarchical assembly of silica nanoparticles in an open porous 3D particle network. Each “pearl” consists itself of a number of smaller, colloidal primary particle building blocks of single digit nanometer diameter. This unique hierarchical structure sets the tone for the material's outstanding physical properties, such as low bulk densities (typically 0.05 to 0.3 g cm−3),17 high porosity (>90%), tailorable surface chemistry and low thermal conductivity and flammability,1–21 making it one of the most promising high-performance insulation materials existing today. Silica aerogels show specific acoustic properties, categorized by low reflection and high sound absorption,22 especially at low frequencies, where the traditional insulation materials show significant flaws.23
Part of the motivation for this work is in fact the rather ambitious objective to improve particularly acoustic, thermal and fire-related properties of wood, by incorporation of functional materials such as silica aerogel inside the lumens, while preserving its aesthetic appearance and high stiffness-to-weight ratio. Recently, silica aerogels in combination with cellulosic scaffolds at nano and micro scale have been used to synthesize high-performance insulation materials with a view on next generation building and engineering applications.24–26 Although studies on wood modification (coating of cell walls with “nano-SiO2”) with colloidal silica materials are well known,16 so far a hybrid material made by a true volume templating of silica aerogel within the wood cell structure has not yet been reported. Existing concepts deal rather with the cell wall modification of wood-based bio scaffolds with silica, where neither hydrophobization nor supercritical drying are employed which are essential to produce a highly mesoporous, hydrophobic, durable silica aerogel substructure.16,27
In this work, we describe a novel approach to synthesise a wood templated silica aerogel hybrid material, starting by impregnation of wood lumens with a low-viscosity, tetraethyl orthosilicate derived silica sol in an ethanol/water solvent system. In-depth impregnation of the wood's cellular structure with the sol was facilitated using vacuum, followed by exposure to ammonia in the gas phase which catalyzes the gelation process. Aged wood/gel composites were hydrophobized using standard solvent exchange/hydrophobization chemistry and the resulting materials dried from supercritical CO2 to yield the final aerogel hybrid. Norway spruce (Picea abies) was used as a reference wood template as it is amongst the most commonly used wood species in construction applications and widely studied scientifically. As a softwood species with fine and partially closed pore structure, it is a difficult substrate to impregnate. In this work, we give proof of concept for aerogel volume templating with emphasis on quantifying the distribution and cell lumen filling extent as well as its homogeneity. Experimental results such as water uptake, dimensional stability and selected thermal and vibro-mechanical properties are discussed in the context of potential application strategies.
Experimental
Material synthesis
Wood specimens. Specimens without visible macroscopic defects were selected from Norway spruce. Twin block specimens of 40 × 10 × 40 mm3 (R: radial × T: tangential × L: longitudinal) were prepared for thermal analysis and strips of 120 (R) × 12 (T) × 2.5 (L) mm3 and 100 (R) × 5 (T) × 150 (L) mm3 for vibro-mechanical tests which were conducted parallel and perpendicular to the grain.
Wood–silica aerogel hybrid synthesis. A pre-polymerized tetraethyl orthosilicate (TEOS) containing 20% w/w SiO2 in ethanol (PEDS-P750E20, PCAS, France) was used as the silica gel precursor. The silica sols were further diluted with ethanol (F25-AF-MEK ethanol denatured with 2% methyl ethyl ketone) to a SiO2 concentration of 6% by weight. In Fig. 1, the processing steps for the preparation of the hybrid materials are schematically presented.
