DOI:
10.1039/C6RA09717K
(Paper)
RSC Adv., 2016,
6, 66595-66608
Design and synthesis of novel dual-target agents for HDAC1 and CK2 inhibition†
Received
14th April 2016
, Accepted 1st July 2016
First published on 6th July 2016
Abstract
Drug entities able to address multiple targets can be more effective than those directed to just one biological target. We disclose herein a series of novel dual inhibitors to target histone deacetylase 1 (HDAC 1) and protein kinase CK2. Our bifunctional compounds combine two complementary chemo-active prototypical scaffolds: a hydroxamate essential for the chelation of the zinc ion present in the active site of HDAC (Zinc Binding Group), and a 4,5,6,7-tetrabromobenzotriazole (TBB) moiety introduced to interact with the ATP binding site in CK2 and to act simultaneously as the cap group in the interaction with HDAC1. The synthesized dual-acting agents exhibited promising inhibitory activities towards HDAC1 and CK2. The best result was obtained for 5c with an IC50 of 5 μM for both enzymes. However, its N-2 substituted isomer 5e presented the best profile in cell-based assays, with cytotoxic activity in the low micromolar LC50 in two mammalian cancer cell lines and 4-fold less activity towards a pseudonormal mammalian cell line. Furthermore, this hybrid molecule induced apoptosis in leukemia cells in a concentration-dependent manner. All together this makes 5e a promising lead compound for future in vivo assays in animal tumor models.
Introduction
Owing to the wide-range importance of chemotherapy as the primary treatment of choice for most cancer cases, the development of new efficient drug entities represents a challenge. To date, significant progress has been made in this area, although most traditional cytotoxic agents are associated with severe toxicities and other undesirable side effects. More recently, combination cancer therapies have expanded the treatment options, including the use of multiple drugs with different pharmacological targets, which can directly achieve the desirable therapeutic outcome concertedly via different mechanisms of action.1–3 However, the employment of these multi-component drug cocktails can also introduce adverse effects related to pharmacokinetics, unpredictable drug–drug interactions, toxicity and patient incompliance.4,5 To address these limitations, an attractive and more elegant strategy in modern cancer medicine is to design a single drug with efficient inhibitory activity for more than one biological target, while maintaining lower side effects.6–8 In addition, from an efficacy perspective, as well as in terms of cost reduction, the design of multitarget drugs instead of discrete inhibitors is also of increasing interest and prominence.9,10 In recent years, the development of dual inhibitors has attracted a great deal of attention among several research groups and successful examples have been published for the treatment of cancer, psychiatric pathologies, inflammation, diabetes and neurodegenerative diseases.11,12
Histone deacetylases (HDACs) are a class of epigenetic enzymes that have generated much interest in cancer therapy, since their aberrant activity has been linked to cell proliferation in a variety of human diseases, most notably myeloid neoplasia and solid tumors.13–18 These enzymes catalyze the hydrolysis of acetylated lysine side chains in histone and non-histone proteins for transcriptional regulation, cell cycle progression, and apoptosis.19,20 There are 18 human HDAC isoforms subdivided into four different classes (I–IV), among which classes I (1, 2, 3 and 8), II (4–7, 9 and 10), and IV (11) are Zn2+-dependent for enzymatic activity. Class I HDACs are ubiquitously expressed and play essential roles in proliferation, while classes II and IV have tissue-specific functions.21,22 The maintenance of equilibrium between acetylation and deacetylation of histones and nonhistone substrates is essential for normal cell growth. As a result, the use of HDAC inhibitors (HDACi) as cancer therapeutics has emerged as an area of active investigation.23,24 By inducing histone hyperacetylation, these compounds alter gene transcription and can exert antitumor effects through growth arrest, differentiation and/or apoptosis.25,26 HDAC inhibition has been consolidated as a strategy in epigenetic drug discovery, with 12 inhibitors currently in clinical trials and the FDA approvals of vorinostat (SAHA)27 and romidepsin (FK228)28 for treatment of cutaneous T-cell lymphoma (Fig. 1A).
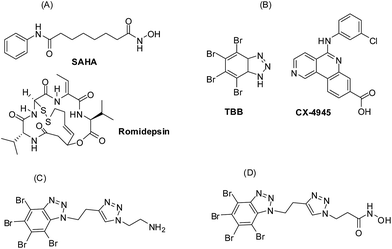 |
| Fig. 1 Representative examples of (A) HDAC, (B) CK2 inhibitors, (C) “clicked” CK2 inhibitors, and (D) chemical structure of one of the designed dual inhibitors. | |
The use of HDACi in combination with other anticancer agents has become a practical method to increase the efficiencies of these agents. There are a few examples of this subtype of bifunctional HDACi derived compounds as therapeutically viable anticancer agents.29–35 One particularly promising approach is the modulation of receptor tyrosine kinase (RTK) pathways by the action of HDACs inhibitors, leading to potential synergistic effects.36,37 In this context, an attractive starting point for a secondary target is protein kinase CK2, which is not only responsible for the regulation of HDACs by post-translational modification (particularly phosphorylation), but also appears to be involved in HDAC activation in hypoxia-associated cancer processes, thus contributing to tumor angiogenesis.38–40
CK2 is a ubiquitously expressed, constitutively active and highly conserved protein serine/threonine kinase essential for cell viability, which is composed of tetrameric complexes consisting of two catalytic subunits (CK2α, CK2α′) and a dimer of regulatory β subunits.41,42 CK2 is a pleiotropic protein kinase with more than 300 substrates, which are localized in different cell compartments and are implicated in various important functions, including gene expression, cell growth and differentiation, signal transduction and apoptosis.43,44 It has been recently demonstrated that CK2 plays an important role in many pathologies and abnormal high levels of its enzymatic activity have been found in a large variety of cancer cells.45 Its proliferative and anti-apoptotic properties create a favourable cellular environment for tumor maintenance and progression, making it a potential target for cancer treatment.46 Up to date many compounds have been reported as potent CK2 inhibitors.47,48 Among the CK2 inhibitors reported so far, ATP-competitive compounds such as coumarin derivatives, ellagic acid, DMAT or TBB stand out for their high efficiency and availability.49–52 More recently, the benzonaphthyridine derivative CX-4945 has been reported to be a first-in-class, orally bioavailable selective CK2 inhibitor in clinical trials (Fig. 1B).53,54 Hence, since both CK2 and HDAC are closely related relevant proteins in cancer therapy, we envisioned that concurrent inhibition of these two enzymes, by the design of a novel potential multi-target single molecule, might enhance drug efficacy and overcome the current pharmacokinetic limitations, as well as likely provide beneficial additive or synergistic biological effects. Herein we describe our efforts toward this goal by reporting a new family of dual inhibitors of CK2 and HDAC1. These chimeric compounds contain a TBB moiety as a capping group and a hydroxamic acid as a zinc-binding group (ZBG), which have been linked using a click chemistry approach (Fig. 1D).
Results and discussion
Design rationale
HDACis can be broadly described by a common pharmacophore, consisting of a cap group, an appropriate linker and a metal-binding moiety (ZBG), being the latter the primary affinity determinant that coordinates to the active site Zn2+ ion (Fig. 2A). Among the HDACis, hydroxamic acids exhibit the most potent efficacy since, after ionization, an exceptionally stable 5-membered ring chelate with the active site Zn2+ ion is created.
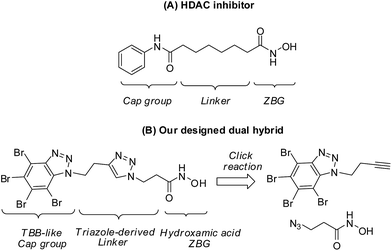 |
| Fig. 2 (A) Prototypical structure of HDACi, as exemplified by SAHA. (B) Our click chemistry approach toward bifunctional inhibitors. | |
We postulated that appropriate conjugation of the surface recognition group (cap) of a prototypical HDACi to other hydrophobic antitumor pharmacophore (e.g., TBB) could furnish the desired bifunctional agents. Presumably, the TBB moiety would act as a surrogate of the cap group in the HDACi and also maintain its activity against the CK2 protein (Fig. 2B). This assumption is supported by our previous results,55 where we described some “clicked” TBB derivatives in which the terminal group is an amino group instead of the hydroxamate present in these new dual-targeting compounds. Those compounds (see Fig. 1C) showed Ki values in the low μM range and high degree of selectivity against a panel of 24 kinases.
Chemistry
A series of HDAC-CK2 dual inhibitors has been synthesized. Our approach involves a Cu-catalyzed azide–alkyne 1,3-dipolar cycloaddition (CuAAC)56 as the crucial step to couple both CK2 and HDAC inhibition scaffolds. As shown in Fig. 2B, the designed inhibitor has a triazole-alkyl linker, resulting of the click connection of the azide bearing the ZBG and the corresponding TBB-alkyne moiety.
For any synthetic method to be useful, the substrates must be readily accessible. As depicted in Scheme 1, alkyne reagents 1 and 2 were prepared by alkylation of TBB with the corresponding alkynyl bromide in the presence of K2CO3.57,58 While derivative 1 was obtained as a single product after the reaction with propargyl bromide, the presence of an extra carbon in the bromide reagent resulted in the formation of both N-1 (2a) and N-2 (2b) alkylated isomers. Fortunately, they were successfully isolated by column chromatography and further tested separately to evaluate the influence of the N-substitution pattern in the inhibitor structure.
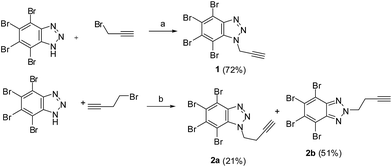 |
| Scheme 1 Reagents: (a) K2CO3, acetone, MW, 150 °C, 1 min; (b) K2CO3, CH3CN, RT, 24 h. | |
Likewise, azides with the hydroxamate function were easily obtained in two steps from the corresponding bromo-derived carboxylic acids (Scheme 2). Thus, the synthesis of the intermediates 3a–d was accomplished by standard azide formation starting from the corresponding bromide. Further reaction of the acid group with O-THP hydroxylamine by EDC coupling led to the desired aliphatic and aromatic azides 4a–d.
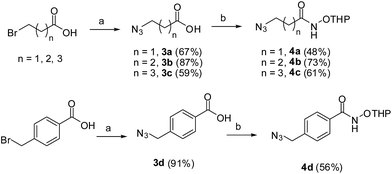 |
| Scheme 2 Reagents: (a) NaN3, DMF, 60 °C, 12 h; (b) NH2OTHP, HOBt, EDCI, NMM, DMF, rt, 12 h. | |
To examine the effect of the linker length on both sides of the triazole ring, TBB derivatives 1 and 2a–b as well as azides 4a–c were selected for the click reaction.
