DOI:
10.1039/C6RA10840G
(Paper)
RSC Adv., 2016,
6, 63954-63964
Atmospheric chemistry of CF3(CX2)2CH2OH: rate coefficients and temperature dependence of reactions with chlorine atoms and the subsequent pathways of alkyl and alkoxy radicals (X = H, F)†
Received
27th April 2016
, Accepted 27th June 2016
First published on 28th June 2016
Abstract
The atmospheric and kinetic properties of CF3(CX2)2CH2OH (X = H, F) with chlorine atoms were studied by density functional and canonical variational transition state theories in conjunction with the small-curvature tunneling correction. The minimum energy path was obtained by the CCSD(T)/6-311++G(d,p)//B3LYP/6-311G(d,p) method. The H-abstraction channel from the –CH2O– group was found to be the dominant channel, whereas that from the –OH site of the title reactions may be negligible because of the high barrier. All rate constants computed within 200–1000 K are in reasonable agreement with the available experimental values. The degradation mechanism of CF3(CX2)2CH2OH is discussed. The subsequent pathways of the CF3(CX2)2C˙HOH and CF3(CX2)2C(O˙)HOH radicals were studied. The atmospheric lifetime and global warming potentials (GWPs) of CF3(CX2)2CH2OH were computed, and it is shown that fluorine substitution may increase the lifetime and GWPs. It is also indicated that fluorine substitution may decrease the reactivity. The reaction enthalpies and reaction Gibbs free energies for all relevant reactions were discussed. The rate coefficient expressions for the title reactions obtained are kT1 = 5.75 × 10−17T2.26
exp(428.02/T) and kT2 = 1.30 × 10−17T1.96
exp(67.40/T) per cm3 per molecule per s.
1. Introduction
Atmospheric chemistry of oxygenated or fluorinated volatile organic compounds (VOCs) will have an impact on air quality and the climate.1 Hydrofluoroalcohols (HFAs) are prototypical VOC species which were applied in widespread industrial use as a new generation of chlorofluorocarbon (CFC) alternatives, especially because of their high chemical reactivity and zero ozone depletion ability.2–4 However, the presence of C–F bonds may cause infrared absorption features and thus make a difference in global warming.5 Atmospheric oxidation processes convert fluorinated alcohols to perfluorinated carboxylic acid (PFCA) which is relatively stable in the atmosphere and difficult to degrade by oxidation, hydrolysis and other means.6,7 In the troposphere, HFAs degrade mainly via reaction with OH radicals, however, the reactions with less abundant Cl atoms may also contribute to their removal due to the higher reactivity of Cl atoms.8 Moreover, Cl atoms are detected with high concentrations over the marine boundary layer and coastal atmospheric environments.9,10 In addition, this work will provide information about the primary reaction pathways of HFAs, and therefore about the degradation mechanism of HFAs in the troposphere.
Herein, we report the results obtained in the study of the reactions of Cl radicals with CF3CH2CH2CH2OH and its fluorine-substituted alcohol CF3CF2CF2CH2OH. Three experimental researches have been performed to give the rate constants for the above two reactions, namely, the rate constant about the reaction of CF3CH2CH2CH2OH + Cl obtained from Jimenez et al.11 at room temperature using absolute and relative techniques; and also, Díaz-de-Mera et al.12 and Hurley et al.13 respectively predicted the kinetics constants of the reaction of CF3CF2CF2CH2OH + Cl within 298–353 K and 296 ± 2 K through a discharge flow tube mass spectrometric technique and relative rate techniques. The experimental data obtained from Díaz-de-Mera et al. and Hurley et al. are in good agreement with each other. However, there is still no other work focusing on the title reactions and thus theoretical studies about the branching ratios and temperature dependencies of these reactions are necessary. There are several hydrogen-abstraction processes from CF3(CX2)2CH2OH (X = H, F) by Cl atoms, which are listed below:
|
CF3CH2CH2CH2OH + Cl → CF3CH2CH2CH2O˙ + HCl
| (R1) |
|
CF3CH2CH2CH2OH + Cl → CF3CH2CH2C˙HOH + HCl
| (R2) |
|
CF3CH2CH2CH2OH + Cl → CF3CH2C˙HCH2OH + HCl
| (R3) |
|
CF3CH2CH2CH2OH + Cl → CF3C˙HCH2CH2OH + HCl
| (R4) |
|
CF3CF2CF2CH2OH + Cl → CF3CF2CF2CH2O˙ + HCl
| (R5) |
|
CF3CF2CF2CH2OH + Cl → CF3CF2CF2C˙HOH + HCl
| (R6) |
In the current work, detailed studies about the above reactions are performed using density functional theory. The direct dynamic strategy and canonical variational transition state theory (CVT) are employed to study the kinetic information of the above reactions to predict the primary products and branching ratios of different pathways. Based on our thermodynamic and dynamic calculations, the radicals of CF3(CX2)2CHOH (X = H, F) are considered to be the major intermediates. In fact, in the atmosphere, the radicals of CF3(CX2)2C˙HOH are likely to proceed via the following reactions and other ways (Fig. 1) which are recommended in experiment and theory:
|
CF3(CX2)2C˙HOH + O2 → CF3(CX2)2CHO + HO2
| (R7) |
|
CF3(CX2)2C˙HOH + O2 → CF3(CX2)2C(OO˙)HOH
| (R8) |
|
CF3(CX2)2C(OO˙)HOH + NO → CF3(CX2)2C(O˙)HOH + NO2
| (R9) |
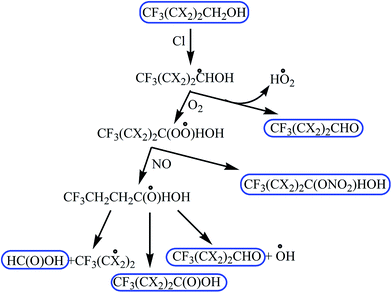 |
| Fig. 1 Atmospheric degradation of [CF3(CX2)2CH2OH] (X = H, F) by Cl atoms to form the alkyl radicals [CF3(CX2)2C˙HOH] (X = H, F) and alkoxy radicals [CF3(CX2)2CH(O˙)OH] (X = H, F). | |
Our calculations show that (R7) is the major reaction channel for the alkyl radicals, and the subsequent reactions from (R8) are secondary. At present, lots of efforts have been focused on the reactivity of alkoxy radicals because of their specificity and variability.1,14–17 There are four possible channels carried out in the subsequent reactions of CF3(CX2)2C(O˙)HOH (X = H, F) involving oxidation process and bond dissociation (C–H, C–C, and C–O) which are listed as follows:
|
CF3(CX2)2C(O˙)HOH + O2 → CF3(CX2)2COOH + HO2˙
| (R10) |
|
CF3(CX2)2C(O˙)HOH → CF3(CX2)2COOH + H
| (R11) |
|
CF3(CX2)2C(O˙)HOH → CF3(CX2)2˙ + HCOOH
| (R12) |
|
CF3(CX2)2C(O˙)HOH → CF3(CX2)2COH + ˙OH
| (R13) |
In this study, we have explored the kinetics in the temperature range of 200–1000 K, which will be used in all practical purposes like modeling of both atmospheric and combustion kinetics. The comparisons between theoretical and experimental results and also the atmospheric degradation mechanism and atmospheric implications of CF3(CX2)2CH2OH (X = H, F) are discussed. Our aim is to gain further insight into the mechanism and kinetics of Cl atoms with CF3(CX2)2CH2OH (X = H, F). The subsequent fate of the CF3(CX2)2C˙HOH and CF3(CX2)2C(O˙)HOH radicals (X = H, F) is also valuable data for the atmospheric chemistry of such species.