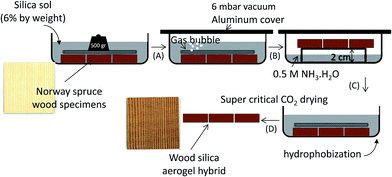 |
| Fig. 1 Schematic presentation of the different steps for synthesis of wood silica aerogel hybrid. | |
First, oven dried (80 °C for 48 h) Norway spruce specimens were immersed into the diluted silica sol, covered with aluminium foil and placed in a vacuum chamber for 30 min at 25 °C (step_A). After 5 minutes, once the target vacuum level of 60 mbar was reached the vacuum pump was switched off. The vacuum impregnated specimens were removed from the sol and placed on top of a small platform which had been placed inside a small container, containing 0.5 M NH3·H2O solution (step_B). After sealing, this container was placed in an oven at 65 °C for 24 h, allowing for the slow release of the NH3 gas from the solution and absorption by the sol-impregnated specimen enabling homogeneous and controlled gelation. The samples were then kept at room temperature and allowed to age overnight. The resulting hybrid gel was first exchanged with ethanol and then hydrophobized in a hexamethyldisiloxane (HMDSO)/hydrochloric acid (HCl, 37%)/ethanol solution for 24 hours at 65 °C (step_C). The final silica aerogel hybrid was obtained after supercritical drying in CO2 in a Supercritical Fluid Extractor (Autoclave 4334/A21–1, Separex, France) (step_D). In addition to the synthesized hybrid wood material, a set of ‘reference’ samples were prepared by hydrophobization in hexamethyldisiloxane (HMDSO)/hydrochloric acid (HCl, 37%)/ethanol solution, but without the impregnation step with the silica sol and the gelation steps (A, B).
Structure and property characterization
Chemical analysis. Small specimens of the hydrophobized “reference” material were analysed by total hydrolysis and compared to the untreated wood to evaluate whether the hydrophobization procedure degraded the wood biopolymers. For this purpose, triplicate specimens were hydrolysed in sulphuric acid, the hydrolysed carbohydrates were separated by borate complex ion exchange chromatography, and detected photometrically with copper-2,2-bicinchoninate reagent.28
Electron microscopy. Scanning Electron Microscopy (SEM) analysis was performed using a FEI Nova NanoSEM 230 instrument (FEI, Hillsboro, OR) at an accelerating voltage of 5 kV and a working distance of 5 mm. Specimens of approximately 5 × 5 × 5 mm3 (R × T × L) were fixed to the sample holder using a carbon adhesive tape and a 5 nm platinum sputter coated. Also, transmission electron microscopy (TEM) images were recorded with a JEOL-2200FS microscope operated at an accelerating voltage of 200 kV. Care has been taken to avoid beam-induced modification of the aerogel morphology by using short exposure times with a low electron dose.
Wavelength-dispersive X-ray spectroscopy (WDX). Measurements were performed with a scanning electron microscope (JEOL, JXA-8800RL), equipped with a wave-dispersive X-ray detector. Silicon was detected at an accelerating voltage of 15 kV and an illuminating current of 2 × 10−8 A. Si element mapping was performed on a selected area of 300 × 300 μm2 with a spatial resolution of 2 μm.
Synchrotron X-ray microtomography. Synchrotron based X-ray tomographic microscopy at the TOMCAT (TOmographic Microscopy and Coherent rAdiology experimenTs) beamline of the Swiss Light Source29 was used to probe the microstructure of wood–silica aerogel hybrids. The beamline is based on a 2.9 T bending magnet with a cut-off X-ray photon energy of 11.1 keV, while the range of used energy can be selected by means of a multilayer monochromator. To reduce phase artefacts and increase signal to noise, simultaneous phase and amplitude retrieval algorithm was implemented for tomographic reconstruction.30 Each reconstructed tomographic dataset consisted of 2160 RT cross-sectional slices with an isotropic voxel size of 0.65 × 0.65 × 0.65 μm3.
Vibro-mechanics. A resonance flexural vibration test setup was used to determine the first resonance frequency (fR) and internal friction (tan
δ) of the strip specimens of the different specimens (untreated, hydrophobized reference and silica aerogel wood hybrid materials). Vibrational emission and data acquisition were carried out using a custom-developed algorithm, according to a previously published procedure.31 In this setup, the material stiffness is calculated using Euler–Bernoulli equation, while the logarithmic decrement of amplitudes in time domain is recorded and compared to the damping in frequency domain. Of each sample type, 3 to 5 identically prepared specimens were analysed.
