DOI:
10.1039/C6RA10378B
(Paper)
RSC Adv., 2016,
6, 89776-89784
Pickering emulsion gels based on insoluble chitosan/gelatin electrostatic complexes†
Received
21st April 2016
, Accepted 12th September 2016
First published on 13th September 2016
Abstract
Food-grade colloidal particles or complexes made from natural polymers via noncovalent interactions can be good candidates for applications in food and non-food industries. In this work, insoluble chitosan/gelatin-B (CH/GB) complex particles were used for the first time as effective Pickering emulsifiers to make long-term stable oil-in-water emulsions and emulsion gels by one-step homogenization. The CH/GB complexes were only formed in the oppositely charged pH region via electrostatic complexation of CH and GB, and the pH showed a remarkable effect on the surface activity of CH/GB mixed systems. The presence of CH/GB insoluble complexes significantly decreased the surface tension of the oil/water interface, therefore benefitting the formation of smaller emulsion droplet sizes and effectively hindering droplet coalescence, as supported by the increase of emulsion long-term stability. Increasing the oil volume fraction considerably accelerated the formation of a droplet network structure, resulting in a solid-like thermoreversible emulsion gel with tunable viscoelastic properties. Our results suggest that CH/GB insoluble complex particles are promising particulate emulsifiers for the preparation of surfactant-free Pickering emulsions as well as emulsion gels.
Introduction
Pickering emulsions are emulsions stabilized by solid or colloidal particles (e.g., complexes) instead of surfactants, either water-in-oil (W/O), oil-in-water (O/W), or even multiple emulsions, with very high resistance to coalescence.1,2 Recently, the development of bio-sourced particulate Pickering emulsifiers has received increasing attention, since most of the particles used in the fundamental studies of Pickering emulsions are synthetic or inorganic particles (e.g., silica, TiO2 particles), which greatly limits their applications in food and beverage industries.3,4 However, finding an effective bio-based Pickering emulsifier is challenging because it should have intermediate wettability and meanwhile insolubility in both the water and oil phases.5,6 In the past decades, only a few biocompatible materials, including chitin nanocrystal particles,7 chitosan aggregates,8,9 starch granules,10 alkaline lignin,11 cellulose particles12 and water-insoluble zein,13 have been shown employable as effective Pickering emulsifiers. Therefore, to meet the variable requests for specific applications, the development of more bio-based and even edible Pickering emulsifiers is desirable.
Here, we introduce chitosan/gelatin-B (CH/GB) complex particles as a novel class of Pickering emulsifier. Both chitosan and gelatin are bio-sourced polyelectrolytes (chitosan is a polycation and gelatin is a polyampholyte), and they are biocompatible, biodegradable polymers and also abundantly and commercially available.14,15 Specifically, chitosan is the only natural-occurring cationic polysaccharide, obtained from the alkaline deacetylation of chitin, while gelatin is a linear protein, derived from collagen either by acid hydrolysis (type A, pI = pH 7–9) or alkaline treatment (type B, pI = pH 4.8–5.2). We have shown that the complexation of CH and GB is a kinetic process, and CH/GB complexes can evolve from soluble to insoluble complexes, to eventually form a colloidal gel.16 In the present work, we mainly focus on the temporal evolution of insoluble CH/GB complexes and the use of these complex particles as Pickering emulsifiers.
The electrostatic-driven complexation between proteins and polysaccharides has been investigated extensively.17–19 Mainly depending on the molecular charge density and conformation, three different structures can be formed based on the electrostatic attraction between proteins and polysaccharides, i.e., soluble/insoluble complexes,20,21 gels22 and coacervates.23 It is still not completely understood which mechanisms favour the formation of these different structures, but a general trend emerges where proteins or polysaccharides having high charge density and/or stiff structure preferably form complexes, whereas the reverse, i.e., moderate charge density and flexible conformation, favours the formation of coacervates.17 There are several studies using protein/polysaccharide complexes directly to form emulsions, or fabricating emulsions by having polysaccharide molecules absorbed on protein-coated oil droplets to form an interfacial complex layer.24–27
CH/GB complex systems have also been investigated. However, most of the previous work focuses on the applications of CH/GB complexes.28–30 Few studies are devoted to understanding the complexation process between CH and GB.31,32 To the best of our knowledge, no studies have systematically examined the temporal evolution of CH/GB complexes at different pH. Since many protein/polysaccharide systems macroscopically change (e.g., from being transparent to turbid, or even leading to phase separation) over the course of minutes to days, the effect of the time-induced structural evolution of protein/polysaccharide systems is of great importance. The CH/GB system is particularly interesting because the majority of protein/polysaccharide complexes reported either involves negatively charged polysaccharides or CH (the only positively charged natural polysaccharide) but with globular proteins, while CH and GB both possess linear molecular structures.
To date, few studies reported on the utilization of CH/GB complexes as Pickering emulsifiers. As compared with existing particulate stabilizers, CH/GB complex nanoparticles acting as Pickering emulsifiers would have the following advantages: (i) they have positive charges,16 which results in cationic droplets that make emulsions less prone to destabilization by multivalent cations (e.g., Ca2+),33 and gives a high antimicrobial efficacy;34 (ii) they are made from nutritional and functional food ingredients; (iii) they can form networks, and as a consequence, a gel-like emulsion structure will lead to extraordinary stability against coalescence.16 In this work, we investigate the role of CH/GB insoluble complex particles as Pickering emulsion stabilizers. In addition, the influence of parameters such as pH and oil fraction on the emulsion stability is examined, while the viscoelastic properties of the resulting Pickering emulsion gels are characterized.