The CuAAC reaction is a regioselective process, forming exclusively the 1,4-substituted adduct.59 Accordingly, we found that only one isomer was obtained, thus achieving the triazole-based conjugates in excellent yields (Scheme 3). We identified the use of CuSO4/Na-ascorbate catalytic system and DMF as the optimized conditions for the cycloaddition step. Subsequent removal of the THP protecting group furnished the final hydroxamic acid-based dual inhibitors 5a–f.
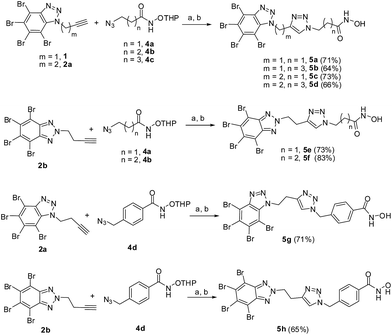 |
| Scheme 3 Reagents: (a) CuSO4 (10 mol%), Na-ascorbate (30 mol%), DMF, rt, 24 h; (b) 4 N HCl in dioxane, MeOH, rt, 2 h. | |
The influence of the position of the attachment (N-1 or N-2) of the linker to the TBB moiety was analyzed by preparing a representative set of N-1 and N-2 alkyl derivatives (5a–f).
Compounds 5g–h were selected to study the influence of the introduction of an aromatic ring attached to the hydroxamate group on the inhibition of the target proteins.
Biological evaluation
The inhibitory activities of the synthesized compounds were determined against both enzymes CK2 and HDAC1. The percentage of inhibition at 50 μM concentration of the tested compound is collected in Table 1. The IC50 for those compounds showing an inhibition higher than 75% was calculated, and the values are depicted also in Table 1.
Table 1 Percentage of inhibition of 5a–h for CK2α and HDAC1 at 50 μM. (IC50 for compounds with an inhibition higher than 75% at 50 μM)
Compound |
% inhibition CK2α,a 50 μM (IC50, μM) |
% inhibition HDAC1,a 50 μM (IC50, μM) |
Enzymatic data are mean values from at least two independent experiments ± SD. |
5a |
22.7 |
32.2 |
5b |
42.0 |
3.5 |
5c |
79.0 (5.4 ± 1.2) |
90.0 (5.0 ± 1.8) |
5d |
61.2 |
65.2 |
5e |
61.6 |
97.0 (2.2 ± 0.2) |
5f |
47.7 |
88.3 (5.1 ± 2.4) |
5g |
47.2 |
99.0 (1.6 ± 0.4) |
5h |
22.2 |
86.0 (2.9 ± 1.1) |
TBB |
99.8 (0.8 ± 0.1) |
— |
SAHA |
— |
25.8 (at 0.1 μM) |
The activity towards human recombinant HDAC1 was tested using a fluorimetric substrate. Compounds 5c, 5e and 5f presented the highest potency within the compounds with aliphatic linkers, with IC50 of 5.0 ± 1.8 μM, 2.2 ± 0.2 μM and 5.1 ± 2.4 μM respectively (inhibitory activities between 88 and 97% at 50 μM) while 5d inhibited the deacetylase activity at a lower extent (65.2% at 50 μM) and weak or no inhibition was observed for compounds 5a and 5b in the same conditions.
The introduction of an aromatic ring in the linker was beneficial for the inhibition of HDAC1, and 5g displayed the highest HDAC1 inhibition of all tested compounds with an IC50 of 1.6 ± 1.1 μM.
The inhibitory activities of these compounds for CK2 were measured using a radiometric method with a short peptide (RRRADDSDDDDD) as a substrate. Recombinant human CK2α was used as a source of CK2. The best potency against CKα was found for compound 5c (79% of inhibition at 50 μM, IC50 of 5.4 ± 1.2 μM), which bears the linker attached to the N-1 position of TBB and two CH2 groups connecting the triazole ring to both TBB and the ZBG. The distance between TBB and the triazole ring provided by a 2(CH2) linker seems to be crucial for activity. Thus, compounds 5a and 5b, where only one methylene group is present at this position are weaker inhibitors (22.7% of inhibition for 5a; 42% for 5b). However, more than 60% of inhibition at 50 μM was maintained for compound 5d, which differ from 5b in the presence of one additional CH2 group at this position.
The effect of changing the position of attachment of the alkyl linkers to the N-2 position of TBB was examined through compounds 5e–f. Again the best activity was obtained for the compound bearing 2(CH2) linkers at both sides of the connecting triazole (compound 5e, 61.6% of inhibition at 50 μM), while an increase in the number of CH2 groups, such as in 5f brought about a loss of activity.
To study the influence of the introduction of an aromatic ring in the linker, compounds 5g–h were synthesized. Both compounds showed a significant loss of activity (47.2% of inhibition for 5g and 22.2% of inhibition for 5h) when compared to its non-aromatic analogues 5d and 5f of similar chain length. This loss of activity is probably due to an increase on the rigidity of the molecule.
In summary, the described compounds have a potential interest as dual target inhibitors, and compound 5c presents the best profile, with identical IC50 values in the low micromolar range for CK2 and HDAC1. Therefore, it was selected to evaluate its antiproliferative effect on several cancer cell lines, and proapoptotic activity towards human Jurkat T-leukemia and MCF-7 breast adenocarcinoma. We selected also compound 5e as representative of the N-2 substituted derivatives.
Cytotoxic activity of the selected inhibitors was evaluated in vitro and the LC50 index, calculated as concentration of drug which kills 50% cells in comparison to control, was used to express the effective inhibition. Both compounds exhibited cytotoxic activity in the micromolar range towards several mammalian tumor cell lines (Fig. 3).
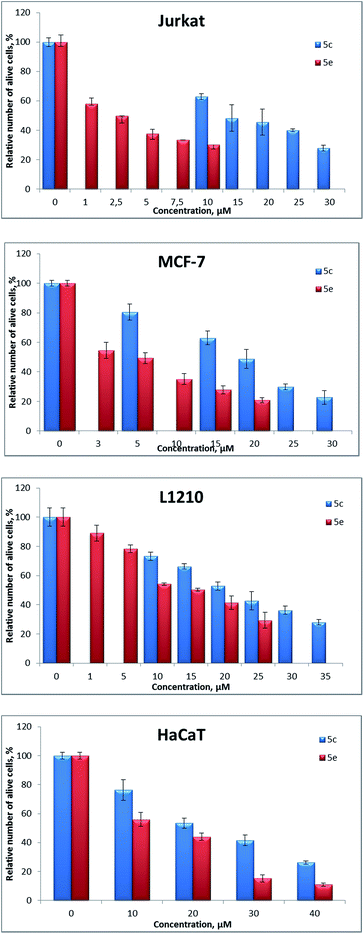 |
| Fig. 3 Cytotoxic effect of dual CK2/HDAC inhibitors 5c and 5e towards human T-leukemia cells of Jurkat line, human breast adenocarcinoma cells of MCF-7 line, murine leukemia cells of L1210 line, and human immortalized keratinocytes of HaCat line, after 24 h of incubation. | |
Interestingly, 5e showed 1.6–4.7 fold higher activities compared to its isomer 5c. Human leukemia cells of Jurkat line (LC50 = 2.9 μM) and human breast adenocarcinoma cells of MCF-7 line (LC50 = 4.26 μM) were the most sensitive to 5e action, while human immortalized keratinocytes of HaCat cells were found to be 3-fold more resistant to this compound (LC50 = 13.66 μM), indicating a selectivity of action of this dual CK2/HDAC inhibitor towards tumor cells. Its isomeric compound 5c demonstrated weaker cytotoxic activity towards all studied cell lines (LC50 in the range of 13.59–27.81 μM), and lack of selectivity for tumor against pseudonormal mammalian cell lines (Table 2).
Table 2 Comparison of LC50 values of dual CK2/HDAC inhibitors 5c and 5e towards several mammalian cell lines
Cell line |
Origin |
5c, LC50, μM |
5e, LC50, μM |
5c : 5e |
Jurkat |
Human leukemia |
13.59 ± 1.04 |
2.87 ± 1.14 |
4.74 |
L1210 |
Murine leukemia |
20.90 ± 1.03 |
13.09 ± 1.07 |
1.60 |
MCF-7 |
Human breast adenocarcinoma |
17.27 ± 1.07 |
4.26 ± 1.09 |
4.05 |
293T |
Human embryonic kidney cells, transfected by SV40 virus |
27.81 ± 1.05 |
14.55 ± 1.11 |
1.91 |
HaCat |
Human immortalized keratinocytes |
22.06 ± 1.05 |
13.66 ± 1.08 |
1.61 |
Three main cell death mechanisms have been identified – apoptosis, autophagy, and necrosis.60 Cell necrosis is an undesirable phenomenon during chemotherapy treatment, since it results in the development of massive inflammatory processes in the organism of cancer patients which can significantly complicate the treatment process. By contrast, the induction of apoptosis by anticancer drugs leads to quick phagocytosis of remnants of tumor cells by the macrophages which considerably diminish the development of side effects of chemotherapy.
Cytomorphologic studies were performed to identify by which way – apoptotic or necrotic – our dual CK2-HDAC inhibitors induced the death of target cells. Phosphatydylserine translocation to the external layer of the cell membrane of dying cells, measured by the Annexin V test, is considered one of the earliest hallmarks of apoptosis.61 Propidium iodide is a DNA targeting dye which detects only necrotic cells, since alive cells use membrane pumps to move it out to the extracellular medium. Supravital double staining with FITC-conjugated Annexin V and propidium iodide of Jurkat T-leukemia cells treated with 5c and 5e in LC50 and LC75 doses, revealed that both compounds induced apoptosis in leukemia cells (Fig. 4). It should be stressed that the rate and intensity of apoptosis under the action of these novel dual CK2/HDAC inhibitors increased in a concentration-dependent manner. In particular, the treatment at LC75 concentration led to a proportional increase in the number of annexin V positive, PI-negative (early apoptotic cells) compared to their action at LC50 (Fig. 4). On the other hand, inhibitor 5e led to induction of apoptosis at lower concentration (3 μM for LC50 and 10 μM for LC75) than 5c, which was able to induce appearance of comparable percent of annexin V+/PI− cells only at significantly higher concentrations (15 μM for LC50 and 30 μM for LC75, respectively). This result is in accordance with the higher cytotoxic activity displayed by 5e in this tumor cell line. Cytotoxic and proapoptotic activities of 5c and 5e were compared to the ones of TBB and SAHA in human Jurkat T-leukemia cells (ESI Fig. S1†). Interestingly 5e possesses significantly higher activity (LC50 = 2.5 μM) than TBB (LC50 = 20 μM) and, although LC50 of 5e is identical to that of SAHA, its proapoptotic activity is significantly higher, as revealed by quantitative annexin V/PI assay by flow cytometry. Thus, dual CK2-HDA1C inhibitor 5e demonstrated an improved behavior in cell-based antitumor assays compared to its predecessors TBB and SAHA, demonstrating the interest of this type of dual targeting inhibitors.