Moisture, heat transfer and calorimetric analysis. As a measure of dimensional stability, swelling of oven-dried (24 hours at 80 °C) block specimens of dimensions 40 × 10 × 40 mm3 (R × T × L) was measured with a calliper after conditioning at 80% relative humidity (RH). Also, moisture sorption of the hybrid material was characterized by means of dynamic vapour sorption instrument (DVS, TA instruments, Germany) and the results were compared with the sorption isotherms of untreated and hydrophobized reference specimens. During the measurement, each specimen of around 5 mg weight was equilibrated at eight RH steps in adsorption, spanning a range from 15 to 95% RH, at a constant temperature of 25 °C and the same for the desorption step. During each step, the time dependent change in the mass of specimen until quasi-equilibrium condition (the time at which the change in mass per minute was less than 0.001% over a 5 min period) was recorded. In addition to the full adsorption–desorption isotherms, we also recorded the transient data for a step RH change from 30 to 90% and 90 to 30%.The total heat of combustion of the wood specimen was measured using a pyrolysis combustion flow calorimeter (PCFC, Fire Testing Technology UK). Untreated, hybrid and hydrophobized reference specimens were heated in an inert atmosphere of nitrogen from 40 to 800 °C at a heating rate of 1 °C s−1 and the heat release rates of decomposing samples were measured as a function of time. The total heat of combustion was calculated by integration of area under total heat release rate and time of experiment. The thermal conductivity of the block specimens were also measured at 50% RH and 25 °C, using a custom built guarded hotplate device.32 For each of the analyses, 3 to 5 replications per specimen were performed.
Results and discussions
Fig. 2 presents the structural hierarchy of the multi-scale hybrid material. In wood and plant tissues, the hierarchy and distribution of building blocks define the way that plant manages to carry and distribute the internal and external stresses. This also determines the physical properties such as moisture uptake and thermal conductivity. In the hybrid material, the lumens of wood are filled with a hierarchical assembly of silica nanoparticles, as their pearl like structure is observed by TEM. Due to the extremely different sorptivity and thermal properties of silica aerogel, the physical properties of the hybrid, depending on the extent of coverage of the lumens with silica aerogel, are expected to be different from untreated wood. SEM and X-ray tomographic analysis confirm the successful impregnation of the silica aerogel phase inside the cell lumens and the formation of a hierarchical structure. In the 3D rendered tomograms of the wood hybrid in Fig. 3a, the skeletal features of the wood scaffold, e.g. longitudinal growth cells in spring (earlywood) and late summer/autumn (latewood) are highlighted in grey while the mesoporous silica aerogel inside the cell lumina is segmented in colour. The red to yellow colouring corresponds to the higher attenuation of X-ray in the optically denser silica aerogel phase. Tomography confirms a high and reproducible degree of filling in both early and latewood cells. Efficient filling of the fine cell cavities of Norway spruce with the silica aerogel is also observed in the SEM longitudinal cross section in Fig. 3b, and further supported by an independent measurement of 4.3% (±0.7%) increase in dry mass of the hybrid material which agrees with the predicted values. In Fig. 3c, cross sections of untreated wood (top) and the silica aerogel hybrid (bottom) are shown. The picture inset in Fig. 3c shows a higher magnification SEM image of the silica aerogel phase, displaying the expected highly-mesoporous particle network structure of the aerogel filler. WDX Si maps reveal that the deposition of silica is restricted to the lumens and doesn't deposit in the wood cell walls (Fig. 3d). This is in fact expected, since the pre-polymerized TEOS based sol already consists of nanometre sized colloidal particles which are too big to enter the rather densified wood cell wall structure. SEM images of the treated samples further reveal the presence of occasional, discretely distributed microcracks, typically at the cell wall interface. These microcracks can be found primarily in the hydrophobized reference (see Fig. 4a) and the hybrid materials. There are two possible explanations for the occurrence of microcracks, namely (i) sample preparation defects which might occur during slicing with diamond knife for microscopy and (ii) a weakening of the wood matrix microstructure through chemical attack as a result of extraction of wood biopolymers during the acid catalyzed hydrophobization procedure. Experimental evidence for hypothesized weakening effect can be found through chemical analysis, as presented in Table 1: for the reference material, the respective content of hemicellulose and lignin in the analysed extracts decrease notably during hydrophobization, while the total cellulose content remains more or less unchanged. Nevertheless, the overall mass loss after hydrophobization appears rather insignificant, indicating only a minor loss of hemicellulose and lignin and/or at least a partial compensation of the lost mass by the silylation treatment.