2. Theoretical calculation details
In this section, all of the stationary points including the minimum energy structures and saddle points are optimized by the density functional theory Becke’s three-parameter non-local exchange functional with the non-local correlation functional of the Lee–Yang–Parr (B3LYP) method,18,19 along with the standard basis set 6-311G(d,p). We choose B3LYP as the computed method because not only is it an economic and accurate computational model for estimating electronic structure, but it also enables comparisons with the previous research.20–22 Harmonic vibrational frequency analysis is carried out to characterize the stationary and saddle points, and meanwhile intrinsic reaction coordinate (IRC) computations23 are performed to construct the minimum energy paths (MEPs) from the transition state structures to the corresponding local minima. The M06-2X theory,24 which was recently used in similar systems,25–27 is also performed to verify the reliability of the stationary points for the reaction of CF3CF2CF2CH2OH + Cl, and is found to be in agreement with the results obtained at the B3LYP level. To get more reliable energy information about these stationary and saddle points, single point corrections are performed using CCSD(T)/6-311++G(d,p) theory28,29 and the B3LYP-optimized geometries. We have recalculated the single energies employing the CCSD(T)/6-311++G(d,p)//M06-2X/6-311G(d,p) method for optimization of the rate-determining step (CF3(CF2)2CH2OH + Cl → TS6 → CF3(CF2)2C˙HOH + HCl). Compared with the results at the CCSD(T)/6-311++G(d,p)//B3LYP/6-311G(d,p) level, the difference of the activation barrier for this process is 0.04 kcal mol−1 approximately, suggesting that the B3LYP level is reasonable and applicable in the current system. These quantum chemistry calculations are executed by the Gaussian 09 program.30
The rate coefficients for reactions (R1)–(R6) are computed over the wide temperature range of 200–1000 K using the canonical variational transition state theory (CVT)31–33 with the small-curvature tunneling (SCT)34,35 method by performing the POLYRATE 9.7 procedure.36 The partition function of the vibrational modes is adopted from the hindered rotor approximation of Truhlar and Chuang. In the estimation of the reactant electronic partition function, two electronic states of the Cl atom, 2P1/2 and 2P3/2, are considered.
3. Results and discussion
3.1 Stationary points and vibrational frequencies
Geometries of the stationary points and saddle points optimized at the B3LYP/6-311G(d,p) level are shown in Fig. 2. From the structure of TS1–TS6, we can see that the breaking O–H (C–H) bond is 1.277, 1.483, 1.505, 1.481, 1.280, and 1.380 Å, and the elongation is 32.88, 34.82, 37.69, 35.69, 32.78, and 25.80%, respectively. At the same time, the newly forming H–Cl bond in TS1–TS6 is respectively extended by 12.51, 13.13, 11.34, 11.50, 11.66, and 17.17% with respect to the equilibrium H–Cl bond in HCl. The elongation of the breaking bond is larger than that of the forming bond in all transition states, indicating that they are all product-like barriers, and reaction pathways will proceed via late transition states.
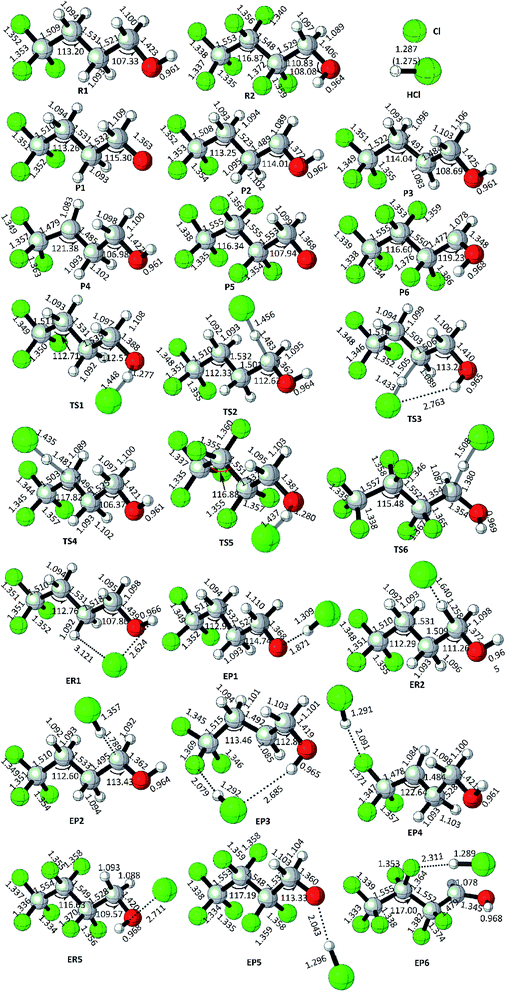 |
| Fig. 2 Optimized geometries of the reactants, products, transition states, and complexes at the B3LYP/6-311G(d,p) level. Bond lengths are in angstroms and angles in degrees. | |
The vibrational frequencies of the stationary points were obtained at the B3LYP/6-311G(d,p) level of theory and are given in Table S1 of the ESI.† All of the optimized geometries of the reactants and products were confirmed with zero imaginary frequencies, and the transition states were confirmed with one imaginary frequency. The values of imaginary frequencies calculated for TS1, TS2, TS3, TS4, TS5, and TS6 are 1113i, 277i, 463i, 690i, 1194i, and 847i cm−1 at the B3LYP/6-311G(d,p) level, respectively.
3.2 Thermodynamics and reaction mechanism
The potential energy surface (PES) is depicted in Fig. 3. For the reaction of CF3CH2CH2CH2OH with a Cl atom, the Cl atom can attack the H atom from –OH (TS1), α-C (TS2), β-C (TS3), and γ-C (TS4). And for the reaction of CF3CF2CF2CH2OH + Cl, there are two transition states, TS5 and TS6, which are caused by the Cl atom attacking the hydroxyl hydrogen and α-C hydrogen of CF3CF2CF2CH2OH. It can be seen from Fig. 3a that the pre-complexes ER1 and ER2 are firstly formed with energy below that of the reactants (CF3CH2CH2CH2OH and Cl). And on the product side, four late complexes (EP1–EP4) are located. The activation energy barriers of (R1)–(R4) are 7.98, −6.50, −0.60, and 3.96 kcal mol−1, which suggest the (R2) is thermodynamically favourable in this reaction. It should be explained that the relative energy of ER2 (−10.43 kcal mol−1) is below that of TS2 (−10.14 kcal mol−1) at the B3LYP/6-311G(d,p) level, however, the relative energy of ER2 (−3.80 kcal mol−1) obtained from the CCSD(T)/6-311++G(d,p)//B3LYP/6-311G(d,p) level is higher than that of TS2 (−6.50 kcal mol−1). A similar situation can be found in other similar reactions with Cl atoms.37,38 Next, we will pay attention to the reaction routes of CF3CF2CF2CH2OH + Cl which are plotted in Fig. 3b. Reaction (R5) may start from the attractive well in the entrance valley of the PES, forming the pre-complex ER5. The stabilization energy is 2.99 kcal mol−1. Then, ER5 undergoes a hydrogen transfer process to form product complex EP1, which lies 0.70 kcal mol−1 below the energies of the corresponding products. For (R6), the products (P6 + HCl) are formed from EP6 via TS6. With respect to the activation barrier heights of channels (R5) and (R6), the energies are 12.20 kcal mol−1 (TS5) and 1.41 kcal mol−1 (TS6), respectively, indicating that (R6) is more favourable as compared to (R5). Given all this, we can summarize that for the reaction of CF3(CX2)2CH2OH (X = H, F) with Cl atoms, by all means the H-abstraction path from the –CH2– group could be dominating the total reaction in kinetics and thermodynamics. The case is similar for the situation appearing in reactions of CH3−nFnCH2OH (n = 1–3)39 and CF3CF2CH2OH
40,41 with Cl atoms. The kinetic rate coefficient computations will prove this view in the following section.