Experimental
Materials
Gelatin type B (GB) with bloom strength of 75 and molecular weight in the range of 20–25 kDa, was purchased from Sigma-Aldrich Canada (Oakville, ON). Commercial chitosan (CH) with degree of deacetylation (DDA) of 85%, dynamic viscosity of 60 mPa s, and weight-average molecular weight of 173 kDa was supplied in powder form by BioLog Biotechnologie und Logistik GmbH (Landsberg, Germany). A fluorescent marker, fluorescein isothiocyanate (FITC) was purchased from Sigma-Aldrich (Canada). Acetic acid (99.9% AcOH) from Fisher Scientific (USA) was used to dissolve chitosan. Corn oil was purchased from a local supermarket in Montreal (Canada). All other chemicals used in this work were of analytical grade from Sigma-Aldrich.
Preparation of CH/GB insoluble complexes
CH and GB solutions were first prepared separately: CH was dissolved in a 1% (v/v) acetic acid solution and stirred with a magnetic stirrer at 300 rpm for 4 h, and then stored overnight at room temperature to allow complete hydration and dissolution. GB was dissolved in deionized water at 40 °C and stirred (300 rpm) for 30 min. CH/GB mixtures were prepared by mixing CH and GB solutions at a volume ratio of 1
:
2 (CH
:
GB) and stirred (300 rpm) for 30 min at room temperature. The pH of the mixtures was adjusted from 3.5 to 6.5 using NaOH or HCl solutions at concentrations of 0.5 to 5 M (higher concentration was used first to minimize the dilution effect for each pH adjustment), and then the CH/GB mixtures were stored at room temperature for up to 5 days to allow the formation of insoluble complexes. The concentrations of CH, GB and CH/GB aqueous solutions were varied from 0.1 to 1 wt%, depending on the requirement for characterization.
Preparation of CH/GB emulsions
CH/GB mixtures at a total polymer concentration of 1 wt% were used to prepare CH/GB-stabilized oil/water emulsions, at different pH (3.5 to 6.5). Specifically, CH/GB aqueous solutions were mixed with corn oil at an oil volume fraction (φ) from 0.1 to 0.4, and then homogenized using a high intensity ultrasonic processor (UIP1000hd (20 kHz, 1000 W), Hielscher Ultrasonics GmbH, Teltow, Germany) with 90% amplitude for 8 min. An ice bath was used to control temperature and avoid overheating. For comparison purposes, oil/water emulsions were also prepared with either CH or GB aqueous solutions. All the emulsions were stored at room temperature for further observation and characterization.
Characterization of CH/GB insoluble complexes
Zeta (ζ) potential and particle size (hydrodynamic diameter) of three individual samples of the same solutions were measured using a dynamic light scattering instrument (Nano-ZPS, Malvern Instruments, Worcestershire, UK). The turbidity evolution was monitored using a UV-Vis Varian spectrophotometer (Cary100, Palo Alto, CA, USA) in transmittance mode (%T) at 633 nm, by plotting the turbidity index (100 − %T at 633 nm) against pH. The microstructure of CH/GB insoluble complexes was observed using confocal laser scanning microscopy (CSLM) (Model Leica TCS SP5, Leica Microsystems Inc., Heidelberg, Germany), equipped with an inverted microscope (Model Leica DMI6000). Before CLSM observation, labeling was conducted using the method established by Sanchez et al.35 GB was firstly labeled by covalently linking a minimum amount of fluorescent markers (fluorescein isothiocyanate, FITC) at pH 8.5 for 90 min, which was shown to have negligible effect on the properties of GB,36 and then FITC-GB solutions were mixed with CH solutions at pH 5.5 to form CH/GB complexes. The interfacial surface tension of CH/GB mixtures and their solvents were determined using an optical contact angle measuring system (OCA-20, DataPhysics Instruments GmbH, Germany) by analyzing the pendant drop shape using the Young–Laplace equation. The data of interfacial tension were then used to calculate surface pressure (π), which is equal to γs − γc, where γc and γs are the surface tensions of CH/GB mixtures and the corresponding solvent (at a comparable test time), respectively.
Characterization of CH/GB emulsions
The microstructure of freshly prepared CH/GB emulsions was observed with a Zeiss Axioskop 40 optical microscope (Carl Zeiss Microscopy GmbH, Jena, Germany). The average emulsion droplet size in terms of volume-average diameter (dv) and number-average diameter (dn) was calculated from microscopy images using a digitizing table from Wacom and SigmaScan v.5 software. The calculation was performed by randomly selecting three images from two parallel experiments and choosing at least 500 droplets. The long-term emulsion stability was assessed by visual observation and calculation of the creaming index (CI), which is reported as (Hs/Ht) × 100, where Ht is the total height of the emulsion and Hs is the height of the serum layer (the sum of the transparent and/or the turbid layers at the bottom of the container). Viscoelastic properties of CH/GB emulsions were characterized at 25 °C using a rotational rheometer (MCR 502, Anton Paar, Graz, Austria) under small amplitude oscillatory shear (SAOS) mode. A regular Couette geometry (with cup and bob diameters of 18.08 and 16.66 mm, respectively) was used. A time sweep was first conducted at 1 rad s−1 for 20 min, showing that the CH/GB emulsions were stable within the test time range. Then a frequency sweep was carried out from 1 to 100 rad s−1 at 25 °C within the linear viscoelastic range. In addition, several temperature sweeps were performed at a constant frequency (1 rad s−1). Specifically, the system temperature was first increased from 25 to 60 °C at a heating rate of 2 °C min−1, and then decreased to 5 °C with a cooling rate of 2 °C min−1, and then two further heating and cooling cycles were carried out between 5 and 60 °C. All measurements at room temperature were also carried out at least in duplicates, unless otherwise specified.