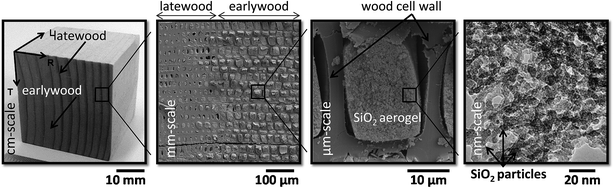 |
| Fig. 2 Geometrical hierarchy in the structure of hybrid across different length scales: cm scale in hybrid, mm-scale in growth rings, μm-scale in lumen and cell wall and nm in SiO2 particles. | |
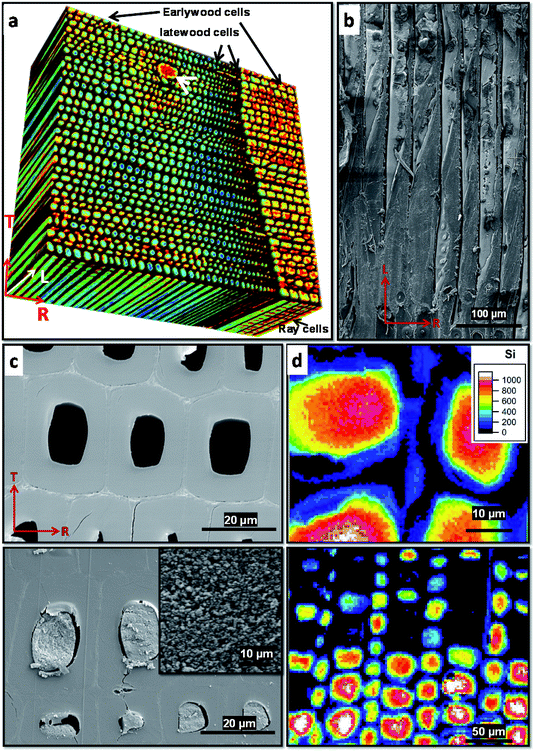 |
| Fig. 3 Microstructure analysis of wood–silica aerogel hybrids featuring, (a) 3D rendered X-ray microtomography, showing the rays, earlywood, latewood cells and a filled vessel with aerogel in grey, while different colours of segmented aerogel represent the slight differences in its density. (b) SEM longitudinal view inside the cell lumina. (c) SEM cross-sectional view before and after aerogel incorporation. (d) Wavelength dispersive X-ray analysis at two different magnifications showing direct evidence of silica aerogel forming exclusively inside the cell lumina. | |
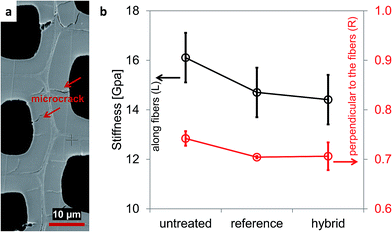 |
| Fig. 4 (a) SEM cross-section of a hydrophobized “reference” wood sample along the RT plane showing occasional microcracks. (b) Resulting decrease in the stiffness of hydrophobized reference material and hybrid, along (right axis) and perpendicular (left axis) to the cell lumen direction. | |
Table 1 Change of the volume fraction of main wood components, determined by sugar analysis of untreated and hydrophobized reference specimens (average of 3 analysed samples per specimen)
Constituent [%] |
Untreated wood |
Hydrophobized reference |
Cellulose |
46.10 (±0.12) |
46.91 (±0.45) |
Hemicellulose |
24.50 (±0.19) |
22.66 (±0.27) |
Lignin and extractives |
26.50 (±0.33) |
25.57 (±0.53) |
As the degradation of cell walls may affect the mechanical performance of the material, stiffness of the untreated, hydrophobized and hybrid specimens were compared. Fig. 4b shows that the stiffness of both hydrophobized and hybrid materials is 5–9% lower than the untreated wood, in both longitudinal and transversal directions. Reduction in the mechanical properties of the hydrophobized and hybrid material is related to the partial degradation of biopolymer matrix, thus further aggravating the innate structural heterogeneities within the wood microstructure. As expected, the reinforcement with silica aerogel fillers with extremely low stiffness in the 1 to 10 kPa range seems to have little influence on the mechanical properties of the hybrid material.