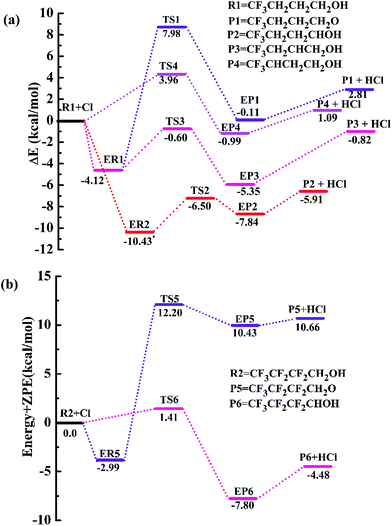 |
| Fig. 3 Schematic potential energy surface (a) for the reaction CF3(CH2)2CH2OH + Cl and (b) for the reaction CF3(CF2)2CH2OH + Cl. Relative energies (in kcal mol−1) are calculated at the CCSD(T)/6-311++G(d,p)//B3LYP/6-311G(d,p) + ZPE level (* obtained at the B3LYP/6-311G(d,p) level). | |
Table 1 lists the relative energies (ΔE), reaction enthalpies (ΔH) and reaction Gibbs free energies (ΔG) of the main species at the B3LYP/6-311G(d,p) and CCSD(T)/6-311++G(d,p)//B3LYP/6-311G(d,p) levels of theory with the ZPE or TZPE correction and T1 diagnostic values obtained at the CCSD(T)/6-311++G(d,p)//B3LYP/6-311G(d,p) level. It can be seen that the T1 diagnostic values of the species calculated at the CCSD(T)/6-311++G(d,p) level are smaller than 0.045, which may indicate that multi-reference character in the CCSD(T) wavefunctions is not a problem. The ΔH data of (R1)–(R6) are respectively 3.41, −5.29, −0.08, 1.88, 11.08, and −3.83 kcal mol−1 at the CCSD(T)/6-311++G(d,p)//B3LYP/6-311G(d,p) level, which suggests that reactions (R2), (R3), (R6) are exothermic and the other reactions are endothermic. In terms of the values of ΔG, except for reactions (R1) and (R5), all the rest of the reactions are spontaneous. For example, the ΔG value is 0.97 for (R1), −7.78 for (R2), −2.98 for (R3), −1.30 for (R4), 8.84 for (R5), and −6.33 kcal mol−1 for (R6). Comparing the activation barrier heights and reaction Gibbs free energies of all reactions, we have found that the favourable channel is the attack on the –CH2– site and the unfavourable pathway is attacking the –OH group for reactions of CF3(CX2)2CH2OH (X = H, F) + Cl. Hurley et al.6 and other authors8,38–41 have studied the kinetics of similar hydrofluoroalcohol reactions with Cl atoms. Their results also show that the Cl atom mainly attacks the –CH2O– group.
Table 1 Relative energies (ΔE) (kcal per mol), reaction enthalpies (ΔH) (kcal per mol), reaction Gibbs free energies (ΔG) (kcal per mol) and T1 diagnostic values of the main species at the CCSD(T)/6-311++G(d,p)//B3LYP/6-311G(d,p) level of theory with ZPE (in a.u.) and TZPE (thermal correction to the enthalpy or Gibbs free energy)
Species |
ZPE |
T1 |
ΔE |
ΔH |
ΔG |
R1 + Cl |
0.114195 |
0.012, 0.006 |
0.00 |
0.00 |
0.00 |
TS1 |
0.106678 |
0.033 |
7.98 |
7.46 |
14.56 |
TS2 |
0.108210 |
0.014 |
−6.50 |
−7.05 |
0.53 |
TS3 |
0.106916 |
0.013 |
−0.60 |
−1.06 |
6.55 |
TS4 |
0.106354 |
0.013 |
3.96 |
3.68 |
10.99 |
P1 + HCl |
0.105435 |
0.014, 0.006 |
2.81 |
3.41 |
0.97 |
P2 + HCl |
0.106978 |
0.014, 0.006 |
−5.91 |
−5.29 |
−7.78 |
P3 + HCl |
0.105990 |
0.013, 0.006 |
−0.82 |
−0.08 |
−2.98 |
P4 + HCl |
0.106200 |
0.013, 0.006 |
1.09 |
1.88 |
−1.30 |
R2 + Cl |
0.081953 |
0.013, 0.006 |
0.00 |
0.00 |
0.00 |
TS5 |
0.073754 |
0.026 |
12.20 |
11.87 |
18.44 |
TS6 |
0.075009 |
0.015 |
1.41 |
0.90 |
7.74 |
P5 + HCl |
0.075956 |
0.015, 0.006 |
10.66 |
11.08 |
8.84 |
P6 + HCl |
0.07478 |
0.015, 0.006 |
−4.48 |
−3.83 |
−6.33 |
3.3 Kinetics and branching ratios
The canonical variational transition state theory (CVT) combined with the small-curvature tunneling (SCT) correction are performed to compute the H-abstraction rate constants of CF3(CX2)2CH2OH (X = H, F) by Cl atoms at the CCSD(T)/6-311++G(d,p)//B3LYP/6-311G(d,p) level. Note that the equilibrium constant between the reactants and pre-reactive complex, and the following VTST rate constant from the complex to the products through the transition state, were taken into account in the dynamic computations. The detailed theoretical discussions can be found in the work of Alvarez-Idaboy et al.42,43 The values of rate constants for reactions of CF3(CH2)2CH2OH + Cl and CF3(CF2)2CH2OH + Cl along with the limited experimental data over the temperature range of 200–1000 K are given in Tables 2 and 3, respectively. The calculated total rate coefficients are depicted in Fig. 4. For the reaction of CF3(CH2)2CH2OH + Cl, only Albaladejo et al.11 measured the rate constant at 298 K. The ratio of k(CVT/SCT)/k(expt) is 1.07 at 298 K, showing that our calculated rate constant is in good agreement with their measured value. For the reaction of CF3(CF2)2CH2OH + Cl, there are two available experimental values measured by Díaz-de-Mera et al.12 and Hurley et al.13 Our calculated rate constants are in good agreement with both experimental results. Comparing with experiment values obtained by Díaz-de-Mera et al., the ratios between k(CVT/SCT) and k(expt) are 1.39, 1.50, 1.53, and 1.68 at 298, 315, 333, and 353 K, respectively. Moreover, the temperature dependence of our rate constants is also in line with the measured results obtained by Díaz-de-Mera et al. From Fig. 4, it is seen that the overall rate constant of the Cl atom with CF3(CH2)2CH2OH is higher than that of the reaction with CF3(CF2)2CH2OH by an order of magnitude at a temperature within 200–1000 K, which is consistent with the previous experimental investigation. Hence, fluorine substitution can decrease the reactivity of the C–H bond by comparing the rate coefficient of the reactions of CF3(CH2)2CH2OH + Cl and CF3(CF2)2CH2OH + Cl. It is also shown that CF3(CH2)2CH2OH