Results and discussion
Evolution of CH/GB complexes
The complexation of proteins and polysaccharides is a kinetic process. To clarify the evolution mechanism from solubility to insolubility, the physical properties of CH/GB complexes, including zeta (ζ) potential, turbidity and microstructure were characterized as a function of time. Fig. 1A reports the ζ potential of CH, GB and CH/GB aqueous solutions for different storage times (from day 1 (preparation day) to day 5), and as a function of pH. CH exhibits positive charges in the tested pH range and its ζ potential decreases as pH increases, due to the deprotonation of amino groups. On the other hand, the net charge of GB is positive at pH 3.5 and 4.5, but negative at 5.5 and 6.5 (the isoelectric point of gelatin-B is around pH 5.0). The ζ potential of the CH/GB mixtures gradually decreases as pH increases from 3.5 to 6.5, and the charge density of CH/GB gets gradually closer to that of CH at higher pH values (e.g., pH 6.5). If there would be no interaction between CH and GB, the ζ potential of the CH/GB mixture could be obtained from a simple mixing rule such as ζCH/GB = 1/3 × ζCH + 2/3 × ζGB, where ζCH and ζGB are respectively the ζ potentials of CH and GB at a corresponding pH and for a ratio of 1
:
2 (CH
:
GB). The calculated result is compared in Fig. 1B with the actual values of ζCH and ζCH/GB for freshly prepared samples. We can see that the latter evolves gradually away from the calculated ζCH/GB but closer to ζCH, as pH increases to 6.5. Previous studies have shown that oppositely charged polyelectrolytes can form complex particles with a neutralized core and a shell made of the excess component in the case of non-stoichiometric ratios.37,38 In the CH/GB system, there is almost no electrostatic interaction between CH and GB at pH 3.5; at pH 4.5, very weak complexation occurs between CH and the negatively charged patches of the GB molecules; however, at pH 5.5 and 6.5, the electrostatic attraction force between CH and GB is enough to form a neutralized core with a shell containing excess CH charges, which would explain why ζCH/GB is almost the same as ζCH in the opposite charge region. Fig. 1A also shows that an increase in storage time does not change the ζ potential of CH/GB in the similar charge region (pH 3.5 and 4.5), but slightly decreases it in the opposite charge region (pH 5.5 and 6.5). It indicates that electrostatic interactions may not be the only driving force in the growth of the CH/GB complexes, although they play a very important role in the formation of primary complex particles. As seen for other polyelectrolyte complex systems,16,30 the evolution of CH/GB complexes is most probably an intricate interplay of electrostatic, van der Waals, hydrogen bonding, and hydrophobic interactions.
 |
| Fig. 1 (A) Zeta potential of CH solutions (0.1 wt%), GB solutions (0.1 wt%) and CH/GB mixtures (0.1 wt%, CH : GB = 1 : 2) at different storage times, as a function of pH; (B) comparison of ζCH, ζCH/GB, and calculated ζCH/GB for freshly prepared samples. | |
The temporal evolution of CH/GB complexes was monitored by measuring turbidity, hydrodynamic diameter, and examination of the microstructure using CLSM. As shown in Fig. 2A, increasing storage time up to 5 days significantly changes the turbidity curves plotted against pH: in the opposite charge range, turbidity indexes steadily increase with time and the maximum is located at pH 5.5, which is in accordance with visual observations shown in Fig. S1 (ESI†). It implies that the size of CH/GB complexes increases with time and that they evolve from soluble to insoluble. Fig. 2B shows the CLSM image of CH/GB insoluble complexes at pH 5.5 and 3 days coexist with their flocs (green particles represent CH/GB complexes). It is believed that the growth of these flocs might be the main reason for the turbidity increase. As shown in Fig. 2C, an increase of pH from 3.5 to 6.5 results in an increase of the hydrodynamic diameter (volume average diameter, dv increases from 6.2 to 212.1 μm), indicating the formation of CH/GB complexes at higher pH (e.g., pH 5.5 and 6.5). After 3 days of storage, no significant change is observed for pH 3.5 and 4.5, but a remarkable increase of the hydrodynamic diameter can be seen for pH 5.5 and 6.5, e.g., dv increases from 33.9 to 459.3 μm for pH 5.5, suggesting the growth of CH/GB flocculates with time. Meanwhile, considering that the CH/GB-stabilized emulsions were prepared using high intensity ultrasonic (HIU) homogenization, the effect of HIU on the CH/GB