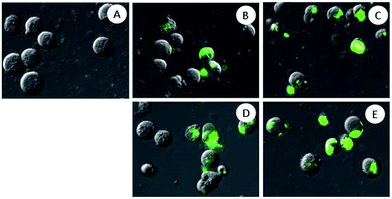 |
| Fig. 4 Proapoptotic activity of dual CK2/HDAC inhibitors (Jurkat T-leukemia cells, combined annexin V/PI staining, 24 h). (A) Control, (B) 5c (15 μM), (C) 5c (30 μM), (D) 5f (3 μM), (E) 5f (10 μM). Green color – FITC-conjugated annexin V, orange color – propidium iodide. | |
Quantitative annexin V/PI assay by flow cytometry showed that both 5c and 5e in sub-LC75 doses (15–25 μM for 5c and 10 μM for 5e) led to significant and almost identical increase (24–25%) in population of late apoptotic cells (AnV(+)/PI(+)). However, cell treatment with 5c was accompanied by the appearance of a higher number of necrotic cells (12–18% AnV(−)/PI(+)) compared to 5e (9.5%), and the same dependency was also observed in previous experiment (see Fig. 4). Thus, 5e induces mostly late apoptosis in Jurkat T-leukemia cells, while 5c, besides having weaker cytotoxic activity, is inducing both necrosis and late apoptosis in these cells, which is considered harmful on the physiological level. Surprisingly, both 5c and 5e demonstrated much higher proapoptotic activity compared to TBB (8.5% late apoptotic cells) and SAHA (18% late apoptotic cells), also taken in sub-LC75 concentrations (Fig. 5). These results clearly demonstrate better proapoptotic activities of novel hybrid CK2-HDAC1 inhibitors compared to their parental compounds.
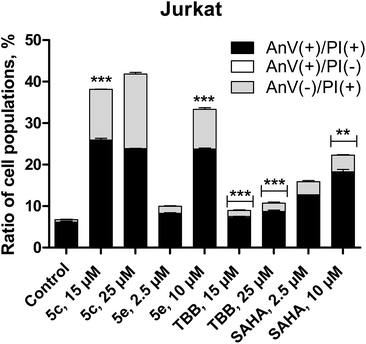 |
| Fig. 5 Comparison of proapoptotic activity of compounds 5c, 5e, TBB and SAHA towards human Jurkat T-leukemia cells. After a 24 h exposure cells were stained with FITC-Annexin V and PI for further analysis by flow cytometry. ***p < 0.001 relative to control, unpaired t-test, **p < 0.01 relative to 5e, 10 μM, unpaired t-test, **p < 0.001 relative to 5e, 10 μM, unpaired t-test. | |
Nuclear chromatine hypercondensation is considered to be another typical hallmark of apoptosis. It can be easily measured by using DNA-intercalating dyes, such as DAPI (4′,6-diamidino-2-phenylindole). Both 5c and 5e led to chromatine hypercondensation, vacuolization of cytosol and formation of membrane blebs at 25 μM and 10 μM concentrations (Fig. 6), which correspond to apoptosis induction. These results fully confirm the annexin V/PI data.
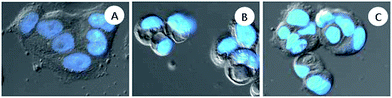 |
| Fig. 6 Cytomorphological studies of chromatin structure of MCF-7 cells under treatment with dual CK2/HDAC inhibitors. DAPI staining, 24 h. (A) Control, (B) 5c (25 μM), (C) 5e (10 μM). | |
Computational studies
In order to give a rationale for the results shown in Table 1, the predicted binding-mode of the described compounds to both enzymes was studied by means of molecular modeling techniques.
HDAC1 binding. The catalytic site of HDAC1 is located at the centre of the enzyme and is accessed through a small hydrophobic tunnel. The side chains of Phe150 and Phe205 leave a gap of approximately 8 Å between them making up this tunnel, at the bottom of which lays the catalytic Zn2+ ion. In normal conditions, the acetylated Lys of the substrate plunges into the tunnel reaching the Zn2+ and becomes part of the coordination sphere through the carbonyl oxygen, which catalyses the deacetylation reaction with the participation of a water molecule, as can be seen in the crystal structure of HDAC8 in complex with a substrate-like molecule (ESI Fig. S1†).62 HDAC inhibitors carrying a ZBG target the enzyme by chelating the Zn2+ and becoming part of its coordination sphere, while the linker interacts with the walls of the tunnel and the capping moiety protrudes out of it and interacts with the surface of the protein.63,64 Crystallographic studies have shown that hydroxamic acid-bearing inhibitors establish a hydrogen bonding network between conserved His residues in the active site and coordinate Zn2+ in a bidentate way, replacing the active-site water molecule with one of the oxygen atoms.62 Moreover, computational studies suggested that one of the His residues in the binding site could be protonated as part of the deacetylation reaction mechanism.65 In this same line, the binding poses obtained for the set of our newly synthesized inhibitors buried the hydroxamic acid moiety deep into the active site, coordinating the two oxygen atoms with the catalytic Zn2+ ion. Apart from chelating the Zn2+, the hydroxamic moiety established several hydrogen bond interactions, and we observed that the terminal OH could be easily deprotonated by the imidaloze side chain of His140. Therefore the dockings and further calculations were carried out with the deprotonated hydroxamates and a protonated side chain of His140. In addition to the hydrogen bond resulting from this deprotonation, the NH group of the hydroxamate interacts with the Nε of the imidaloze side chain of His141, and the carbonyl oxygen with the OH group on the side chain of Tyr303. The linker between the hydroxamate and the triazole can establish van der Waals interactions with Phe150 and Phe205, making the TBB cap protrude out of the binding tunnel. A linker of two or three methylene groups between the hydroxamate and the triazole keep the latter inside the tunnel and at van der Waals interaction reach of the side chains of Phe150 and Phe205 as it’s the case for compounds 5a, 5c and 5e–f, whereas 5b and 5d that present four methylene groups make the triazole protrude out of the binding tunnel.The introduction of an aromatic moiety to the linker between the hydroxamate and the triazole in compounds 5g–h increases the interaction with the side chains of Phe150 and Phe205 due to the establishment of π–π interactions between the aromatic groups. On the other hand, a shorter linker with only one methylene group between the TBB moiety and the triazole present in compounds 5a and 5b reduces the flexibility at the end of the ligand and, therefore, the ability to further accommodate the TBB cap to the surface of the protein outside the tunnel (ESI Fig. S2†).
Given their interesting IC50, the complexes with compounds 5c and 5e were subsequently used for MD simulations to further study their binding-stability. In both complexes (Fig. 7) the two methylene groups attached to the hydroxamate and the triazole interact with the side chains of Phe150 and Phe205 that make up the walls of the tunnel, while the second two-carbon linker and the TBB moiety protrude out of the binding site and interact with the surface of the protein. During the 10 ns simulation the binding modes of both compounds 5c and 5e remained stable and the interactions with the residues in the active site where maintained, while the triazole accommodated itself into the tunnel optimizing the van der Waals contacts with the side chains of Phe150 and Phe205. The different attachment (N1 or N2) of the linker to the TBB moiety does not change much the binding pose outside the catalytic cleft: compound 5c sits mainly on the side chain of Leu271, whereas compound 5e sways a little more so it interacts, not only with the side chain of Leu271, but also with Pro29 and with Phe150 in an edge to face interaction with the latter.
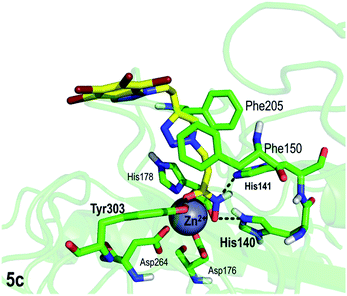 |
| Fig. 7 PyMOL stick and semi-transparent cartoon representation of the bound conformation of compounds 5c and 5e to HDAC1 after the 10 ns MD simulation. The main amino acids involved in the binding are represented as sticks and the catalytic Zn2+ is represented as a grey sphere. Hydrogen bonding and coordinating interactions are shown as dashed lines and the residues involved are labelled in bold. For the sake of clarity only polar hydrogens are shown. | |
CK2 binding. The catalytic subunit of CK2 has the common characteristic structure of all kinases, which is two distinct domains with the ATP binding site between them. This binding site is mainly a hydrophobic flat pocket made up of the side chains of Val53 and Ile66 at the ceiling, and Met163 and Ile174 at the bottom. The natural substrate ATP binds by inserting the adenine into the binding site, thus establishing van der Waals interactions with the amino acids at the bottom and ceiling of the pocket. The position is further stabilized by hydrogen bonding interactions between N1 and N7 of the base and the backbone NH of Val116 and carbonyl of Glu114, respectively; whilst the triphosphate moiety and the two Mg2+ ions interact with Asp156, Lys158, Asn161 and Asp175 (ESI Fig. S3†).66 Albeit having only halogen bond acceptors, TBB is a selective competitive inhibitor of the ATP-binding to CK2. It occupies the ATP-binding pocket establishing strong van der Waals interactions with the side chains of Val53, Ile66, Met163 and Ile174, which due to the shape and size of the pocket they make up, they are responsible for the marked selectivity of TBB for CK2.67 Moreover, a hydrogen bond between N2 and Lys68 is established, as TBB is deprotonated and negatively charged at physiological pH, as it can be seen in the PDB structure 1J91 (ESI Fig. S4†).67 In the case of the TBB analogue tetrabromobenzimidazole, the lack of the N2 changes the overall charge of the ligand and thus the ionic interaction with Lys68 is not established. This change modifies the orientation of the binding that promotes the formation of a halogen bond between one of the Br atoms and the backbone carbonyl of Val116 (ESI Fig. S5†).68 These modifications not only do not impair the binding to CK2, but also make it possible to introduce different substitutions at position 2.The docking of the majority of the designed inhibitors lodged the TBB moiety in a similar way as in the crystallized complex, burying it deep inside the hydrophobic pocket of CK2 in such an orientation that, in some cases, a halogen bond could be established with the carbonyl oxygen of Val116, similar to the one established by tetrabromobenzoimidazole in PDB code 2OXY. Moreover, in all of these dockings strong van der Waals contacts are established with the side chains of Val45, Val53, Ile66, Val95, Phe113, Val116, Met163 and Ile174, analogue to the ones in the crystallized complexes. Depending on the length of the ligands and the position of the substitution on the TBB moiety they adopt different orientations within the binding site and display different orientations of their substituents. Compounds 5a–5f orient the hydroxamic acid moiety towards the side chains of Asp156, Lys158, Asn161 and Asp175 that make up one of the Mg2+-binging sites in CK2. The presence of the additional aromatic ring of compounds 5g–h does not give good binding poses as they are not able to fit within the binding site due to the increase in length derived from the extra aromatic ring and the linker (ESI Fig. S6†). They are not able to interact with the side chains of Asp156, Lys158, Asn161 and Asp175, and due to this increased length these compounds interact with Val116 through the hydroxamic acid in a bidentate interaction while the TBB moiety sits on top of the side chain of Lys158, probably stabilized by a π-cation interaction. These results suggest that equilibrium between length and ligand flexibility has to be met in order to have an efficient binding of TBB derivatives to the ATP-binding site in CK2. The complexes of CK2 with compound 5c and its direct analogue 5e, that presented the highest inhibitory activity, were subjected to MD simulations to further study their binding stability and try to understand the slight differences brought about by distinct TBB substitution.