may be more suitable for becoming a replacement for the chlorofluorocarbon than CF3(CF2)2CH2OH due to the faster rate coefficient and fewer fluorine atoms. The rate constant of the reactions of the Cl atom with CF3CH2CH2OH is larger than that with CF3CF2CH2OH,6,8 as well as showing that the fluorine substitution can decrease the rate coefficient. As reported in the works of Díaz-de-Mera et al. and Hurley et al.,12,13 the kinetic rate constant for fluorinated alcohols [CF3(CF2)nCH2OH] (n = 1–3) with OH or Cl atoms may be considered as not dependent on the number of –CF2– groups in the perfluorinated chain. However, the rate constant of the reaction of Cl atoms with some fluorinated alcohols at 298 K in experiment or theory follows this order: kCF3CH2CH2CH2OH > kCF3CH2CH2OH > kCF3CH2OH.6,8,11,39 This indicates that the rate constants will increase with the number of –CH2– groups in the reaction with Cl atoms, which is in accordance with the order of the kinetics of some fluorinated alcohols with OH radicals reported in our previous work.44
Table 2 Computed CVT/SCT rate constants (in cm3 per molecule per s) along with the experimental values (italics) for reactions of Cl atoms with CF3(CH2)2CH2OH in the temperature range of 200–1000 K
T (K) |
k1 |
k2 |
k3 |
k4 |
kT1 |
Obtained from ref. 11. |
200 |
1.22 × 10−18 |
7.87 × 10−11 |
1.69 × 10−13 |
1.28 × 10−16 |
7.89 × 10−11 |
220 |
2.99 × 10−18 |
7.95 × 10−11 |
1.86 × 10−13 |
3.87 × 10−16 |
7.97 × 10−11 |
230 |
4.47 × 10−18 |
8.05 × 10−11 |
1.95 × 10−13 |
6.27 × 10−16 |
8.07 × 10−11 |
250 |
9.27 × 10−18 |
8.33 × 10−11 |
2.13 × 10−13 |
1.49 × 10−15 |
8.35 × 10−11 |
270 |
1.77 × 10−17 |
8.69 × 10−11 |
2.33 × 10−13 |
1.78 × 10−15 |
8.71 × 10−11 |
298 |
3.91 × 10−17 |
9.33 × 10−11 |
2.62 × 10−13 |
4.54 × 10−15 |
9.36 × 10−11 |
|
|
|
|
|
(8.71 ± 0.24) × 10−11a |
315 |
6.01 × 10−17 |
9.78 × 10−11 |
2.82 × 10−13 |
7.49 × 10−15 |
9.81 × 10−11 |
333 |
9.14 × 10−17 |
1.03 × 10−10 |
3.03 × 10−13 |
1.21 × 10−14 |
1.03 × 10−10 |
353 |
1.40 × 10−16 |
1.09 × 10−10 |
3.28 × 10−13 |
1.97 × 10−14 |
1.09 × 10−10 |
400 |
3.38 × 10−16 |
1.26 × 10−10 |
3.93 × 10−13 |
5.25 × 10−14 |
1.26 × 10−10 |
500 |
1.03 × 10−15 |
1.69 × 10−10 |
5.58 × 10−13 |
2.52 × 10−13 |
1.70 × 10−10 |
600 |
3.03 × 10−15 |
2.21 × 10−10 |
7.63 × 10−13 |
7.85 × 10−13 |
2.23 × 10−10 |
700 |
7.13 × 10−15 |
2.83 × 10−10 |
9.33 × 10−13 |
1.88 × 10−12 |
2.86 × 10−10 |
800 |
1.44 × 10−14 |
3.53 × 10−10 |
1.20 × 10−12 |
3.78 × 10−12 |
3.58 × 10−10 |
900 |
2.57 × 10−14 |
4.31 × 10−10 |
1.51 × 10−12 |
6.74 × 10−12 |
4.39 × 10−10 |
1000 |
4.28 × 10−14 |
5.16 × 10−10 |
1.87 × 10−12 |
1.10 × 10−11 |
5.29 × 10−10 |
Table 3 Computed CVT/SCT rate constants (in cm3 per molecule per s) along with the experimental values (italics) for reactions of Cl atoms with CF3(CF2)2CH2OH in the temperature range of 200–1000 K
T (K) |
k5 |
k6 |
kT2 |
Obtained from ref. 12. Obtained from ref. 13. |
200 |
2.76 × 10−25 |
5.66 × 10−13 |
5.66 × 10−13 |
220 |
2.00 × 10−24 |
6.73 × 10−13 |
6.73 × 10−13 |
230 |
5.12 × 10−24 |
7.30 × 10−13 |
7.30 × 10−13 |
250 |
3.05 × 10−23 |
8.48 × 10−13 |
8.48 × 10−13 |
270 |
1.59 × 10−22 |
9.74 × 10−13 |
9.74 × 10−13 |
298 |
1.27 × 10−21 |
1.16 × 10−12 |
1.16 × 10−12 |
|
|
|
(8.35 ± 1.63) × 10−13a |
|
|
|
(6.48 ± 0.53) × 10−13b |
315 |
3.95 × 10−21 |
1.28 × 10−12 |
1.28 × 10−12 |
|
|
|
(8.51 ± 1.62) × 10−13a |
333 |
1.18 × 10−20 |
1.42 × 10−12 |
1.42 × 10−12 |
|
|
|
(9.30 ± 1.84) × 10−13a |
353 |
3.58 × 10−20 |
1.57 × 10−12 |
1.57 × 10−12 |
|
|
|
(9.35 ± 1.77) × 10−13a |
400 |
3.31 × 10−19 |
1.97 × 10−12 |
1.97 × 10−12 |
500 |
1.07 × 10−17 |
2.93 × 10−12 |
2.93 × 10−12 |
600 |
1.20 × 10−16 |
4.07 × 10−12 |
4.07 × 10−12 |
700 |
7.26 × 10−16 |
5.40 × 10−12 |
5.40 × 10−12 |
800 |
2.94 × 10−15 |
6.93 × 10−12 |
6.93 × 10−12 |
900 |
9.08 × 10−15 |
8.65 × 10−12 |
8.65 × 10−12 |
1000 |
2.31 × 10−14 |
1.06 × 10−11 |
1.06 × 10−11 |
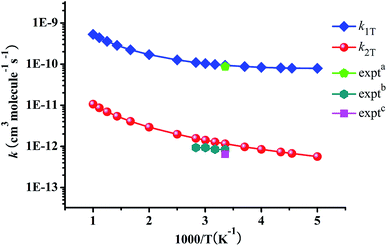 |
| Fig. 4 Computed total CVT/SCT rate constants along with the experimental data as a function of 103/T for the reaction CF3(CH2)2CH2OH + Cl (a), and the reaction CF3(CF2)2CH2OH + Cl (b) in the temperature range of 200–1000 K. | |
In addition, the temperature dependence of the k1/kT1, k2/kT1, k3/kT1, k4/kT1, k5/kT2 and k6/kT2 branching ratios are plotted in Fig. 5a and b. With respect to the reaction CF3(CH2)2CH2OH + Cl, the values of k1 are nearly equal to the total rate coefficient (kT1). Similarly as can be seen from Fig. 5b, the contribution of hydrogen-abstraction of CF3(CF2)2CH2OH + Cl from the –CH2O– group as the major pathway increases in the whole temperature region of 200–1000 K. This is in good agreement with the results about the reaction of CF3(CH2)2CH2OH + OH.44 In contrast, CF3(CX2)2CH2OH (X = H, F) leading to the radicals CF3(CX2)2C˙HOH (X = H, F) and HCl is dominant over the whole temperature range and the contribution from other reaction channels is almost negligible even at higher temperatures. In addition, the temperature-dependent rate expressions for the title reactions obtained are kT1 = 5.75 × 10−17T2.26
exp(428.02/T) and kT2 = 1.30 × 10−17T1.96
exp(67.40/T) per cm3 per molecule per s.