A more significant effect is observed in the moisture uptake of the hybrid material, particularly in terms of the respective sorption rates. In Fig. 5a and b, the normalized moisture uptake rates in ad- and desorption mode respectively are presented. For relative humidity step changes from 30 to 90% RH and 90 to 30% RH, the aerogel hybrid wood show only minor differences compared to the untreated wood. The response onset in the transient adsorption curves in untreated, reference and hybrid wood specimens occurs after 3.7 minutes change of the humidity and for the pure silica aerogel, after only 2 minutes. This delay time is linked to the diffusion of gaseous water and the onset of capillary condensation in the large and accessible macropores (cell lumina) in wood. After the peak, the difference in the slope of sorption curves suggests the water transport in the untreated wood is slower than in the hybrid and reference material. This phase represents the earlier stage of bulk uptake of water by the material, i.e. the process of water transport through the cell walls which lasts for hours until moisture equilibration is reached.33 The slope of the curve is steeper (faster kinetics) in the hybrid and pure silica aerogel, compared to the reference material. Similarly, faster moisture transport of the reference and hybrid specimens compared to the untreated wood was observed in the desorption branch (Fig. 5c). Faster water vapour transport in the hybrid specimen is attributed to the solvent treatment and processing which may create additional cracks/interfaces that increase transport properties. The moisture sorption isotherms of the untreated, hydrophobized reference and hybrid wood show a typical hysteresis behaviour, less moisture uptake at a given RH point in the adsorption than in the desorption branch, as presented in Fig. 5c. Hybrid and hydrophobized reference specimens show very similar isotherms and roughly 5% less moisture uptake than the untreated Norway spruce. This difference is attributed primarily to a partial hydrophobization of the inner cell walls during the hydrophobization (reference treatment) step. Additionally, the acid catalysed hydrophobization process may cause a reduction in hemicellulose content as previously discussed (Table 1), which may also lower the moisture uptake in the cell walls. However, sorption isotherms show no evidence of an alteration in moisture retention capacity at equilibrium, to be directly linked to the formation of an aerogel phase inside the cellular structure of the scaffold, e.g. in wood lumina and pits. This suggests that the hydrophobization treatment is the primarily responsible for the difference in the observed transient data as a result of chemical functionalization of the inner lumen cell wall surface.
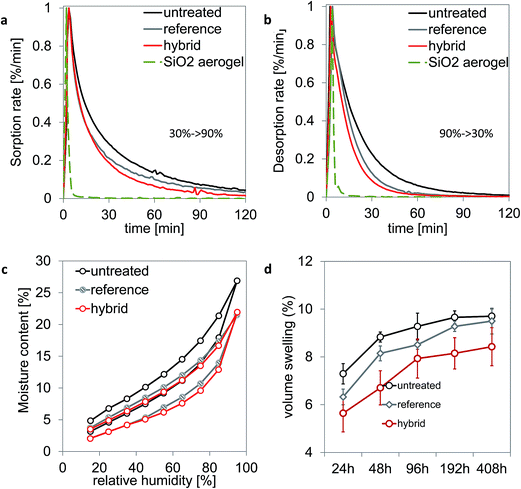 |
| Fig. 5 Interaction of untreated wood, hydrophobized reference, hybrid and pure silica aerogel with water (a) time derivative of sorption for step change of humidity 30–>90% and (b) desorption for 90–>30% RH. (c) Complete water sorption isotherms. (d) Volumetric swelling of dry samples upon conditioning at 80% RH for 40 × 40 × 10 mm3 block specimen. | |
Due to the reduced sorption capacity, swelling strains and thus dimensional stability of the hybrid and also reference materials are improved, as confirmed by dimensional stability measurements on block specimens of the size 4 × 4 × 1 cm3 (Fig. 5d). From an engineering perspective, both, reduced moisture uptake and improved dimensional stability are important motivator for further studies of wood aerogel hybrid materials with a view on construction and building related applications. A reduced moisture retention capacity will help improve the durability of hybrid material against bacterial and fungal attack, particularly at high humidity loads. Also, improved dimensional stability decreases the risk of formation of cracks and loosening of the wood and fasteners interaction in timber engineering. In the same framework, heat transfer and decomposition behaviour of wood–silica aerogel hybrids will influence both thermal insulation performance and fire safety. We observed roughly 30% reduction of the total heat of combustion as measured via pyrolysis combustion flow calorimetry (PCFC) for the hybrid material compared to the untreated wood samples, while there was virtually no improvement for the hydrophobized reference material (Fig. 6a). The measured thermal conductivities differ only slightly from those of the pure wood. In the case of the hydrophobized reference, the lower thermal conductivity is attributed to a partial loss of hemicellulose by chemical dissolution and reduced moisture uptake due to hydrophobization treatment. In the hybrid wood, the thermal conductivity is roughly 10% lower than for the untreated wood due to the filling of the macropores with an additional silica aerogel phase, the latter exhibiting a typical thermal conductivity around 12–15 mW (m−1 K−1). As far as sound wave propagation is concerned, the aerogel hybrid has a damping coefficient which is roughly 60% higher in the longitudinal and 20% higher in the transversal direction when compared to untreated wood (Fig. 6b). The gain in the damping coefficient, as a result of silica aerogel incorporation inside the wood structure is desired for acoustic insulation panels or wood floors with improved acoustic comfort.