The initial good van der Waals interactions between the TBB moiety of both compounds and the side chains of Val45, Val53, Ile66, Phe113, Met163 and Ile174 (as can be seen in the corresponding images of ESI Fig. S6†) were maintained during the MD simulation, in addition to the interaction of the triazole moiety with the protonated side chain of His160. Both complexes remained quite stable due to the interaction of the hydroxamic acid moiety with the side chains of Asp156, Lys158 and Asp175 and the establishment of a halogen bond between the carbonyl oxygen of the backbone of Val116 and the Br at position 4 and 5 of the TBB moieties of compounds 5e and 5c, respectively (Fig. 8).
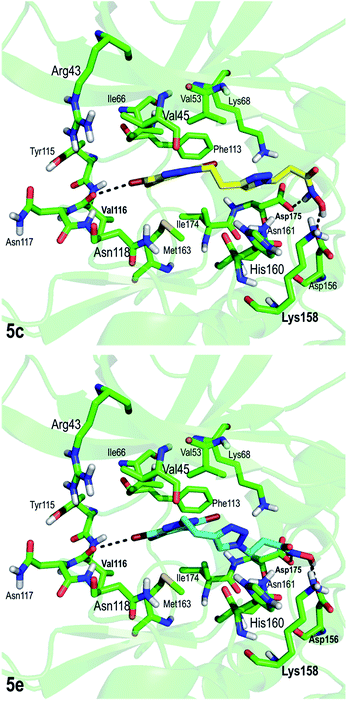 |
| Fig. 8 PyMOL stick and semi-transparent cartoon representation of the bound conformation of compounds 5c and 5e to CK2 after the 10 ns MD simulation. The main amino acids involved in the binding are represented as sticks. For the sake of clarity only polar hydrogens are shown. | |
Experimental
Materials and methods
Melting points (uncorrected) were taken in open-end capillary tubes and were determined on a Stuart Scientific SMP3 apparatus. Thin-layer chromatography (TLC) was run on Merck silica gel 60 F254 plates. Flash column chromatography was performed using silica gel Merk-60 (230–400 mesh). Unless stated otherwise, purchased starting materials used were high-grade commercial products. NMR spectra were recorded at 400 MHz (1H) and 101 MHz (13C) on a Bruker 400-AC magnetic resonance spectrometer at room temperature in CDCl3 [calibrated at 7.26 ppm (1H) and 77.0 ppm (13C)] and in DMSO-d6 [calibrated at 2.50 ppm (1H) and 39.5 ppm (13C)]. Data are presented as follows: chemical shift (ppm), multiplicity (s singlet, bs broad singlet, d doublet, t triplet, q quartet, m multiplet), coupling constant J (Hz) and integration. Data for 13C NMR are reported in terms of chemical shifts (ppm). HPLC-MS was performed at Synthelia Organics SL., using an Agilent 1100 HPLC system and Xterra C18-5 μm or ACE C18-3 μm reverse phase columns. The following conditions were used: method A, Xterra C18-5 μm column, a flow rate of 2 mL min−1, eluting with solvent A (20 mM NH4HCO3/water) and solvent B (ACN) at an isocratic run of 40% of solvent A and 60% of solvent B over 15 min with detection at 254 nm; method B, a flow rate of 0.75 mL min−1, eluting with solvent A (20 mM NH4HCO3/water) and solvent B (ACN) at a gradient run from 20–95% of solvent B over 6 min with detection at 254 nm. For reporting HPLC-MS data, m/z values and retention time for each method are given. Elemental analyses (C, H, N, S) were performed on a LECO CHNS-932 apparatus at the Microanalyses Service of the Universidad Complutense de Madrid.
4,5,6,7-Tetrabromo-1H-benzotriazole (TBB),69 N-alkyl derivatives 1 and 2a–b58 and azides 3a–e70,71 were synthesized according to literature procedures. Melting points, 1H and/or 13C NMR data were in agreement with those published.
4,5,6,7-Tetrabromo-1-(prop-2-yn-1-yl)-1H-benzo[d][1,2,3]triazole (1). To a suspension of TBB (500 mg, 1.1 mmol) and K2CO3 (1.4 g, 10.15 mmol) in acetone (20 mL) was added 3-bromo-1-propyne (0.3 mL, 3.4 mmol). This reaction was carried out by microwave assistance at 150 °C for 1 min. After cooling to room temperature, the solvent was removed under vacuum and the residue was dissolved in 20 mL of AcOEt and washed with brine (2 × 20 mL). The organic layer was dried (MgSO4), filtered, evaporated to dryness and purified by flash chromatography (Hex/DCM 4
:
1) to afford 1 as a white solid (382 mg, 72%), 1H NMR (400 MHz, DMSO) δ 5.81 (d, J = 2.3 Hz, 2H), 3.68 (t, J = 2.3 Hz, 1H). 13C NMR (101 MHz, DMSO) δ 144.8, 131.4, 129.1, 124.1, 115.8, 106.6, 78.3, 77.4, 40.2.
4,5,6,7-Tetrabromo-1-(but-3-yn-1-yl)-1H-benzo[d][1,2,3]triazole (2a) and 4,5,6,7-tetrabromo-2-(but-3-yn-1-yl)-2H-benzo[d][1,2,3]triazole (2b). To a suspension of TBB (200 mg, 0.46 mmol) and K2CO3 (565 mg, 4.1 mmol) in acetonitrile (10 mL) was added 4-bromo-1-butyne (0.12 mL, 1.38 mmol) and the mixture was stirred at RT under argon for 24 hours. After cooling to room temperature, the solvent was removed under vacuum and the residue was dissolved in 20 mL of AcOEt and washed with brine (2 × 20 ml). The organic layer was dried (MgSO4), filtered, evaporated to dryness and purified by flash chromatography (Hex/DCM 4
:
1) to afford 2a (47 mg, 21%) and 2b (114 mg, 51%). 2a: 1H NMR (400 MHz, DMSO) δ 5.07 (t, J = 6.8 Hz, 2H), 2.96–2.86 (m, 3H). 13C NMR (101 MHz, DMSO) δ 144.8, 131.8, 128.7, 123.6, 115.8, 106.9, 79.7, 74.0, 48.1, 20.5. 2b: 1H NMR (400 MHz, DMSO) δ 4.94 (t, J = 6.7 Hz, 2H), 3.03 (td, J = 6.7, 2.6 Hz, 2H), 2.87 (t, J = 2.6 Hz, 1H). 13C NMR (101 MHz, DMSO) δ 142.5, 125.8, 113.6, 79.8, 73.6, 55.5, 19.1.
General procedure for the synthesis of azides 3a–d
To a solution of the corresponding alkyl bromide (1.0 mmol) in DMF (5 mL) under argon was added NaN3 (65 mg, 1.0 mmol) and the mixture was stirred at 60 °C overnight. After cooling to room temperature, AcOEt (20 mL) and aqueous HCl 0.1 M (20 mL) were successively added, and the water phase was extracted with AcOEt (2 × 20 mL). The combined organic extracts were washed with brine, dried over MgSO4, filtered and concentrated under vacuum to afford the desired azides, which were used in the next step without further purification.
3-Azidopropanoic acid (3a). Following the general procedure, the reaction of 3-bromopropionic acid (1.0 g, 6.5 mmol) with NaN3 (423 mg, 6.5 mmol) gave 3a as a white solid (501 mg, 67%). 1H NMR (400 MHz, CDCl3) δ 3.58 (t, J = 6.4 Hz, 2H), 2.62 (t, J = 6.4 Hz, 2H).
4-Azidobutanoic acid (3b). The reaction of 4-bromobutanoic acid (835 mg, 5 mmol) with NaN3 (325 mg, 5 mmol) gave 3b as a white solid (561 mg, 87%). 1H NMR (400 MHz, CDCl3) δ 3.38 (t, J = 7.2 Hz, 2H), 2.26 (t, J = 7.2 Hz, 2H), 1.97–1.88 (m, 2H).
5-Azidopentanoic acid (3c). The reaction of 5-bromopentanoic acid (1.0 g, 5.5 mmol) with NaN3 (358 mg, 5.5 mmol) gave 3c as a white solid (464 mg, 59%). 1H NMR (CDCl3, 400 MHz) δ 3.26–3.33 (m, 2H), 2.36–2.42 (m, 2H), 1.60–1.75 (m, 4H).
4-(Azidomethyl)benzoic acid (3d). The reaction of 4-(bromomethyl)benzoic acid (1.0 g, 4.7 mmol) with NaN3 (306 mg, 4.7 mmol) gave 3d as a white solid (752 mg, 91%). 1H NMR (400 MHz, CDCl3) δ 8.12 (s, 1H), 7.50–7.38 (m, 4H), 2.48 (s, 2H).
4-Azidobenzoic acid (3e). To a solution of methyl 4-aminobenzoate (1.0 g, 6.6 mmol) in anhydrous CH3CN (60 mL) under argon were successively added t-BuONO (1.3 mL, 9.9 mmol) and TMSN3 (1.0 mL, 7.9 mmol) dropwise at 0 °C. The reaction mixture was stirred at the same temperature for 30 min and at room temperature for 3 h. The solvent was removed under vacuum and the residue was purified by flash chromatography (Hexane/AcOEt 95
:
5) to afford 640 mg of a white solid, which was subsequently dissolved in methanol (3 mL). To this solution, aqueous NaOH 1 M (3 mL) was added and the mixture was stirred for 2 h at room temperature. After addition of AcOEt (10 mL) the solution was neutralized with aqueous HCl 1 M (pH ≈ 1). The two phases were separated and the water phase was extracted with AcOEt (2 × 10 mL). The combined organic extracts were washed with brine, dried over MgSO4 and concentrated under vacuum to give 3e as a white solid (570 mg, 53% over two steps). 1H NMR (400 MHz, CDCl3) δ 8.10 (d, J = 8.5 Hz, 2H), 7.11 (d, J = 8.5 Hz, 2H).