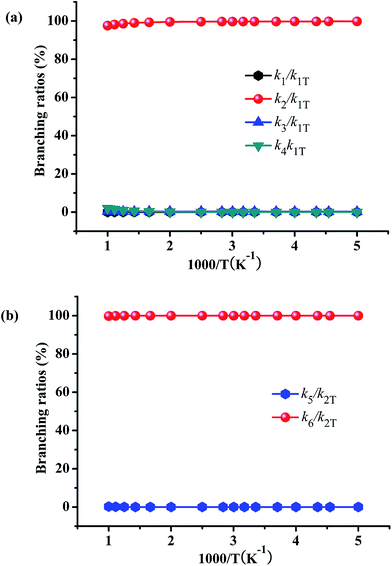 |
| Fig. 5 Computed branching ratios as a function of 103/T (a) for the reaction CF3(CH2)2CH2OH + Cl and (b) for the reaction CF3(CF2)2CH2OH + Cl. | |
3.4 Subsequent behavior of CF3(CX2)2C˙HOH radicals (X = H, F)
Subsequent reaction channels of CF3(CX2)2C˙HOH radicals (X = H, F) in a NO-rich environment are plotted in Fig. 6. The vibrational frequencies of the stationary points obtained at the B3LYP/6-311G(d,p) level of theory are listed in Table S2.† The reaction of CF3(CX2)2CH2OH (X = H, F) with Cl atoms proceeds mainly by H-abstraction from the –CH2O– group, and this leads to the production of CF3(CX2)2C˙HOH (X = H, F) radicals. Then, the CF3(CX2)2C˙HOH (X = H, F) radicals react with O2 through H-abstraction leading to the formation of CF3(CX2)2CHO (X = H, F) by overcoming the −21.78 and −12.05 kcal mol−1 activation barrier heights at the B3LYP/6-311++G(3df,3pd)//B3LYP/6-311G(d,p) level, respectively. This is a spontaneous and exothermic process. We have found that F substitution can passivate the reaction by comparing the activation barrier heights, reaction enthalpies, and reaction Gibbs free energies between CF3(CH2)2C˙HOH + O2 and CF3(CF2)2C˙HOH + O2 reactions. The CF3(CX2)2C˙HOH (X = H, F) radicals can also react with O2 to form the corresponding peroxy radicals of CF3(CX2)2C(OO˙)HOH (X = H, F) as the minor intermediates. Subsequently, the peroxy radicals can also react in the troposphere with NO molecules. The production of O–N in CF3(CX2)2C(OONO˙)HOH (X = H, F) is a barrier-free process with an exothermic energy of −20.26 (−21.14). The elimination of NO2 in the following step produces intermediates CF3(CX2)2C(O˙)HOH (X = H, F) with an exothermic energy of −18.73 and −21.56 kcal mol−1 at the B3LYP/6-311++G(3df,3pd)//B3LYP/6-311G(d,p) level, respectively. F substitution can also passivate the reactions to form the intermediates CF3(CX2)2C(O˙)HOH (X = H, F).
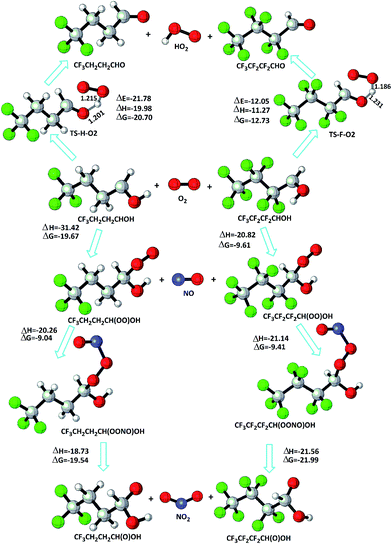 |
| Fig. 6 Optimized geometries and atmospheric degradation mechanism for alkyl radicals [CF3(CX2)2C˙HOH] (X = H, F) at the B3LYP/6-311G(d,p) level of theory. The activation barrier heights, reaction enthalpies, and reaction Gibbs free energies are obtained at the B3LYP/6-311++(3df,3pd)//B3LYP/6-311(d,p) level. | |
3.5 Subsequent behavior of CF3(CX2)2C(O˙)HOH radicals (X = H, F)
As stated previously and in some experimental investigations by Mellouki et al.1 and Orlando et al.,17 alkoxy radicals play crucial roles in the subsequent reactions of H-abstraction from VOCs by free radicals. Atmospheric chemistry of oxygenated and fluorinated VOCs will have an impact on air quality and climate. Therefore, it is necessary to study the subsequent behavior of the CF3(CX2)2C(O˙)HOH radicals (X = H, F). It can be seen from Fig. 7 and Table 4 that CF3(CX2)2C(O˙)HOH radicals (X = H, F) can proceed via four processes, namely, reaction with O2, C–C bond breakage, C–H bond breakage, and C–O bond breakage. As reported in the literature,16,17 the bond breakage process may compete with the oxidation channel. Herein, we have carried out DFT calculations to find out the energy barriers for all the plausible channels. The geometries and structural parameters of the alkoxy radical, transition states for all the possible channels and products are optimized at the B3LYP/6-311G(d,p) level of theory and are shown in Fig. 7. The vibrational frequencies of the stationary points obtained at the B3LYP/6-311G(d,p) level of theory are listed in Table S3.† The transition states labelled TSnO2, TSnC–O, TSnC–C, and TSnC–H (n = 1, 2) correspond to the reaction pathways through reaction with O2, reaction through C–C bond scission, C–H bond scission, and C–O bond scission, respectively. The potential energy profiles for the thermal decomposition and oxidation pathways of the CF3(CX2)2C(O˙)HOH radicals (X = H, F) are depicted in Fig. 8. The single point energy calculations were also carried out to get better energies, at the B3LYP/6-311++G(3df,3pd) level of theory. For the oxidation and C–C/C–H/C–O decomposition reactions of CF3(CH2)2C(O˙)HOH radical, the energy barriers are 9.41, 4.73, 11.62, and 22.96 kcal mol−1, respectively. Also, the energy barriers are respectively 3.29, 2.12, 16.59, and 24.29 kcal mol−1 for the oxidation and C–C/C–H/C–O decomposition pathways of CF3(CF2)2C(O˙)HOH. It is obvious that the decomposition pathways of the C–C bond of CF3(CX2)2C(O˙)HOH radicals (X = H, F) to form formic acid and CF3CX2CX2˙ radicals (X = H, F) go through favourable transition states, that is, these channels require relatively low energy. The decomposition pathways of the C–H bond and C–O bond scission are endothermic reactions (Table 4) at both the B3LYP/6-311G(d,p) and B3LYP/6-311++G(3df,3pd) levels. However, channels of C–C bond scission and reaction with molecular oxygen are exothermic and more favourable. In terms of the values of ΔG, the pathways of C–C/C–H bond scission and reaction with molecular oxygen are spontaneous. Nevertheless, the barrier heights, reaction enthalpies, and Gibbs free energies of the paths of C–H/C–O bond scission are still large compared to those of the reaction with O2 and C–C bond scission. Therefore, from Table 4 we conclude that the thermal decomposition pathways (C–H and C–O) may not compete with the decomposition pathway of the C–C bond. However, the oxidation process may compete with the decomposition pathway of the C–C bond with a difference of the energy barrier of 1.17–4.68 kcal mol−1. By comparing the energy barrier of oxidation and decomposition processes between CF3(CH2)2C(O)HOH and CF3(CF2)2C(O)HOH, we can find that fluorine substitution can promote the oxidation process and C–C bond decomposition process by reducing the energy barrier of 2.61 and 6.12 kcal mol−1, respectively. On the contrary, fluorine substitution will passivate the C–H and C–O scission processes. From the above analysis and Fig. 7, we can see that formic acid and CF3(CX2)2COOH (X = H, F) might be the primary products from the subsequent reactions which will be degraded in the troposphere and contribute to the environmental burden of trifluoroacetic acid (TFA) or derivatives.