complex particle size was also examined. Interestingly, after treating the insoluble CH/GB complexes formed at pH 5.5 and 6.5 (3 days of age) with HIU, the dv decreases significantly, e.g., it drops from 459.3 and 877.2 to 39.5 and 60.8 nm for pH 5.5 and 6.5, respectively. The hydrodynamic diameter data agrees well with the visual observation of HIU-treated CH/GB complexes, which becomes transparent after HIU treatment (Fig. S2, ESI†). Based on the analysis of Fig. 1, it is already known that CH/GB complex particles are colloidal, with a ζ potential lower than 20 mV. Generally, a ζ potential larger than 30 mV is necessary for a stable electrostatic stabilized dispersion, otherwise, flocculation may take place. The origin for particles flocculation was examined from a theoretical point of view in a recent work, which describes that same-charge complex particles tend to interact with each other to form larger secondary particles, once short-range attractions (e.g., van der Waals, hydrophobic effect) overcome long-range electrostatic repulsion.37
 |
| Fig. 2 (A) Time evolution of turbidity for CH/GB mixtures (1 wt%, CH : GB = 1 : 2) as a function of pH; (B) CLSM images of CH/GB complexes (1 wt%, CH : GB = 1 : 2, pH 5.5, 3 days of age); (C) hydrodynamic diameter of CH/GB complexes as functions of pH, storage time and HIU treatment. D1 indicates freshly prepared CH/GB complexes and D3 with three days of age, and D3-HIU means CH/GB complexes stored for 3 days and then treated with HIU for 8 min. | |
In addition, the difference between CH/GB complexes particles formed at pH 5.5 and 6.5 also need to be considered. It should be noted that the molecular weight of CH (173 kDa) used in this work is about 8 times that of GB (20–25 kDa), and thus when CH and GB interact with each other, CH may act as a framework in the structuration of the CH/GB complex particles. Another point is that the conformation of CH greatly depends on pH, and the extended molecular structure can gradually turn to be more compact as pH increases, mainly due to the deprotonation of amino groups.16,39 Therefore, the structure of the corresponding CH/GB complexes should be denser at pH 6.5, while looser at pH 5.5.
Note that for CH/GB mixtures, it is difficult to prepare complexes at pH higher than 6.5 (the pKa of chitosan) due to the agglomeration of chitosan molecules, as shown in Fig. S1 (ESI†), eventually followed by precipitation.
Interfacial activities of insoluble CH/GB complexes
The interfacial adsorption kinetics of insoluble CH/GB complexes (3 days of age) was characterized, and the role of pH (3.5 to 5.5) was examined. Surface pressure (π) is plotted against t1/2 (up to 2.5 h) in Fig. 3, and in the initial diffusion-controlled adsorption process, the slope of the plot represents the diffusion rate constant (kdiff). The values of kdiff and π at the beginning (0 s; π0) and end (9000 s; π9000) of adsorption are summarized in Table 1. All the curves show an increase in surface pressure (π), especially in the first 400 s, however, there is a significant difference between the tested pH values: the higher the pH, the higher the π value, indicating better surface activities. At pH 3.5, the suspension is made of soluble mixed biopolymers, since there is almost no interaction between CH and GB. Thus, CH and GB molecules tend to absorb independently on the oil/water interface. Compared to the π value of CH alone at a comparable time,40 π is higher because of the presence of GB molecules. At pH 4.5, based on the analysis of zeta potential results, soluble complexes of CH and GB are formed, and as a consequence, they absorb on the oil/water interface and thus decrease the interfacial tension. As pH increases to 5.5, the adsorption of insoluble CH/GB complexes greatly increases the π value as compared to CH at the same pH (e.g., at the end of the adsorption test, π is only 5.95 mN m−1 for CH alone,40 while it rises to 9.06 mN m−1 for CH/GB complexes in this work). It indicates that the complexation results in CH/GB complex particles with higher surface activities, due to the combination of functional groups in CH and GB. By comparing kdiff, π0 and π9000 in Table 1, the improvement of surface activities induced by increasing pH can be appreciated quantitatively: e.g., pH increasing from 3.5 to 5.5 makes kdiff increase from 0.16 to 0.26 mN m−1 s−0.5, which implies a faster adsorption of molecules or colloidal particles at the oil/water interface; both π0 and π9000 increases from 0.22 to 2.03 mN m−1, and from 3.99 to 9.06 mN m−1, respectively.