General procedure for the synthesis of hydroxamate derivatives 4a–e
To a solution of the corresponding acid (3a–e) (2.0 mmol) in DMF (10 mL) were successively added HOBt (1.2 equiv, 324 mg, 2.4 mmol), NMM (3 equiv, 0.66 mL, 6.0 mmol), NH2OTHP (2 equiv, 468 mg, 4.0 mmol) and EDCI (1.4 equiv, 538 mg, 2.8 mmol) and the mixture was stirred at room temperature overnight. The solution was then diluted with AcOEt (40 mL) and subsequently washed with saturated aqueous NH4Cl (40 mL) and brine, dried over MgSO4. The drying agent was filtered off, the solvent was removed in vacuo and the residue was purified by flash chromatography on silica gel (the eluent is indicated in each case) to afford the desired hydroxamic acid derivatives 4a–e.
3-Azido-N-((tetrahydro-2H-pyran-2-yl)oxy)propanamide (4a). Following the general procedure, the reaction of 3-azidopropanoic acid 3a (230 mg, 2.0 mmol) gave, after flash chromatography (DCM/MeOH 95
:
5), 4a as a white solid (205 mg, 48%). 1H NMR (400 MHz, CDCl3) δ 8.42 (s, 1H), 4.93 (t, J = 6.8 Hz, 1H), 3.68–3.52 (m, 2H), 2.33 (t, J = 6.9 Hz, 2H), 1.77 (t, J = 6.8 Hz, 2H), 1.69–1.47 (m, 6H).
4-Azido-N-((tetrahydro-2H-pyran-2-yl)oxy)butanamide (4b). Following the general procedure, the reaction of 4-azidobutanoic acid 3b (258 mg, 2.0 mmol) gave, after flash chromatography (hexane/AcOEt 3
:
2), 4b as a white solid (333 mg, 73%). 1H NMR (400 MHz, CDCl3) δ 8.36 (s, 1H), 4.96 (t, J = 7.0 Hz, 1H), 3.69 (t, J = 7.1 Hz, 2H), 2.31–2.24 (m, 2H), 1.99–1.52 (m, 10H).
5-Azido-N-((tetrahydro-2H-pyran-2-yl)oxy)pentanamide (4c). Following the general procedure, the reaction of 5-azidopentanoic acid 3c (286 mg, 2.0 mmol) gave, after flash chromatography (hexane/AcOEt 3
:
2), 4c as a white solid (295 mg, 61%). 1H NMR (400 MHz, CDCl3) δ 8.41 (s, 1H), 4.93 (t, J = 6.8 Hz, 1H), 3.66–3.58 (m, 2H), 2.39 (t, J = 6.9 Hz, 2H), 1.97–1.55 (m, 12H).
4-(Azidomethyl)-N-((tetrahydro-2H-pyran-2-yl)oxy)benzamide (4d). Following the general procedure, the reaction of 4-(azidomethyl)benzoic acid 3d (354 mg, 2.0 mmol) gave, after flash chromatography (hexane/AcOEt 7
:
3), 4d as a white solid (309 mg, 56%). 1H NMR (400 MHz, CDCl3) δ 8.98 (s, 1H), 7.77 (d, J = 8.1 Hz, 2H), 7.38 (d, J = 8.1 Hz, 2H), 5.07 (t, J = 6.8 Hz, 1H), 4.39 (s, 2H), 4.05–3.93 (m, 1H), 3.72–3.52 (m, 1H), 1.85–1.52 (m, 6H).
4-Azido-N-((tetrahydro-2H-pyran-2-yl)oxy)benzamide (4e). Following the general procedure, the reaction of 4-azidobenzoic acid 3e (326 mg, 2.0 mmol) gave, after flash chromatography (DCM/MeOH 9
:
1), 4e as a white solid (309 mg, 59%). 1H NMR (400 MHz, DMSO) δ 9.02 (s, 1H), 7.82 (d, J = 7.6 Hz, 2H), 7.40 (d, J = 7.6 Hz, 2H), 4.98 (t, J = 7.2 Hz, 1H), 3.52 (t, J = 7.1 Hz, 2H), 1.86–1.48 (m, J = 7.1 Hz, 6H).
General procedure for the click reaction: synthesis of triazole-based dual inhibitors 5a–h
To a mixture of the azide (0.1 mmol) and the corresponding alkyne (1.1 equiv, 0.11 mmol) in DMF (1 mL) under argon, were successively added sodium ascorbate (30 mol%, 0.03 mmol, 30 μL of freshly prepared 1 M solution in water) and copper(II) sulfate pentahydrate (10 mol%, 0.01 mmol, 33 μL of freshly prepared 0.3 M solution in water). The heterogeneous mixture was stirred vigorously overnight at room temperature. After removing the solvent under vacuum, AcOEt (10 mL) was added and the resulting solution was washed with NH4Cl (10 mL) and brine and dried over (MgSO4). Filtration and evaporation of the solvent gave a residue which was purified by flash chromatography (the eluent is indicated in each case). Final removal of the THP protecting group was carried out as follows: to a solution of the protected hydroxamic acid in MeOH (4 mL) was added 4 M HCl in dioxane (0.4 mL, 0.4 mmol) and the mixture was stirred at room temperature for 2 h. Solvent co-evaporation with MeOH (×3) afforded the corresponding adducts 5a–h.
N-Hydroxy-3-(4-((perbromo-1H-benzo[d][1,2,3]triazol-1-yl)methyl)-1H-1,2,3-triazol-1-yl) propanamide (5a). Following the general procedure, the reaction of 4a (30 mg, 0.14 mmol) with 1 (71 mg, 0.15 mmol) afforded, after flash chromatography (Hex/AcOEt 1
:
3) and further hydroxamate deprotection, 5a as a white solid (59 mg, 71%), mp 130–132 °C. 1H NMR (400 MHz, DMSO) δ 10.12 (br s, 1H), 9.93 (br s, 1H), 8.09 (s, 1H), 6.25 (s, 2H), 4.54 (t, J = 6.7 Hz, 2H), 2.94 (t, J = 6.7 Hz, 2H). 13C NMR (101 MHz, CDCl3) δ 170.8, 145.6, 131.6, 129.80, 124.7, 123.9, 116.8, 114.1, 106.0, 52.3, 46.1, 34.4. HPLC-MS: m/z 603 [M − 2H]+, tR: 11.12 min, method A. Anal. calcd for C12H11Br4N7O2 requires C, 23.83; H, 1.83; Br, 52.84; N, 16.21; O, 5.29; found: C, 23.72; H, 1.92; N, 16.31.
N-Hydroxy-5-(4-((perbromo-1H-benzo[d][1,2,3]triazol-1-yl)methyl)-1H-1,2,3-triazol-1-yl)pentanamide (5b). The reaction of 4c (30 mg, 0.12 mmol) with 1 (64 mg, 0.14 mmol) afforded, after flash chromatography (Hex/AcOEt 1
:
5) and further hydroxamate deprotection, 5b as a white solid (48 mg, 64%), mp 167–169 °C. 1H NMR (400 MHz, DMSO) δ 10.04 (br s, 1H), 9.85 (br s, 1H), 8.09 (s, 1H), 6.26 (s, 2H), 4.31 (t, J = 7.0 Hz, 2H), 2.30 (t, J = 7.4 Hz, 2H), 1.84–1.68 (m, 2H), 1.51–1.37 (m, 2H). 13C NMR (101 MHz, DMSO) δ 172.9, 144.9, 142.0, 131.8, 128.8, 123.7, 123.3, 115.7, 106.8, 51.2, 45.6, 32.4, 28.9, 21.2. HPLC-MS: m/z 631 [M]+, tR: 11.67 min, method A. Anal. calcd for C14H13Br4N7O2 requires C, 26.65; H, 2.08; Br, 50.66; N, 15.54; O, 5.07; found: C, 26.49; H, 2.07; N, 15.63.
N-Hydroxy-3-(4-(2-(perbromo-1H-benzo[d][1,2,3]triazol-1-yl)ethyl)-1H-1,2,3-triazol-1-yl)propanamide (5c). The reaction of 4a (30 mg, 0.14 mmol) with 2a (75 mg, 0.15 mmol) afforded, after flash chromatography (Hex/AcOEt 1
:
3) and further hydroxamate deprotection, 5c as a white solid (63 mg, 73%), mp 130–132 °C. 1H NMR (400 MHz, DMSO) δ 10.50 (br s, 1H), 8.83 (br s, 1H), 7.88 (s, 1H), 5.17 (t, J = 7.2 Hz, 2H), 4.51 (t, J = 5.3 Hz, 2H), 3.30 (t, J = 7.2 Hz, 2H), 2.56 (t, J = 5.2 Hz, 2H). 13C NMR (101 MHz, DMSO) δ 165.8, 144.9, 142.0, 131.8, 128.5, 123.5, 123.2, 115.7, 106.8, 49.4, 45.5, 32.7, 27.0. HPLC-MS: m/z 618 [M + H]+, tR: 3.01 min, method B. Anal. calcd for C13H11Br4N7O2 requires C, 25.31; H, 1.80; Br, 51.81; N, 15.89; O, 5.19; found: C, 25.43; H, 1.75; N, 15.96.
N-Hydroxy-5-(4-(2-(perbromo-1H-benzo[d][1,2,3]triazol-1-yl)ethyl)-1H-1,2,3-triazol-1-yl)pentanamide (5d). The reaction of 4c (25 mg, 0.1 mmol) with 2a (56 mg, 0.12 mmol) afforded, after flash chromatography (Hex/AcOEt 1
:
6) and further hydroxamate deprotection, 5d as a white solid (45 mg, 66%), mp 141–142 °C. 1H NMR (300 MHz, DMSO) δ 10.19 (br s, 1H), 7.85 (s, 1H), 5.18 (t, J = 7.1 Hz, 2H), 4.28 (t, J = 6.9 Hz, 2H), 3.32 (t, J = 7.1 Hz, 2H), 2.32 (t, J = 7.14 Hz, 2H), 1.77–1.69 (m, 2H), 1.46–1.38 (m, 2H). 13C NMR (101 MHz, DMSO) δ 173.0, 144.8, 142.1, 131.8, 128.5, 123.5, 122.9, 115.7, 106.7, 51.2, 49.5, 32.5, 29.0, 27.0. HPLC-MS: m/z 645 [M]+, tR: 3.93 min, method B. Anal. calcd for C15H15Br4N7O2 requires C, 27.93; H, 2.34; Br, 49.56; N, 15.20; O, 4.96; found: C, 28.50; H, 2.25; N, 14.85.