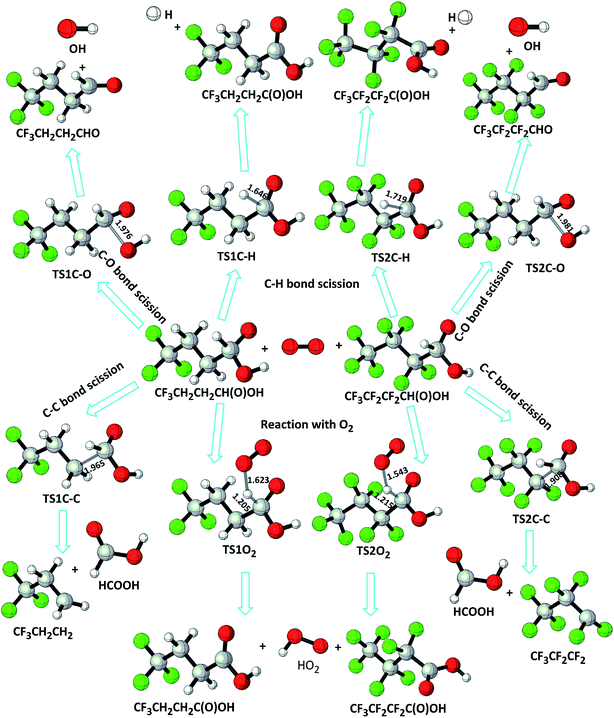 |
| Fig. 7 Optimized geometries and atmospheric degradation mechanism for alkoxy radicals [CF3(CX2)2CH(O˙)OH] (X = H, F) at the B3LYP/6-311G(d,p) level of theory. | |
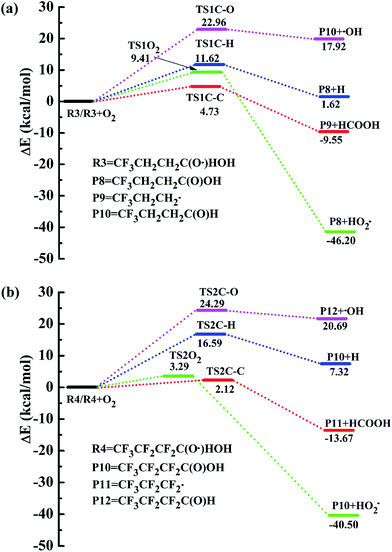 |
| Fig. 8 Schematic potential energy surface (a) for subsequent reactions of CF3(CH2)2C(O˙)HOH and (b) for subsequent reactions of CF3(CF2)2C(O˙)HOH. Relative energies (in kcal mol−1) are calculated at the B3LYP/6-311++G(3df,3pd)//B3LYP/6-311G(d,p) + ZPE level. | |
Table 4 Relative energies (ΔE) (kcal per mol), enthalpies (ΔH) (kcal per mol), and Gibbs free energies (ΔG) (kcal per mol) for the species involved in thermal decomposition of the alkoxy radicals [CF3(CX2)2C(O˙)HOH] (X = H, F) at the B3LYP/6-311++G(3df,3pd)//B3LYP/6-311G(d,p) level of theory
Reaction channels |
ΔE |
ΔH |
ΔG |
TS1O2 (reaction with O2) |
9.41 |
8.82 |
19.19 |
CF3CH2CH2COOH + HO2˙ |
−46.20 |
−46.21 |
−47.32 |
TS1C–H (C–H bond scission) |
11.62 |
11.47 |
11.81 |
CF3CH2CH2COOH + H |
1.62 |
2.77 |
−4.80 |
TS1C–C (C–C bond scission) |
4.73 |
4.74 |
4.34 |
CF3CH2CH2˙ + HCOOH |
−9.55 |
−9.22 |
−20.79 |
TS1C–O (C–O bond scission) |
22.96 |
23.15 |
22.46 |
CF3CH2CH2CHO + ˙OH |
19.72 |
20.99 |
10.48 |
TS2O2 (reaction with O2) |
3.29 |
2.65 |
13.51 |
CF3CF2CF2COOH + HO2˙ |
−40.50 |
−40.55 |
−41.79 |
TS2C–H (C–H bond scission) |
16.59 |
16.43 |
16.80 |
CF3CF2CF2COOH + H |
7.32 |
8.43 |
0.73 |
TS2C–C (C–C bond scission) |
2.12 |
2.02 |
2.01 |
CF3CF2CF2˙ + HCOOH |
−13.67 |
−13.25 |
−26.46 |
TS2C–O (C–O bond scission) |
24.29 |
24.49 |
23.59 |
CF3CF2CF2CHO + ˙OH |
20.69 |
21.95 |
10.85 |
3.6 Atmospheric implications of CF3(CX2)2CH2OH (X = H, F)
The atmospheric lifetimes of CF3(CX2)2CH2OH (X = H, F) determined by Cl atoms (τCl) can be expressed as τCl = (kCl × [Cl])−1. Two different chlorine concentrations such as the global average concentration (1.0 × 103 molecule per cm3)45 and the concentration (1.3 × 105 molecule per cm3)10 at the marine boundary are considered. Using the 270 K value of kT1 = 8.71 × 10−11 cm3 per molecule per s and kT2 = 9.74 × 10−13 cm3 per molecule per s, the estimated atmospheric lifetime of CF3(CH2)2CH2OH and CF3(CF2)2CH2OH is found to be in the range of 0.0028–0.36 years and 0.25–32.56 years, respectively (Table 5). It can be seen that F substitution can dramatically increase the atmospheric lifetimes and it is not environmental friendly. In fact, the global average OH concentration is larger than that of Cl, and thus the roles of its impact on the degradation of CF3(CH2)2CH2OH and CF3(CF2)2CH2OH should be considered. However, the rate coefficients of CF3(CH2)2CH2OH and CF3(CF2)2CH2OH with OH radicals are smaller than those with Cl atoms by 33.24 and 7.26 times, respectively. Antinolo et al.46 and Indulkar et al.47 reported the lifetimes of the above two kind of HFAs determined by OH radicals, which is 4.5 days for CF3(CH2)2CH2OH at 275 K and 0.26 years for CF3(CF2)2CH2OH at 272 K, which are smaller than the lifetimes controlled by Cl atoms in the marine boundary layer. Therefore, we can deduce that Cl atoms may take hold of the degradation process of CF3(CH2)2CH2OH and CF3(CF2)2CH2OH in the marine boundary layer.