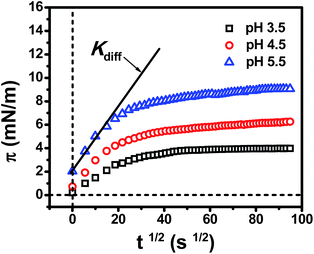 |
| Fig. 3 Time evolution of surface pressure for the adsorption of CH/GB complexes (1 wt%, CH : GB = 1 : 2, 3 days of age) at an oil/water interface for different pH values. | |
Table 1 Diffusion rate constants (kdiff) and surface pressure at the beginning (0 s; π0) and end (9000 s; π9000) of adsorption for CH/GB complexes (1 wt%, CH
:
GB = 1
:
2, 3 days of age) at an oil/water interfacea
pH |
kdiff (mN m−1 s−0.5) (LR) |
π0 (mN m−1) |
π9000 (mN m−1) |
LR = linear regression coefficient (in parenthesis). |
3.5 |
0.16 (0.997) |
0.22 |
3.99 |
4.5 |
0.21 (0.995) |
0.73 |
6.27 |
5.5 |
0.26 (0.984) |
2.03 |
9.06 |
Emulsifying capacity and emulsion stability
Fig. 4A shows the optical microscopy images of fresh emulsions prepared with CH/GB mixtures at various pH values, and the volume- and number-average droplet sizes are summarized in Fig. 4B. The formation of CH/GB complexes, either soluble (pH 4.5) or insoluble (pH 5.5 and 6.5), can benefit the formation of conventional and Pickering emulsions with a narrower distribution of droplet size; while at pH 3.5, the adsorption of CH and GB molecules also contributes to the formation of fine emulsion droplets, but large aggregations can be observed, which directly leads to an unstable emulsion system. The emulsifying properties of CH alone increases with pH, however, as indicated in Fig. S3 (ESI†), the emulsifying properties of GB are higher at lower pH (e.g., pH 3.5 and 4.5). The reason for droplet agglomeration at pH 3.5 is still unclear and would require more investigation to clarify it. By comparing the volume- and number-average diameter (dv and dn) as well as size distribution (dv/dn) of CH/GB-based emulsion droplets (Fig. 4B), it can be concluded that the emulsion capacity of CH/GB complexes can be improved by increasing pH, since a smaller droplet size (dv drops to 2.1 μm) and a narrower size distribution (dv/dn as low as 1.1) are obtained at the highest pH value. According to the ζ potential results displayed in Fig. 1A, it is reasonable to conclude that CH/GB complexes can be more compact as pH increases, due to the conformational contraction of the CH molecules. It will be easier for CH/GB complexes with a more compact structure to adsorb at the oil/water interface because of lower steric hindrance, and also to form a firmer coating layer around oil droplets. In addition, a slight flocculation can be observed at pH 6.5, which is probably due to the lower ζ potential value (11.2 mV) at this pH, at which the emulsion droplets tend to interact with each other because of lower electrostatic repulsion.
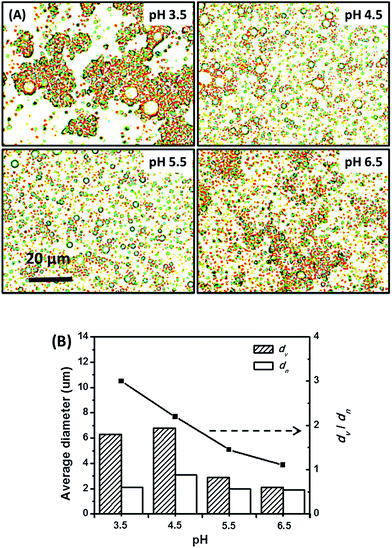 |
| Fig. 4 (A) Optical microscopy images of fresh emulsions prepared with CH/GB mixtures at different pH values (3.5–6.5). (B) Volume- and number-average diameter as well as size distribution of fresh CH/GB-based emulsion droplets. The concentration of CH/GB mixtures is 1 wt%, and the oil volume fraction is 0.1. | |
The freshly prepared CH/GB-based emulsions were kept at room temperature for one year and the stability was determined by visual observation of creaming and calculation of creaming index (CI). As indicated from Fig. 5A and B, the emulsion prepared with the CH/GB mixture at pH 3.5 is extremely unstable, and there is a large amount of serum layer even for the fresh one. For the other three pH values, the emulsions are homogeneous at least within the first 10 days according to the creaming index. Then, with an increase of storage time up to 20 days, the CI gradually increases to 62% for the CH/GB emulsion prepared at pH 4.5; and after one month, CI for pH 5.5 and 6.5 rises to 7% and 3%, respectively. Afterwards the CI was continuously recorded for up to 5 months but there was no further increase. Moreover, based on visual observations, there was no obvious change for all the pH values except that the surface of the emulsion turned to be yellowish after 8 months (data not shown), which might be due to the oxidation of the oil. Compared to CH-based emulsions40 and GB-based emulsions (Fig. S4, ESI†), the pH-induced evolution of CI for the CH/GB-stabilized emulsions is similar with that of CH, but the value of CI at a comparable pH is lower, especially at higher pH (e.g., at pH 5.5, the highest CI for CH/GB emulsions is just 7% in this work but it goes up to 60% for CH emulsions40). It suggests that the bonding of GB with CH molecules confers a higher surface activity to the CH/GB complexes and also a denser structure, thus favouring the formation of a stronger protecting layer around oil droplets and consequently a stable Pickering emulsion. Another point to note is that the long-term stability of the emulsion prepared at pH 6.5 is better than that at pH 5.5, possibly because of the denser structure of CH/GB at pH 6.5. It is interesting to point out that a gel-like emulsion is formed at pH 5.5 and 6.5 with an increase of storage time, as indicated from Fig. 5B, where the vials of emulsions prepared at different pH values and with 2 months of age are also displayed in a bottom-up position. In our previous work, it had been shown that CH/GB complex particles (formed at pH 5.5) tend to form a network structure after storage at room temperature for 20 days, and that the elasticity of the CH/GB gel continues to increase with time.16 Once these particles adsorb on the surface of oil droplets, they can benefit the formation of a network structure via particle–particle interactions combined to droplet–droplet contacts, thus resulting in a uniform Pickering emulsion gel. Furthermore, the formation of a gel-like structure among emulsion droplets can impart remarkable stability against coalescence and creaming of the emulsions. At last, it should be noted that the homogenization technique (HIU) used in this work is also an effective way to break insoluble CH/GB complexes into nanoscale particles (Fig. 2C), which can facilitate the adsorption of CH/GB complex particles at the oil/water interface.
 |
| Fig. 5 (A) Creaming index and photographs of vials (B) of emulsions prepared with CH/GB mixtures at different pH (3.5 to 6.5) and storage time (up to 5 months), with an oil volume fraction of 0.1. | |
One may question the role of free biopolymers (CH and GB) in the emulsification process. The long-term stability of the current emulsion systems at the highest pH (5.5 and 6.5) should be mainly due to the presence of CH/GB complexes, since either CH or GB alone confer limited emulsion stability as compared to the complexes40 (see also ESI Fig. S3 and S4†).