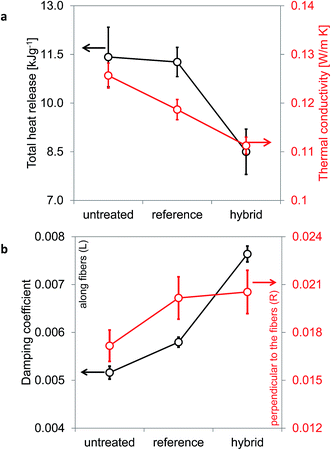 |
| Fig. 6 (a) Total heat release rate (right axis) and thermal conductivity (left axis). (b) Damping coefficient along and perpendicular to the fibres in untreated, hydrophobized reference and hybrid wood. | |
Conclusions
We have in this work presented a proof of concept for the synthesis of a hierarchical wood–silica aerogel nanocomposite through a novel, sol–gel based in situ impregnation route to incorporate mesoporous silica aerogel filler inside the complex cellular substructure of Norway spruce wood. It was shown that the hydrophobization process itself leads to a somewhat reduced moisture uptake which also partially contributes to the improved dimensional stability and thermal insulation properties of the novel hybrid wood material. Owing to the superior thermal properties of the mesoporous silica aerogel, the hierarchically structured hybrid material exhibited reduced total heat of combustion and improved thermal insulation performance as well as internal damping and dimensional stability. Except for the acid catalyzed hydrophobization step, the chemical synthesis process did not influence the physical properties and durability of the wood material. The methodology opens up an avenue for potential future applications for next generation bio-templated hierarchical hybrid aerogel and composite materials.
Acknowledgements
We thank Anja Huch for acquiring the SEM and WDX images, Milijana Jovic for pyrolysis analysis, Dr Anne Bonnin for X-ray tomography imaging and Dr Samuel Brunner for thermal conductivity measurements.
Notes and references
- S. Sakuragawa, Y. Miyazaki, K. Kaneko and T. Makita, J. Wood Sci., 2005, 51, 136 CrossRef.
- V. Brulé, A. Rafsanjani, D. Pasini and T. Western, Plant Sci., 2016, 250, 79–96 CrossRef PubMed.
- L. J. Gibson, J. R. Soc., Interface, 2012, 9, 2749 CrossRef CAS PubMed.
- J. Siau, Transport processes in wood, Springer-Verlag, New York, NY, 1984 Search PubMed.
- V. Bucur, Acoustics of wood, Springer-Verlag, Berlin: Germany, 1987 Search PubMed.
- F. W. M. R. Schwarze and M. Schubert, Appl. Microbiol. Biotechnol., 2011, 92, 431 CrossRef CAS PubMed.
- C. Mai and H. Militz, Wood Sci. Technol., 2004, 37, 339 CrossRef CAS.
- R. Rowel, For. Prod. J., 2006, 56, 4 Search PubMed.
- Y. Yan, Y. Dong, J. Li, S. Zhang, C. Xia, S. Q. Shi and L. Cai, RSC Adv., 2015, 5, 54148 RSC.