Table 5 Computed atmospheric lifetimes at 270 K and global warming potentials (GWPs) of CF3(CH2)2CH2OH and CF3(CF2)2CH2OH computed for the time horizons of 20, 100, and 500 years using the B3LYP/6-311G(d,p) level of theory
Molecules |
[Cl] molecule per cm3 |
Atmospheric lifetime (years) |
GWPs |
20 years |
100 years |
500 years |
CF3CH2CH2CH2OH |
1.3 × 105 |
2.8 × 10−3 |
0.86 |
0.26 |
0.082 |
1 × 103 |
0.36 |
112.36 |
34.38 |
10.74 |
CF3CF2CF2CH2OH |
1.3 × 105 |
0.25 |
99.87 |
30.56 |
9.54 |
1 × 103 |
32.56 |
5959.42 |
3788.75 |
1240.82 |
In addition, CF3(CH2)2CH2OH (0.36 years) and CF3(CF2)2CH2OH (32.56 years) are long-lived at ambient conditions. And the contribution of these compounds to the global warming potential (GWP) may be important at ambient conditions. Thus, we calculated the GWPs relative to carbon dioxide48,49 for CF3(CH2)2CH2OH and CF3(CF2)2CH2OH using the following formula:
|
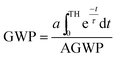 | (1) |
In eqn (1), a is the total instantaneous infrared radiative forcing (W m−2 ppbv−1), TH is the time horizon, and AGWP is the absolute global warming potential for carbon dioxide.50 a in eqn (1) can be obtained from:
|
 | (2) |
where
F(vk) is the radiative forcing function per unit cross-section per wavenumber (W m
−2 (cm
−1)
−1 (cm
2 per molecule)
−1) evaluated at the scaled band center frequency (
vk).
44 Ak is the intensity of the corresponding vibrational mode
k of CF
3(CH
2)
2CH
2OH and CF
3(CF
2)
2CH
2OH obtained at the B3LYP/6-311G(d,p) level of theory. The GWPs computed for different time horizons are presented in
Table 5. The GWPs are found to be large at ambient conditions and small in the marine boundary layer. The GWPs of CF
3(CH
2)
2CH
2OH and CF
3(CF
2)
2CH
2OH are 112.36 and 5959.42 at the 20 year time horizon, respectively. The GWPs of CF
3(CH
2)
2CH
2OH are much smaller when compared with those of CFCs and HFCs. However, the GWPs of CF
3(CF
2)
2CH
2OH may compete with those of CFC-11 (the GWP is 6330 for a 20 year time horizon). This indicates that CF
3(CF
2)
2CH
2OH may be environmentally unfriendly in ambient conditions.
4. Conclusions
In the present work, the rate coefficients for the gas phase reactions of Cl atoms with CF3(CX2)2CH2OH (X = H, F) were computed over the temperature range 200–1000 K using canonical variational transition state theory (CVT) with small-curvature tunneling (SCT) and the CCSD(T)/6-311++G(d,p)//B3LYP/6-311G(d,p) level of theory. A systematic computational investigation is performed on the Cl-initiated oxidation mechanism of CF3(CH2)2CH2OH and CF3(CF2)2CH2OH. On the basis of the energetics and kinetic calculations, it is shown that the attack of the Cl atom occurs predominantly at the –CH2O– position in the test molecules. CF3(CX2)2CH2OH (X = H, F) can rapidly react with OH radicals or Cl atoms and form the corresponding alkyl radicals. CF3(CX2)2C˙HOH (X = H, F) can react with O2 to form CF3(CX2)2CHO (X = H, F) as the major products. Also, the alkoxy radicals of CF3(CX2)2C(O˙)HOH (X = H, F) may be produced by reaction of CF3(CX2)2C(OO˙)HOH (X = H, F) with NO. Then, CF3(CX2)2C(O˙)HOH (X = H, F) can further proceed via oxidation and bond dissociation processes (C–H, C–C, and C–O) to form the corresponding products. On the basis of our computational studies, it is concluded that the thermal decomposition pathways (C–H, C–C, and C–O bond scissions) do not compete with the oxidation pathway. In addition, the atmospheric lifetimes of CF3(CH2)2CH2OH are very short and it may not contribute to global warming. However, the atmospheric lifetimes of CF3(CF2)2CH2OH are still long in the global atmosphere, suggesting that it might have a contribution toward the global warming potentials. The calculated GWPs in this paper can explain the view thoroughly.
Acknowledgements
The authors thank Professor Donald G. Truhlar for providing the Polyrate, Version 9.7 program. We acknowledge financial support from the Natural Science Foundation of China (No. 21377021).
References
- A. Mellouki, T. J. Wallington and J. Chen, Chem. Rev., 2015, 115, 3984–4014 CrossRef CAS PubMed.
- B. Rajakumar, J. B. Burkholder and A. R. Ravishankara, Phys. Chem. Chem. Phys., 2005, 7, 2498–2505 RSC.
- S. R. Sellevg, C. J. Nielsen, O. A. Søvde, G. Myhre, J. K. Sundet, F. Stordal and I. S. A. Isaken, Atmos. Environ., 2004, 38, 6725–6735 CrossRef.
- E. S. Vasil’ev, I. I. Morozov, W. Hack, K. H. Hoyermann and M. Hold, Kinet. Catal., 2006, 47, 834–845 CrossRef.
- I. Bravo, A. Aranda, M. D. Hurley, G. Marston, D. R. Nutt, K. P. Shine, K. Smith and T. J. Wallington, J. Geophys. Res., 2010, 115, D24317 CrossRef.
- M. D. Hurley, J. A. Misner, J. C. Ball, T. J. Wallington, D. A. Ellis, J. W. Martin, S. A. Mabury and M. P. Sulbaek Andersen, J. Phys. Chem. A, 2005, 109, 9816–9826 CrossRef CAS PubMed.
- C. J. Young, V. I. Furdui, J. Franklin, R. M. Koerner, D. C. G. Muir and S. A. Mabury, Environ. Sci. Technol., 2007, 41, 3455–3461 CrossRef CAS PubMed.
- V. C. Papadimitriou, D. K. Papanastasiou, V. G. Stefanopoulos, A. M. Zaras, Y. G. Lazarou and P. Papagiannakopoulos, J. Phys. Chem. A, 2007, 111, 11608–11617 CrossRef CAS PubMed.
- K. W. Oum, M. J. Lakin, D. O. DeHaan, T. Brauers and B. J. Finlayson-Pitts, Science, 1998, 279, 74–76 CrossRef CAS PubMed.
- C. W. Spicer, E. G. Chapman, B. J. Finlayson-Pitts, R. A. Plastridge, J. M. Hubbe, J. D. Fast and C. M. Berkowitz, Nature, 1998, 394, 353–356 CrossRef CAS.
- E. Jimenez, M. Antinolo, B. Ballesteros, E. Martinez and J. Albaladejo, ChemPhysChem, 2010, 11, 4079–4087 CrossRef CAS PubMed.