It is noted that other protein/polysaccharides complex particles have been used to produce Pickering emulsions. For example, Wang et al.41 reported on an antioxidant Pickering emulsion system with zein/chitosan complex particles. In another study, Shi et al.42 prepared Pickering emulsions stabilized by zein/chitosan colloidal particles, and edible films with excellent oxygen barrier properties were obtained. Compared to these studies, Pickering emulsions obtained with the CH/GB complexes exhibit very fine droplet size (below 2 μm) and long-term stability (up to 5 months); the properties of the emulsions (e.g., droplet size, stability) can also be readily controlled by simply adjusting the pH of the system. Moreover, as will be shown in the following section, a solid-like thermoreversible emulsion gel with tuneable viscoelasticity can be obtained with the CH/GB complex stabilized emulsion systems.
Rheological properties of CH/GB emulsions
Depending on oil volume fraction (φ), the rheological properties of emulsions generally vary from Newtonian for non-flocculated and dilute emulsions, to shear-thinning for concentrated and highly flocculated emulsions. To further examine the effect of oil volume fraction and pH on the microstructural properties of CH/GB emulsions, the rheological properties were investigated. As shown in Fig. 6, for all pH values, an increase of φ leads to an increase of both G′ and G′′, and G′ is higher than G′′ when φ reaches 0.4. As demonstrated in Knudsen's work, the possible reason should be attributed to the droplet packing effect, namely, the oil droplets are close enough to interact with each other in concentrated emulsions, and consequently, a network structure may build up among oil droplets.43 At a comparable φ, an increase of pH from 3.5 to 6.5 significantly increases the modulus, indicating a stronger inter-droplet interaction at higher pH, which is consistent with optical microscopy observations (Fig. 4A). At pH 5.5 and 6.5, with the increase of φ, G′ turns to be higher than G′′ for the whole test frequency range, implying the existence of an elastic gel-like emulsion. The difference in the moduli dependence on φ between low pH (pH 4.5) and high pH (pH 5.5 and 6.5) emulsions is mainly due to the difference in CH/GB particle interactions in the system. As shown in Fig. 2C, it is clear that the CH/GB particles formed at higher pH values have stronger attractive interactions than the lower pH ones, thus leading to more agglomerated particles. For example, for CH/GB complexes formed at pH 5.5 and 6.5, the hydrodynamic diameter increases significantly with time (day 1 to day 3) (e.g., from 33.89 to 459.25 nm for pH 5.5), while it just changes slightly for pH 4.5 (from 14.18 to 22.74 nm). Therefore, stronger inter-droplet interactions can be the result of CH/GB inter-particle interactions, especially when the CH/GB particles with higher attractive interactions are used to stabilize oil droplets.
 |
| Fig. 6 Moduli (G′ and G′′) of fresh emulsions prepared with CH/GB complexes at different pH (4.5 to 6.5) and oil volume fractions (0.2 to 0.4). | |
It has been reported that a relatively small temperature change can affect non-covalent interactions. Specifically, the bonding formed with the release of heat, like ionic bonding, hydrogen bonding and van der Waals forces can be weakened by increasing temperature, while hydrophobic interactions which are accompanied by heat absorption can be facilitated upon increasing temperature.44 Aiming to further identify the driving force for the formation of the droplet network structure, three successive heating and cooling cycles were carried out for CH/GB emulsions at pH 5.5 and 6.5 (φ = 0.4). At pH 5.5, as shown in Fig. 7A, an increase in temperature from 25 to 60 °C in the first cycle decreases both G′ and G′′, but the three-dimensional network formed by the flocculated droplets is still maintained. Then, a decrease of temperature from 60 to 5 °C continuously increases both G′ and G′′, and the increase rate of G′ is larger than for G′′, which indicates that a stronger network structure dominated by hydrogen bonding is formed at lower temperature. In the second and third heating and cooling cycles, a similar rheological behaviour is observed. Interestingly, at pH 6.5 (Fig. 7B), the rheological response to temperature is different with that at pH 5.5. In the first temperature sweep cycle, the heating process increases both G′ and G′′, while the cooling process shows a negligible effect on modulus. In the second and third cycles, the curves are almost the same. By comparing Fig. 7A and B, it can be concluded that the emulsion gel formed at pH 5.5 and 6.5 are both thermoreversible within the test temperature range. However their different responses to temperature suggest that hydrophobic interactions contribute more in the formation of the emulsion gel in the case of pH 6.5, which could be attributed to the differences in the structural properties of CH/GB complex particles formed at pH 5.5 and 6.5, respectively.