N-Hydroxy-3-(4-(2-(perbromo-2H-benzo[d][1,2,3]triazol-2-yl)ethyl)-1H-1,2,3-triazol-1-yl)propanamide (5e). The reaction of 4a (30 mg, 0.14 mmol) with 2b (75 mg, 0.15 mmol) afforded, after flash chromatography (Hex/AcOEt 1
:
3) and further hydroxamate deprotection, 5e as a white solid (63 mg, 73%), mp 173–175 °C. 1H NMR (400 MHz, DMSO) δ 10.48 (br s, 1H), 8.82 (br s, 1H), 7.80 (s, 1H), 5.05 (t, J = 7.2 Hz, 2H), 4.49 (t, J = 6.7 Hz, 2H), 3.44 (t, J = 6.7 Hz, 2H), 2.55 (t, J = 7.2 Hz, 2H). 13C NMR (101 MHz, DMSO) δ 165.8, 142.5, 142.2, 125.6, 123.1, 113.6, 56.4, 45.5, 32.7, 25.6. HPLC-MS: m/z 618 [M − H]+, tR: 3.34 min, method B. Anal. calcd for C13H11Br4N7O2 requires C, 25.31; H, 1.80; Br, 51.81; N, 15.89; O, 5.19; found: C, 25.14; H, 1.75; N, 16.01.
N-Hydroxy-4-(4-(2-(perbromo-2H-benzo[d][1,2,3]triazol-2-yl)ethyl)-1H-1,2,3-triazol-1-yl)butanamide (5f). The reaction of 4b (40 mg, 0.18 mmol) with 2b (93 mg, 0.19 mmol) afforded, after flash chromatography (Hex/AcOEt 1
:
5) and further hydroxamate deprotection, 5f as a white solid (91 mg, 83%), mp 175–177 °C. 1H NMR (400 MHz, DMSO) δ 10.40 (br s, 1H), 7.86 (s, 1H), 5.07 (t, J = 7.2 Hz, 2H), 4.28 (t, J = 6.6 Hz, 2H), 3.44 (t, J = 7.1 Hz, 2H), 2.07–1.78 (m, 4H). 13C NMR (101 MHz, DMSO) δ 168.0, 142.5, 142.3, 125.5, 122.9, 113.6, 56.4, 48.8, 28.9, 25.91, 25.7. HPLC-MS: m/z 632 [M + H]+, tR: 9.92 min, method A. Anal. calcd for C14H13Br4N7O2 requires C, 26.65; H, 2.08; Br, 50.66; N, 15.54; O,5.07; found: C, 26.84; H, 1.75; N, 15.48.
N-Hydroxy-4-((4-(2-(perbromo-1H-benzo[d][1,2,3]triazol-1-yl)ethyl)-1H-1,2,3-triazol-1-yl)methyl)benzamide (5g). The reaction of 4d (50 mg, 0.18 mmol) with 2a (96 mg, 0.20 mmol) afforded, after flash chromatography (DCM/MeOH 95
:
5) and further hydroxamate deprotection, 5g as a white solid (87 mg, 71%), mp 139–141 °C. 1H NMR (400 MHz, DMSO) δ 11.24 (br s, 1H), 7.98 (s, 1H), 7.74 (d, J = 8.2 Hz, 2H), 7.26 (d, J = 8.2 Hz, 2H), 5.59 (s, 2H), 5.18 (t, J = 7.1 Hz, 2H), 3.34 (t, J = 7.0 Hz, 2H). 13C NMR (101 MHz, DMSO) δ 164.2, 145.4, 143.1, 139.6, 133.0, 132.3, 129.1, 128.1, 127.8, 124.1, 116.3, 107.3, 52.7, 50.0, 27.5. HPLC-MS: m/z 680 [M + H]+, tR: 3.22 min, method A. Anal. calcd for C18H13Br4N7O2 requires C, 31.84; H, 1.93; Br, 47.07; N, 14.44; O, 4.71; found: C, 31.71; H, 1.73; N, 14.63.
N-Hydroxy-4-((4-(2-(perbromo-2H-benzo[d][1,2,3]triazol-2-yl)ethyl)-1H-1,2,3-triazol-1-yl) methyl)benzamide (5h). The reaction of 4d (50 mg, 0.18 mmol) with 2b (96 mg, 0.20 mmol) afforded, after flash chromatography (Hex/AcOEt 1
:
5) and further hydroxamate deprotection, 5h as a white solid (79 mg, 65%), mp 166–168 °C. 1H NMR (400 MHz, DMSO) δ 11.21 (br s, 1H), 7.89 (s, 1H), 7.71 (d, J = 8.3 Hz, 2H), 7.26 (t, J = 8.3 Hz, 2H), 5.57 (s, 2H), 5.07 (t, J = 7.0 Hz, 2H), 3.45 (t, J = 7.0 Hz, 2H). 13C NMR (101 MHz, DMSO) δ 163.6, 142.7, 142.5, 139.0, 132.4, 127.6, 127.3, 125.6, 123.3, 113.6, 56.4, 52.2, 25.7. HPLC-MS tR: 10.33 min, non-ionizable, method A. Anal. calcd for C18H13Br4N7O2 requires C, 31.84; H, 1.93; Br, 47.07; N, 14.44; O, 4.71; found: C, 31.40; H, 1.98; N, 14.35.
Molecular modeling
On one hand, since no molecular model was available for the structure of HDAC1 at the beginning of the project, an homology model was built using SWISS MODEL web server72–74 and was assessed by superimposition to other type I HDAC crystallized structures. The H++ web server75 which relies on the AMBER76 force field parameters and finite difference solutions to the Poisson–Boltzmann equation was used to calculate the protonation state of tritable groups such as the imidazole side chains of the histidine residues located in the catalytic site: His140 doubly protonated, His141 protonated in Nδ and His178 protonated in Nε. However, after all the computational work in this project was finished, a crystal structure of the complex of acetic acid-bound HDAC1 with the regulatory protein MTA1 was published under the PDB code 4BKX.77 The amino acid sequence of the crystallized HDAC1 started at Arg8 and ended at Ala376, whereas the modelled one started at Arg8 and ended at Leu373, only three amino acids less than the crystal structure, which did not alter the overall structure of the modelled protein. Both structures were superimposed using PyMOL to assess any possible differences that would make imperative to repeat the modelling work. The overall alignment of the α-carbons of the matching amino acids presented an RMS of 0.386 Å and visual inspection of the overall structure and the binding site showed no remarkable differences that would compromise the modelled results (ESI Fig. S7†). Following these inspections we resolved not to repeat the modelling work as the results would not be substantially different. The numbering of the amino acids of the model is equivalent to the one of the crystal structure in 4BKX.
On the other hand, the structure of CK2 bound to a non-hydrolysable ATP analogue in complex with a Mg2+ ion (PDB code 1DAW) was used to build the kinase–bound complexes. The H++ web server predicted the side chain of His160, located at the entrance on the binding site to be doubly protonated. The missing residues and side chains were added and the macromolecule geometries refined by using Protein Preparation module in Maestro. The ligands (compounds 5c, 5e) were built using Maestro LigPrep module (http://www.Schrodinger.com). The pKa of the tritable groups were calculated using the Sparc pKa online calculator78 and the non-protonated form of the hydroxamate was used for the docking calculations. The Glide module79–81 was used to perform the docking calculations. For the HDAC1 complexes the centre of the box was positioned on the catalytic zinc ion present in the active site, while for CK2 it was located at the centre of the non-hydrolysable ATP analogue of the crystal structure. The box size was set up to enclose the ligand-binding domain to ensure a proper exploration of the binding poses. The docking procedure was performed with XP (extra precision) mode, and a van der Waals radii scale factor of 1.0/0.8 for receptor and ligand, respectively. The best-obtained result for each ligand was considered for analysis of the ligand–receptor interactions and subsequent molecular modelling simulations.
For the MD simulations the charge distribution for the ligands studied was obtained by fitting the quantum mechanically calculated (RHF/6-31G**//RHF/3-21G*) molecular electrostatic potential (MEP) of the geometry-optimized molecules to a point charge model, as implemented in Gaussian 03 (Gaussian, Inc., Wallingford, CT). The general AMBER (http://ambermd.org/) force field (GAFF) was used to assign bonded and nonbonded parameters (parm03) to the ligand atoms. Each HDAC1 molecular system was immersed in a truncated octahedron containing ∼10
000 TIP3P water molecules82 and 4 Cl− ions,83 while each CK2 system was immersed in a truncated octahedron containing ∼13
000 TIP3P water molecules and 9 Cl− ions to achieve system electroneutrality. The sander and pmemd modules of the AMBER12 suite (http://ambermd.org/) were used for the restrained and unrestrained MD simulations, respectively. Periodic boundary conditions were applied and electrostatic interactions were treated using the smooth particle mesh Ewald method84 with a grid spacing of 1 Å. The cutoff distance for the non-bonded interactions was 9 Å, the SHAKE85 algorithm was applied to all bonds, and an integration step of 2.0 fs was used throughout. After an initial energy minimization of the water molecules and counter-ions, the system was heated to 300 K in 25 ps after which the solvent was allowed to redistribute around the positionally restrained solute for 220 ps. After this time, they were allowed to move freely so as to explore the mutual adaptation between ligand and the protein. Snapshots from each 10 ns MD trajectory were collected every 20 ps for further analysis carried out with the ptraj module of AMBER to monitor the stability of the complexes.
Determination of CK2α inhibition
The reaction mixture (20 μL) for determination of human CK2α (prepared as described elsewhere)86 activity contained: peptide substrate (100 μM, RRRADDSDDDDD from Aldrich), Tris–HCl pH 7.5 (20 mM), MgCl2 (15 mM), 2-mercaptoethanol (6 mM) and γ[32P] ATP (100 μM) and the compound (50 μM) in 5% DMSO. After 30 min of incubation at 37 °C, 10 μL of every assay mixture was spotted onto a square (1 cm × 1 cm) of Whatman P81 paper and dried for 15 min. Next, squares were immersed in cold 0.5% phosphoric acid, and washed 3 times, for 15 min each. Then the squares were washed with 96% EtOH and dried for 30 min at 40 °C. The radioactivity was quantified by BECKMAN LS6500 scintillation counter. The activity was calculated as the percentage of incorporated [32P]. The concentration of compound that provided 50% inhibition of enzymatic activity (IC50) was determined by semi-logarithmic dose–response plots (Graph Pad Prism 5.0 for Windows, Graph Pad Software Inc., San Diego, California, USA, 2007).
HDAC1 inhibition assay
In vitro HDAC1 inhibition was performed using HDAC1 Fluorimetric Drug Discovery Assay Kit from Enzo Life Sciences, Inc. The reaction was prepared in HDAC assay buffer (50 mM Tris–HCl, pH = 8.0, 137 mM NaCl, 2.7 mM KCl, 1 mM MgCl2, 1 mg mL−1 BSA). HDAC1 enzyme (0.1 μg per well) was incubated at 37 °C with 5 μM fluorogenic substrate at indicated concentrations of inhibitor diluted in 5% DMSO. Reactions were stopped after 60 min with the developer, and the plate was incubated at RT for 45 min, followed by measuring the fluorescence (Ex. 360 nm, Em. 460 nm, Synergy Mx, BioTek).