- M. A. Ermeydan, E. Cabane, N. Gierlinger, J. Koetz and I. Burgert, RSC Adv., 2014, 25, 12981 RSC.
- H. Miyafuji and S. Saka, J. Wood Sci., 2001, 47, 483 CrossRef CAS.
- G. Tondi, L. Haurie, S. Wieland, A. Petutschnigg, A. Lacasta and J. Monton, Fire Mater., 2013, 38, 381 CrossRef.
- V. Merk, M. Chanana, N. Gierlinger, A. M. Hirt and I. Burgert, ACS Appl. Mater. Interfaces, 2014, 6, 9760 Search PubMed.
- S. Trey, R. T. Olsson, V. Strom, L. Berglund and M. Johansson, RSC Adv., 2014, 4, 35678 RSC.
- V. Merk, M. Chanana, T. Keplinger, S. Gaan and I. Burgert, Green Chem., 2015, 17, 1423 RSC.
- X. Zhu, Y. Wu, C. Tian, Y. Qing and C. Yao, J. Nanomater., 2014, 2014, 8 Search PubMed.
- M. Reim, W. Korner, J. Manara, S. Korder, M. Arduini-Schuster, H. P. Ebert and J. Fricke, Sol. Energy, 2005, 79, 131 CrossRef CAS.
- A. V. Rao and P. B. Wagh, Mater. Chem. Phys., 1998, 53, 13 CrossRef.
- L. W. Hrubesh, J. Non-Cryst. Solids, 1998, 225, 335 CrossRef CAS.
- D. M. Smith, A. Maskara and U. Boes, J. Non-Cryst. Solids, 1998, 225, 254 CrossRef CAS.
- M. Koebel, A. Rigacci and P. Achard, in Aerogels Handbook, Springer, New York, 2011 Search PubMed.
- M. Gronauer and J. Fricke, Acustica, 1986, 59, 176 Search PubMed.
- M. Schmidt and F. Schwertfeger, J. Non-Cryst. Solids, 1998, 225, 364 CrossRef CAS.
- A. Demilecamps, C. Beauger, C. Hildenbrand, A. Rigacci and T. Budtova, Carbohydr. Polym., 2015, 122, 293 CrossRef CAS PubMed.
- S. Zhao, Z. Zhang, G. Sebe, R. Wu, R. V. Rivera Virtudazo, P. Tingaut and M. M. Koebel, Adv. Funct. Mater., 2015, 25, 2326–2334 CrossRef CAS.
- M. Sedighi Gilani, M. N. Boone, J. L. Fife, S. H. Zhao, M. M. Koebel, T. Zimmermann and P. Tingaut, Compos. Sci. Technol., 2016, 124, 71 CrossRef CAS.
- J. Götze, R. Möckel, N. Langhof, M. Hengst and M. Klinger, Ceram.-Silik., 2008, 52, 268 Search PubMed.
- A. Uremovic, T. Dokk, J. Schuseil, B. Saake, A. Borchmann and A. Herrmann, Holz Roh- Werkst., 1994, 52, 347 CrossRef CAS.
- M. Stampanoni, A. Groso, A. Isenegger, G. Mikuljan, Q. Chen, D. Meister, M. Lange, R. Betemps, S. Henein and R. Abela, in Proc. of 9th Conf. on Synchrotron Radiat. Instrum, 2007 Search PubMed.
- D. Paganin, S. C. Mayo, T. E. Gureyev, P. R. Miller and S. W. Wilkins, J. Microsc., 2002, 206, 33 CrossRef CAS PubMed.
- I. Brémaud, PhD dissertation, University of Montpellier II, France, 2006.
- T. Stahl, B. Brunner, M. Zimmermann and K. Ghazi Wakili, Energ. Build., 2012, 44, 114 CrossRef.
- P. R. Ebrahimzadeh and D. G. Kubat, J. Mater. Sci., 1993, 28, 5668 CrossRef CAS.
Footnotes |
† Electronic supplementary information (ESI) available. See DOI: 10.1039/c6ra12480a |
‡ These two authors made equal contributions. |
|
This journal is © The Royal Society of Chemistry 2016 |
Click here to see how this site uses Cookies. View our privacy policy here.