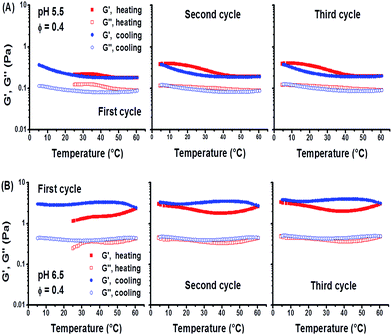 |
| Fig. 7 Temperature sweep (three cycles) of fresh CH/GB-based emulsions prepared at pH 5.5 (A) and 6.5 (B), at an oil volume fraction (φ) of 0.4. | |
Fig. 8 presents the schematic mechanism of the formation of CH/GB-based Pickering emulsion gels at pH values of 5.5 and 6.5. The adsorption of insoluble CH/GB complexes formed at pH 5.5 and 6.5 on the surface of oil droplets allow forming a protective coating layer around oil droplets and benefits the formation of a network structure via particle–particle interactions combined to droplet–droplet contacts, and thus results in a Pickering emulsion gel with considerable long-term stability. However, the pH-induced difference in the properties of CH/GB complex particles (e.g., charge density and conformational structure) directly determines the emulsification and stabilization performance. As compared to pH 5.5, the CH/GB complex particles formed at pH 6.5 have lower charge density (zeta potential 11.2 mV vs. 16.4 mV), which gives them a higher tendency to interact with each other, leading to flocculation and thus facilitating the development of the gel-like structure. Moreover, the molecular structure of CH is more compact at pH 6.5 due to less electrostatic repulsion, therefore the conformation of the resulting complexes should be denser than those formed at pH 5.5. The adsorption of the more compact complex particles (at pH 6.5) on the surface of the oil droplets provides a stronger protection against coalescence and creaming. Both factors discussed above contribute to the long-term stability of the emulsions prepared at pH 6.5.
 |
| Fig. 8 Schematic representation of the formation of Pickering emulsion gels stabilized with CH/GB complexes at pH 5.5 and 6.5. As compared to at pH 5.5, the CH/GB complex particles formed at pH 6.5 have lower charge density and more compact conformation structure. | |
Conclusions
In this study, the effects of pH and storage time on the properties of CH/GB complexes, including charge density, hydrodynamic size, microstructure and interfacial tension were investigated. The emulsification and stabilization properties of the CH/GB complex particles were also examined in oil/water systems. It was found that the formation and properties of CH/GB complexes greatly depend on pH and storage time: insoluble CH/GB complexes were only formed in the oppositely charged pH range (5 < pH < 6.5), and the size of insoluble CH/GB complex particles increased with time. The emulsions stabilized with insoluble CH/GB complexes showed a smaller droplet size and a better long-term stability when compared to soluble complexes, due to the stronger protecting barriers at the oil/water interface. For CH/GB complexes prepared at pH 5.5 and 6.5, the difference in charge density and conformational structure also resulted in corresponding emulsions with different creaming rates, flocculation and gelling properties. Our results suggest that CH/GB insoluble complexes can be used as effective pH-controlled Pickering emulsifiers and stabilizers, for the production of surfactant-free and bio-based O/W emulsions for food and non-food applications. This work might also inspire further developments in exploiting biocompatible Pickering emulsifiers based on the electrostatic complexation of proteins and polysaccharides.
Acknowledgements
This research work has been funded by NSERC (Natural Sciences and Engineering Research Council of Canada). A scholarship for Ms Wang provided by the China Scholarship Council (CSC) is also gratefully acknowledged.
Notes and references
- T. N. Hunter, R. J. Pugh, G. V. Franks and G. J. Jameson, Adv. Colloid Interface Sci., 2008, 137, 57–81 CrossRef CAS PubMed.
- Y. Chevalier and M.-A. Bolzinger, Colloids Surf., A, 2013, 439, 23–34 CrossRef CAS.
- E. Dickinson, Curr. Opin. Colloid Interface Sci., 2010, 15, 40–49 CrossRef CAS.
- Y. Zhu, J. Jiang, K. Liu, Z. Cui and B. P. Binks, Langmuir, 2015, 31, 3301–3307 CrossRef CAS PubMed.
- S. Melle, M. Lask and G. G. Fuller, Langmuir, 2005, 21, 2158–2162 CrossRef CAS PubMed.
- B. P. Binks and J. H. Clint, Langmuir, 2002, 18, 1270–1273 CrossRef CAS.
- M. V. Tzoumaki, T. Moschakis, V. Kiosseoglou and C. G. Biliaderis, Food Hydrocolloids, 2011, 25, 1521–1529 CrossRef CAS.
- H. Liu, Z. J. Wei, M. Hu, Y. H. Deng, Z. Tong and C. Y. Wang, RSC Adv., 2014, 4, 29344–29351 RSC.
- H. Liu, C. Wang, S. Zou, Z. Wei and Z. Tong, Langmuir, 2012, 28, 11017–11024 CrossRef CAS PubMed.
- A. Yusoff and B. S. Murray, Food Hydrocolloids, 2011, 25, 42–55 CrossRef CAS.
- Z. Wei, Y. Yang, R. Yang and C. Wang, Green Chem., 2012, 14, 3230–3236 RSC.
- I. Kalashnikova, H. Bizot, B. Cathala and I. Capron, Langmuir, 2011, 27, 7471–7479 CrossRef CAS PubMed.
- J. W. J. de Folter, M. W. M. van Ruijven and K. P. Velikov, Soft Matter, 2012, 8, 6807–6815 RSC.
- M. Rinaudo, Prog. Polym. Sci., 2006, 31, 603–632 CrossRef CAS.
- A. A. Karim and R. Bhat, Food Hydrocolloids, 2009, 23, 563–576 CrossRef CAS.
- X.-Y. Wang, C.-S. Wang and M.-C. Heuzey, Int. J. Polym. Mater. Polym. Biomater., 2016, 65, 96–104 CrossRef CAS.