In vitro studies on mammalian cell lines
Human T-leukemia cells of Jurkat line and human breast adenocarcinoma cells of MCF-7 line were obtained from cell culture collection of Institute of Cancer Research, Vienna Medical University (Vienna, Austria). Human keratinocytes of HaCat line were obtained from Ludwig Institute for Cancer Research (Uppsala, Sweden). Murine leukemia cells of L1210 line and human embryonic kidney cells of 293T line were obtained from cell culture collection of R.E. Kavetsky Institute of Experimental Pathology, Oncology and Radiobiology, National Academy of Sciences of Ukraine (Kyiv, Ukraine). Cells were cultured in the RPMI medium supplemented with 10% fetal calf serum (Sigma Chemical Co., St. Louis, USA), 50 μg mL−1 streptomycin (Sigma Chemical Co., St. Louis, USA), 50 units per mL penicillin (Sigma Chemical Co., St. Louis, USA) in 5% CO2-containing humidified atmosphere at 37 °C.
For experiments, cells were seeded into 24-well tissue culture plates (Greiner Bio-one, Germany). Stock solutions (2 mM) of each CK2 inhibitor in DMSO (99.5% pure, Sigma, USA) were prepared, and additionally dissolved in serum-free culture medium (RPMI for leukemia cells and DMEM for carcinoma cells) prior to addition to cell culture. Final concentration of DMSO in cell culture was 0.5% or less. Cytotoxicity studies on Jurkat and MCF-7 cells revealed no statistically significant toxicity of 0.5% DMSO solution on these cell lines.
Cytotoxic effect of antitumor drugs was studied under the light microscope (Evolution 300, Delta Optical, Poland) after cell staining with trypan blue dye (0.1%).
FITC-conjugated Annexin V (BD Pharmingen, USA) and propidum iodide (Sigma, USA) double staining were performed to detect early apoptotic events under treatment of Jurkat cels by CK2-HDAC inhibitors. In 24 h after the addition of 5c and 5e Jurkat cells were centrifuged at 2000 rpm, washed twice with 1× PBS, and incubated for 15 min in Annexin V binding buffer (BD Pharmingen, USA) containing 1/20 volume of FITC-conjugated Annexin V solution and PI (20 PI 20 of FITC-conjugated training 1x PBS, and incubated for 15 min in Annexin V. Cytomorphological investigations were performed on Zeiss AxioImager A1 fluorescent microscope.
DAPI staining was performed for studying chromatin condensation in MCF-7 cells after treatment with 5c and 5e. 24 h after the addition of CK2 inhibitors, MCF-7 cells were washed twice with 1× PBS, fixed in 4% solution of paraformaldehyde for 15 min at room temperature, and then permeabilized by 0.1% Triton X-100 solution in PBS for 3 min. Then, cells were incubated with 1 bated with 1 solution of pa,6-diamidino-2-phenylindole) (Sigma, USA) for 5 min, washed twice with PBS and cover glasses with fixed cells were placed on slides. Cytomorphological investigations were performed on Zeiss AxioImager A1 fluorescent microscope (Zeiss, Germany).
All experiments were performed in triplicate and repeated 3 times. For statistical analysis of the obtained results, standard variation data within a group was calculated together with a statistical reliability of differences between two groups of data assessed by Student alt test. The level of significance was set to 0.05.
Conclusions
We have synthesized a series of molecules that combine HDAC1 and CK2 inhibiting moieties (a hydroxamate ZBG and a TBB core, respectively) using a “click” chemistry approach. We have found dual hit compounds demonstrating excellent binding features at both target proteins. Docking and MD experiments allowed us to analyze the best linkers to connect the hydroxamate ZBG and the TBB moiety, maintaining a binding mode that resembles the one found in the previously reported crystal structures for both enzymes (ESI Fig. S1, S4 and S5†). As expected, the TBB moiety characteristic of CK2 reported inhibitors can act as a good cap group upon binging to HDAC 1. On the other hand, the widely used hydroxamate ZBG for HDAC 1 has demonstrated versatility to establish a highly effective hydrogen bonding network within the phosphate binding area in CK2. As CK2 is responsible for the regulation of HDACs by phosphorylation, we hypothesize that the combined inhibition of both enzymes in the same molecule could find clinical utility as antitumor agents. We have found compounds with similar affinity for the target proteins and cytotoxic activity in the low micromolar LC50 in two mammalian cancer cell lines. We consider that these new molecules are an interesting starting point for further development of antitumor drugs targeting two relevant enzymes involved in the same signaling pathway, as an attractive alternative to classical combination therapy.
Acknowledgements
This work was supported by the Spanish Ministry of Science and Innovation (CTQ2011-24741 and CTQ2014-52604 R). We thank Airbus Military for fellowships to M. P. Grants to J. H. T., C. C. and K. F. from Fundación Universitaria San Pablo CEU are also acknowledged. We thank also Synthelia Organics SL. for HPLC-MS experiments.
References
- C. L. Sawyers, Nature, 2007, 449, 993–996 CrossRef CAS PubMed.
- G. R. Zimmermann, J. Lehar and C. T. Keith, Drug Discovery Today, 2007, 12, 34–42 CrossRef CAS PubMed.
- E. L. Kwak, J. W. Clark and B. Chabner, Clin. Cancer Res., 2007, 13, 5232–5237 CrossRef CAS PubMed.
- S. Frantz, Nat. Rev. Drug Discovery, 2006, 5, 881–882 CrossRef PubMed.
- R. Morphy and Z. Rankovic, J. Med. Chem., 2005, 48, 6523–6543 CrossRef CAS PubMed.
- J. R. Morphy and C. J. Harris, Designing multi-target drugs, Royal Society of Chemistry, Cambridge, UK, 2012 Search PubMed.
- J. U. Peters, Polypharmacology in Drug Discovery, Wiley, Hoboken, New Jersey, Hindawi Publishing Corporation edn, 2011 Search PubMed.
- N. M. O'Boyle and M. J. Meegan, Curr. Med. Chem., 2011, 18, 4722–4737 CrossRef.
- M. J. Fray, G. Bish, A. D. Brown, P. V. Fish, A. Stobie, F. Wakenhut and G. A. Whitlock, Bioorg. Med. Chem. Lett., 2006, 16, 4345–4348 CrossRef CAS PubMed.
- J. L. Neumeyer, X. Peng, B. I. Knapp, J. M. Bidlack, L. H. Lazarus, S. Salvadori, C. Trapella and G. Balboni, J. Med. Chem., 2006, 49, 5640–5643 CrossRef CAS PubMed.
- A. Bornot, U. Bauer, A. Brown, M. Firth, C. Hellawell and O. Engkvist, J. Med. Chem., 2013, 56, 1197–1210 CrossRef CAS PubMed.
- L. Costantino and D. Barlocco, Curr. Med. Chem., 2012, 19, 3353–3387 CrossRef CAS PubMed.
- O. Witt, H. E. Deubzer, T. Milde and I. Oehme, Cancer Lett., 2009, 277, 8–21 CrossRef CAS PubMed.
- P. A. Marks, R. A. Rifkind, V. M. Richon, R. Breslow, T. Miller and W. K. Kelly, Nat. Rev. Cancer, 2001, 1, 194–202 CrossRef CAS PubMed.
- J. Graeff and L.-H. Tsai, Annu. Rev. Pharmacol. Toxicol., 2013, 53, 311–330 CrossRef PubMed.
- K. T. Andrews, A. Haque and M. K. Jones, Immunol. Cell Biol., 2012, 90, 66–77 CrossRef CAS PubMed.
- D. Rotili, G. Simonetti, A. Savarino, A. T. Palamara, A. R. Migliaccio and A. Mai, Curr. Top. Med. Chem., 2009, 9, 272–291 CrossRef CAS PubMed.
- P. D. Gluckman, M. A. Hanson, T. Buklijas, F. M. Low and A. S. Beedle, Nat. Rev. Endocrinol., 2009, 5, 401–408 CrossRef CAS PubMed.
- M. Haberland, R. L. Montgomery and E. N. Olson, Nat. Rev. Genet., 2009, 10, 32–42 CrossRef CAS PubMed.
- H. Lehrmann, L. L. Pritchard and A. Harel-Bellan, Adv. Cancer Res., 2002, 86, 41–65 CrossRef CAS PubMed.
- D. M. Fass, M. M. Kemp, F. A. Schroeder, F. F. Wagner, Q. Wang and E. B. Holson, Epigenetics Regulation and Epigenomics: Advances in Molecular Biology and Medicine, Wiley-VCH-Verlag & Co. KGaA, Weinheim, Germany, 2012, pp. 515–561 Search PubMed.
- I. V. Gregoretti, Y. M. Lee and H. V. Goodson, J. Mol. Biol., 2004, 338, 17–31 CrossRef CAS PubMed.
- E. Pontiki and D. Hadjipavlou-Litina, Med. Res. Rev., 2012, 32, 1–165 CrossRef CAS PubMed.
- M. Paris, M. Porcelloni, M. Binaschi and D. Fattori, J. Med. Chem., 2008, 51, 1505–1529 CrossRef CAS PubMed.
- A. A. Lane and B. A. Chabner, J. Clin. Oncol., 2009, 27, 5459–5468 CrossRef CAS PubMed.
- S. Minucci and P. G. Pelicci, Nat. Rev. Cancer, 2006, 6, 38–51 CrossRef CAS PubMed.
- P. A. Marks, Oncogene, 2007, 26, 1351–1356 CrossRef CAS PubMed.
- C. Grant, F. Rahman, R. Piekarz, C. Peer, R. Frye, R. W. Robey, E. R. Gardner, W. D. Figg and S. E. Batest, Expert Rev. Anticancer Ther., 2010, 10, 997–1008 CrossRef CAS PubMed.
- C. Tang, C. Li, S. Zhang, Z. Hu, J. Wu, C. Dong, J. Huang and H.-B. Zhou, J. Med. Chem., 2015, 58, 4550–4572 CrossRef CAS PubMed.
- W. Duan, J. Li, E. S. Inks, C. J. Chou, Y. Jia, X. Chu, X. Li, W. Xu and Y. Zhang, J. Med. Chem., 2015, 58, 4325–4338 CrossRef CAS PubMed.
- T. Ai, H. Cui and L. Chen, Curr. Med. Chem., 2012, 19, 475–487 CrossRef CAS PubMed.
- S.-Y. Seo, Arch. Pharmacal Res., 2012, 35, 197–200 CrossRef CAS PubMed.
- W. Guerrant, V. Patil, J. C. Canzoneri and A. K. Oyelere, J. Med. Chem., 2012, 55, 1465–1477 CrossRef CAS PubMed.
- L. Chen, R. Petrelli, G. Gao, D. J. Wilson, G. T. McLean, H. N. Jayaram, Y. Y. Sham and K. W. Pankiewicz, Bioorg. Med. Chem., 2010, 18, 5950–5964 CrossRef CAS PubMed.