- Y. Díaz-de-Mera, A. Aranda, I. Bravo, D. Rodriguez, A. Rodriguez and E. Moreno, Environ. Sci. Pollut. Res., 2008, 15, 584–591 CrossRef PubMed.
- M. D. Hurley, T. J. Wallington, M. P. Sulbaek Andersen, D. A. Ellis, J. W. Martin and S. A. Mabury, J. Phys. Chem. A, 2004, 108, 1973–1979 CrossRef CAS.
- G. Srinivasulu and B. Rajakumar, J. Phys. Chem. A, 2015, 119, 9294–9306 CrossRef CAS PubMed.
- G. Srinivasulu and B. Rajakumar, J. Phys. Chem. A, 2013, 117, 4534–4544 CrossRef CAS PubMed.
- B. K. Mishra, RSC Adv., 2014, 4, 16759–16764 RSC.
- J. J. Orlando, G. S. Tyndall and T. J. Wallington, Chem. Rev., 2003, 103, 4657–4689 CrossRef CAS PubMed.
- A. D. Becke, Density-functional thermochemistry. III, J. Chem. Phys., 1993, 98, 5648–5652 CrossRef CAS.
- P. J. Stephen, F. J. Devlin, C. F. Chabalowski and M. J. Frisch, J. Chem. Phys., 1994, 98, 11623–11627 CrossRef.
- F. Y. Bai, G. Sun, X. Wang, Y. Q. Sun, R. S. Wang and X. M. Pan, J. Phys. Chem. A, 2015, 119, 1256–1266 CrossRef CAS PubMed.
- F. Y. Bai, X. L. Zhu, Z. M. Jia, X. Wang, Y. Q. Sun, R. S. Wang and X. M. Pan, ChemPhysChem, 2015, 16, 1768–1776 CrossRef CAS PubMed.
- F. Y. Bai, X. Wang, Y. Q. Sun and X. M. Pan, RSC Adv., 2015, 5, 88087–88095 RSC.
- C. Gonzalez and H. B. Schlegel, J. Chem. Phys., 1989, 90, 2154–2161 CrossRef CAS.
- Y. Zhao and D. G. Truhlar, Acc. Chem. Res., 2008, 41, 157–167 CrossRef CAS PubMed.
- B. K. Mishraa, M. Lily, A. K. Chandra and R. C. Deka, J. Phys. Org. Chem., 2014, 27, 811–817 CrossRef.
- D. Bhattacharjee, B. K. Mishra and R. C. Deka, J. Mol. Model., 2015, 21, 69 CrossRef PubMed.
- B. K. Mishraa, M. Lily, A. K. Chandra and R. C. Deka, New J. Chem. 10.1039/c5nj02752g.
- G. D. Purvis and R. J. Bartlett, J. Chem. Phys., 1982, 76, 1910–1918 CrossRef CAS.
- G. E. Scuseria, C. L. Janssen and H. F. Schaefer, J. Chem. Phys., 1988, 89, 7382–7387 CrossRef CAS.
- M. J. Frisch, G. W. Trucks, H. B. Schlegel, P. W. M. Gill, B. G. Johnson, M. A. Robb, J. R. Cheeseman, T. A. Keith, G. A. Petersson and J. A. Pople, et al., Gaussian 09, Gaussian Inc., Wallingford, CT, 2009 Search PubMed.
- B. C. Garrett and D. G. Truhlar, J. Am. Chem. Soc., 1979, 101, 4534–4548 CrossRef CAS.
- B. C. Garrett, D. G. Truhlar, R. S. Grev and A. W. Magnuson, J. Phys. Chem., 1980, 84, 1730–1748 CrossRef CAS.
- A. Gonzalez-Lafont, T. N. Truong and D. G. Truhlar, J. Chem. Phys., 1991, 95, 8875–8894 CrossRef CAS.
- Y. P. Liu, G. C. Lynch, T. N. Truong, D. H. Lu, D. G. Truhlar and B. C. Garrett, J. Am. Chem. Soc., 1993, 115, 2408–2415 CrossRef CAS.
- D. H. Lu, T. N. Truong, V. S. Melissas, G. C. Lynch, Y. P. Liu, B. C. Garrett, R. Steckler, A. D. Isaacson, S. N. Rai and G. C. Hancock, Comput. Phys. Commun., 1992, 71, 235–262 CrossRef CAS.
- J. C. Corchado, Y. Y. Chuang, P. L. Fast, W. P. Hu, Y. P. Liu, G. C. Lynch, K. A. Nguyen, C. F. Jackels, A. Fernandez-Ramos and B. A. Ellingson, et al., POLYRATE, version 9.7, University of Minnesota, Minneapolis, MN, 2007 Search PubMed.
- H. Q. He, J. Y. Liu, Z. S. Li and C. C. Sun, J. Comput. Chem., 2005, 26, 642–650 CrossRef CAS PubMed.
- Y. M. Ji, J. Y. Wu, J. Y. Liu, Z. S. Li and C. C. Sun, Chem. Phys. Lett., 2006, 417, 345–350 CrossRef CAS.
- Y. Wang, J. Y. Liu and Z. S. Li, Ab initio direct dynamics studies on the reactions of chlorine atom with CH3−nFnCH2OH (n = 1–3), J. Comput. Chem., 2007, 28, 2517–2530 CrossRef CAS PubMed.
- A. Garzón, M. Antiñolo, M. Moral, A. Notario, E. Jiménez, M. Fernández-Gómez and J. Albaladejo, Mol. Phys., 2013, 111, 753–763 CrossRef.
- A. Y. Yu and H. X. Zhang, J. Mol. Model., 2013, 19, 4503–4510 CrossRef CAS PubMed.
- J. R. Alvarez-Idaboy, N. Mora-Diez, R. J. Boyd and A. Vivier-Bunge, J. Am. Chem. Soc., 2001, 123, 2018–2024 CrossRef CAS PubMed.
- J. R. Alvarez-Idaboy, L. Reyes and N. Mora-Diez, Org. Biomol. Chem., 2007, 5, 3682–3689 CAS.
- F. Y. Bai, X. Wang, Y. Q. Sun, R. S. Wang and X. M. Pan, RSC Adv., 2016, 6, 36096–36108 RSC.
- O. W. Wingenter, D. R. Blake, N. J. Blake, B. C. Sive, F. S. Rowland, E. Atlas and F. J. Flocke, J. Geophys. Res., 1999, 104, 21819–21828 CrossRef CAS.
- M. Antinolo, E. Jimenez and J. Albaladejo, Environ. Sci. Technol., 2011, 45, 4323–4330 CrossRef CAS PubMed.
- Y. N. Indulkar, S. SenGupta, S. B. Waghmode, A. Kumar, S. Dhanya and P. D. Naik, Atmos. Environ., 2011, 45, 6973–6979 CrossRef CAS.
- P. Blowers, D. M. Moline, K. F. Tetrault, R. R. Wheeler and S. L. Tuchawena, J. Geophys. Res.: Atmos., 2007, 112, D15108 CrossRef.
- S. Pinnock, M. D. Hurley, K. P. Shine, T. J. Wallington and T. J. Smyth, J. Geophys. Res.: Atmos., 1995, 100, 23227–23238 CrossRef CAS.
- D. J. Wuebbles, Annu. Rev. Energy Environ., 1995, 20, 45–70 CrossRef.
Footnote |
† Electronic supplementary information (ESI) available. See DOI: 10.1039/c6ra10840g |
|
This journal is © The Royal Society of Chemistry 2016 |