- S. L. Turgeon, M. Beaulieu, C. Schmitt and C. Sanchez, Curr. Opin. Colloid Interface Sci., 2003, 8, 401–414 CrossRef CAS.
- S. L. Turgeon, C. Schmitt and C. Sanchez, Curr. Opin. Colloid Interface Sci., 2007, 12, 166–178 CrossRef CAS.
- C. L. Cooper, P. L. Dubin, A. B. Kayitmazer and S. Turksen, Curr. Opin. Colloid Interface Sci., 2005, 10, 52–78 CrossRef CAS.
- S. A. Fioramonti, A. A. Perez, E. Elena Aringoli, A. C. Rubiolo and L. G. Santiago, Food Hydrocolloids, 2014, 35, 129–136 CrossRef CAS.
- C. M. R. Rocha, H. K. S. Souza, N. F. Magalhaes, C. T. Andrade and M. P. Goncalves, Carbohydr. Polym., 2014, 110, 345–353 CrossRef CAS PubMed.
- X. Ding and P. Yao, Langmuir, 2013, 29, 8636–8644 CrossRef CAS PubMed.
- C. G. de Kruif, F. Weinbreck and R. de Vries, Curr. Opin. Colloid Interface Sci., 2004, 9, 340–349 CrossRef CAS.
- M. Evans, I. Ratcliffe and P. A. Williams, Curr. Opin. Colloid Interface Sci., 2013, 18, 272–282 CrossRef CAS.
- A. Benichou, A. Aserin and N. Garti, J. Dispersion Sci. Technol., 2002, 23, 93–123 CrossRef CAS.
- E. Dickinson, Soft Matter, 2008, 4, 932–942 RSC.
- B. Yin, W. Deng, K. Xu, L. Huang and P. Yao, J. Colloid Interface Sci., 2012, 380, 51–59 CrossRef CAS PubMed.
- S. Haider, W. A. Al-Masry, N. Bukhari and M. Javid, Polym. Eng. Sci., 2010, 50, 1887–1893 CAS.
- W. Xia, W. Liu, L. Cui, Y. Liu, W. Zhong, D. Liu, J. Wu, K. Chua and Y. Cao, J. Biomed. Mater. Res., Part B, 2004, 71, 373–380 CrossRef PubMed.
- F. Shen, Y. L. Cui, L. F. Yang, K. D. Yao, X. H. Dong, W. Y. Jia and H. D. Shi, Polym. Int., 2000, 49, 1596–1599 CrossRef CAS.
- J. Mao, S. Kondu, H.-F. Ji and M. J. McShane, Biotechnol. Bioeng., 2006, 95, 333–341 CrossRef CAS PubMed.
- N. G. Voron'ko, S. R. Derkach, Y. A. Kuchina and N. I. Sokolan, Carbohydr. Polym., 2016, 138, 265–272 CrossRef PubMed.
- S. Ogawa, E. A. Decker and D. J. McClements, J. Agric. Food Chem., 2003, 51, 2806–2812 CrossRef CAS PubMed.
- M. Jumaa, F. H. Furkert and B. W. Muller, Eur. J. Pharm. Biopharm., 2002, 53, 115–123 CrossRef CAS PubMed.
- C. Sanchez, G. Mekhloufi, C. Schmitt, D. Renard, P. Robert, C. M. Lehr, A. Lamprecht and J. Hardy, Langmuir, 2002, 18, 10323–10333 CrossRef CAS.
- A. Lamprecht, U. Schafer and C. M. Lehr, AAPS PharmSciTech, 2000, 1, E17 CrossRef CAS PubMed.
- Y. Zhang, E. Yildirim, H. S. Antila, L. D. Valenzuela, M. Sammalkorpi and J. L. Lutkenhaus, Soft Matter, 2015, 11, 7392–7401 RSC.
- H. Dautzenberg and N. Karibyants, Macromol. Chem. Phys., 1999, 200, 118–125 CrossRef CAS.
- E. Colombo, F. Cavalieri and M. Ashokkumar, ACS Appl. Mater. Interfaces, 2015, 7, 12972–12980 CAS.
- X. Y. Wang and M. C. Heuzey, Langmuir, 2016, 32, 929–936 CrossRef CAS PubMed.
- L. J. Wang, Y. Q. Hu, S. W. Yin, X. Q. Yang, F. R. Lai and S. Q. Wang, J. Agric. Food Chem., 2015, 63, 2514–2524 CrossRef CAS PubMed.
- W. J. Shi, C. H. Tang, S. W. Yin, Y. Yin, X. Q. Yang, L. Y. Wu and Z. G. Zhao, Food Hydrocolloids, 2016, 52, 253–264 CrossRef CAS.
- J. C. Knudsen, L. H. Ogendal and L. H. Skibsted, Langmuir, 2008, 24, 2603–2610 CrossRef CAS PubMed.
- U. Klinkesorn, P. Sophanodora, P. Chinachoti, E. A. Decker and D. J. McClements, Food Hydrocolloids, 2005, 19, 1044–1053 CrossRef CAS.
Footnote |
† Electronic supplementary information (ESI) available: Visual observation of CH/GB complexes; visual observation of HIU-treated CH/GB complexes; optical microscopy images of fresh emulsions prepared with GB; creaming index of GB-based emulsions. See DOI: 10.1039/c6ra10378b |
|
This journal is © The Royal Society of Chemistry 2016 |
Click here to see how this site uses Cookies. View our privacy policy here.