- L. E. Tavera-Mendoza, T. D. Quach, B. Dabbas, J. Hudon, X. Liao, A. Palijan, J. L. Gleason and J. H. White, Proc. Natl. Acad. Sci. U. S. A., 2008, 105, 8250–8255 CrossRef CAS PubMed.
- X. Cai, H.-X. Zhai, J. Wang, J. Forrester, H. Qu, L. Yin, C.-J. Lai, R. Bao and C. Qian, J. Med. Chem., 2010, 53, 2000–2009 CrossRef CAS PubMed.
- S. Mahboobi, S. Dove, A. Sellmer, M. Winkler, E. Eichhorn, H. Pongratz, T. Ciossek, T. Baer, T. Maier and T. Beckers, J. Med. Chem., 2009, 52, 2265–2279 CrossRef CAS PubMed.
- D. H. Khan, S. He, J. Yu, S. Winter, W. Cao, C. Seiser and J. R. Davie, J. Biol. Chem., 2013, 288, 16518–16528 CrossRef CAS PubMed.
- S. Pluemsampant, O. S. Safronova, K.-i. Nakahama and I. Morita, Int. J. Cancer, 2008, 122, 333–341 CrossRef CAS PubMed.
- S. C. Tsai and E. Seto, J. Biol. Chem., 2002, 277, 31826–31833 CrossRef CAS PubMed.
- G. Lolli, L. A. Pinna and R. Battistutta, ACS Chem. Biol., 2012, 7, 1158–1163 CrossRef CAS PubMed.
- D. W. Litchfield, Biochem. J., 2003, 369, 1–15 CrossRef CAS PubMed.
- N. A. St-Denis and D. W. Litchfield, Cell. Mol. Life Sci., 2009, 66, 1817–1829 CrossRef CAS PubMed.
- K. A. Ahmad, G. Wang, G. Unger, J. Slaton and K. Ahmed, in Advances in Enzyme Regulation, ed. G. Weber, C. E. ForrestWeber and L. Cocco, 2008, vol. 48, pp. 179–187 Search PubMed.
- B. Guerra and O.-G. Issinger, Curr. Med. Chem., 2008, 15, 1870–1886 CrossRef CAS PubMed.
- G. M. Unger, A. T. Davis, J. W. Slaton and K. Ahmed, Curr. Cancer Drug Targets, 2004, 4, 77–84 CrossRef CAS PubMed.
- G. Cozza, L. A. Pinna and S. Moro, Curr. Med. Chem., 2013, 20, 671–693 CrossRef CAS PubMed.
- G. Cozza, C. Girardi, A. Ranchio, G. Lolli, S. Sarno, A. Orzeszko, Z. Kazimierczuk, R. Battistutta, M. Ruzzene and L. A. Pinna, Cell. Mol. Life Sci., 2014, 71, 3173–3185 CrossRef CAS PubMed.
- R. Battistutta, Cell. Mol. Life Sci., 2009, 66, 1868–1889 CrossRef CAS PubMed.
- S. Sarno, S. Moro, F. Meggio, G. Zagotto, D. Dal Ben, P. Ghisellini, R. Battistutta, G. Zanotti and L. A. Pinna, Pharmacol. Ther., 2002, 93, 159–168 CrossRef CAS PubMed.
- J. Raaf, B. Guerra, I. Neundorf, B. Bopp, O.-G. Issinger, J. Jose, M. Pietsch and K. Niefind, ACS Chem. Biol., 2013, 8, 901–907 CrossRef CAS PubMed.
- R. Prudent and C. Cochet, Chem. Biol., 2009, 16, 112–120 CrossRef CAS PubMed.
- F. Pierre, P. C. Chua, S. E. O'Brien, A. Siddiqui-Jain, P. Bourbon, M. Haddach, J. Michaux, J. Nagasawa, M. K. Schwaebe, E. Stefan, A. Vialettes, J. P. Whitten, T. K. Chen, L. Darjania, R. Stansfield, K. Anderes, J. Bliesath, D. Drygin, C. Ho, M. Omori, C. Proffitt, N. Streiner, K. Trent, W. G. Rice and D. M. Ryckman, J. Med. Chem., 2011, 54, 635–654 CrossRef CAS PubMed.
- R. Battistutta, G. Cozza, F. Pierre, E. Papinutto, G. Lolli, S. Sarno, S. E. O'Brien, A. Siddiqui-Jain, M. Haddach, K. Anderes, D. M. Ryckman, F. Meggio and L. A. Pinna, Biochemistry, 2011, 50, 8478–8488 CrossRef CAS PubMed.
- R. Swider, M. Maslyk, J. M. Zapico, C. Coderch, R. Panchuk, N. Skorokhyd, A. Schnitzler, K. Niefind, B. de Pascual-Teresa and A. Ramos, RSC Adv., 2015, 5, 72482–72494 RSC.
- P. Thirumurugan, D. Matosiuk and K. Jozwiak, Chem. Rev., 2013, 113, 4905–4979 CrossRef CAS PubMed.
- M. A. Pagano, M. Andrzejewska, M. Ruzzene, S. Sarno, L. Cesaro, J. Bain, M. Elliott, F. Meggio, Z. Kazimierczuk and L. A. Pinna, J. Med. Chem., 2004, 47, 6239–6247 CrossRef CAS PubMed.
- R. Swider, M. Maslyk, S. Martin-Santamaria, A. Ramos and B. de Pascual-Teresa, Mol. Cell. Biochem., 2011, 356, 117–119 CrossRef CAS PubMed.
- J. M. Zapico, P. Serra, J. Garcia-Sanmartin, K. Filipiak, R. J. Carbajo, A. K. Schott, A. Pineda-Lucena, A. Martinez, S. Martin-Santamaria, B. de Pascual-Teresa and A. Ramos, Org. Biomol. Chem., 2011, 9, 4587–4599 CAS.
- A. L. Edinger and C. B. Thompson, Curr. Opin. Cell Biol., 2004, 16, 663–669 CrossRef CAS PubMed.
- I. Vermes, C. Haanen, H. Steffensnakken and C. Reutelingsperger, J. Immunol. Methods, 1995, 184, 39–51 CrossRef CAS PubMed.
- A. Vannini, C. Volpari, P. Gallinari, P. Jones, M. Mattu, A. Carfi, R. De Francesco, C. Steinkuehler and S. Di Marco, EMBO Rep., 2007, 8, 879–884 CrossRef CAS PubMed.
- J. R. Somoza, R. J. Skene, B. A. Katz, C. Mol, J. D. Ho, A. J. Jennings, C. Luong, A. Arvai, J. J. Buggy, E. Chi, J. Tang, B. C. Sang, E. Verner, R. Wynands, E. M. Leahy, D. R. Dougan, G. Snell, M. Navre, M. W. Knuth, R. V. Swanson, D. E. McRee and L. W. Tari, Structure, 2004, 12, 1325–1334 CrossRef CAS PubMed.
- D. P. Dowling, S. L. Gantt, S. G. Gattis, C. A. Fierke and D. W. Christianson, Biochemistry, 2008, 47, 13554–13563 CrossRef CAS PubMed.
- K. Vanommeslaeghe, C. Van Alsenoy, F. De Proft, J. C. Martins, D. Tourwe and P. Geerlings, Org. Biomol. Chem., 2003, 1, 2951–2957 CAS.
- K. Niefind, M. Putter, B. Guerra, O. G. Issinger and D. Schomburg, Nat. Struct. Biol., 1999, 6, 1100–1103 CrossRef CAS PubMed.
- R. Battistutta, E. De Moliner, S. Sarno, G. Zanotti and L. A. Pinna, Protein Sci., 2001, 10, 2200–2206 CrossRef CAS PubMed.
- R. Battistutta, M. Mazzorana, L. Cendron, A. Bortolato, S. Sarno, Z. Kazimierczuk, G. Zanotti, S. Moro and L. A. Pinna, ChemBioChem, 2007, 8, 1804–1809 CrossRef CAS PubMed.
- R. Szyszka, N. Grankowski, K. Felczak and D. Shugar, Biochem. Biophys. Res. Commun., 1995, 208, 418–424 CrossRef CAS PubMed.
- Y. Zhou, S. Wang, K. Zhang and X. Jiang, Angew. Chem., Int. Ed., 2008, 47, 7454–7456 CrossRef CAS PubMed.
- K. Barral, A. D. Moorhouse and J. E. Moses, Org. Lett., 2007, 9, 1809–1811 CrossRef CAS PubMed.
- M. C. Peitsch, Bio. Tech., 1995, 13, 723 Search PubMed.
- F. Kiefer, K. Arnold, M. Kuenzli, L. Bordoli and T. Schwede, Nucleic Acids Res., 2009, 37, D387–D392 CrossRef CAS PubMed.
- K. Arnold, L. Bordoli, J. Kopp and T. Schwede, Bioinformatics, 2006, 22, 195–201 CrossRef CAS PubMed.
- J. C. Gordon, J. B. Myers, T. Folta, V. Shoja, L. S. Heath and A. Onufriev, Nucleic Acids Res., 2005, 33, W368–W371 CrossRef CAS PubMed.
- W. D. Cornell, P. Cieplak, C. I. Bayly, I. R. Gould, K. M. Merz, D. M. Ferguson, D. C. Spellmeyer, T. Fox, J. W. Caldwell and P. A. Kollman, J. Am. Chem. Soc., 1995, 117, 5179–5197 CrossRef CAS.
- C. J. Millard, P. J. Watson, I. Celardo, Y. Gordiyenko, S. M. Cowley, C. V. Robinson, L. Fairall and J. W. Schwabe, Mol. Cell, 2013, 51, 57–67 CrossRef CAS PubMed.
- S. H. Hilal, S. W. Karickhoff and L. A. Carreira, Quant. Struct.-Act. Relat., 1995, 14, 348–355 CrossRef CAS.
- R. A. Friesner, R. B. Murphy, M. P. Repasky, L. L. Frye, J. R. Greenwood, T. A. Halgren, P. C. Sanschagrin and D. T. Mainz, J. Med. Chem., 2006, 49, 6177–6196 CrossRef CAS PubMed.
- T. A. Halgren, R. B. Murphy, R. A. Friesner, H. S. Beard, L. L. Frye, W. T. Pollard and J. L. Banks, J. Med. Chem., 2004, 47, 1750–1759 CrossRef CAS PubMed.
- R. A. Friesner, J. L. Banks, R. B. Murphy, T. A. Halgren, J. J. Klicic, D. T. Mainz, M. P. Repasky, E. H. Knoll, M. Shelley, J. K. Perry, D. E. Shaw, P. Francis and P. S. Shenkin, J. Med. Chem., 2004, 47, 1739–1749 CrossRef CAS PubMed.
- W. L. Jorgensen, J. Chandrasekhar, J. D. Madura, R. W. Impey and M. L. Klein, J. Chem. Phys., 1983, 79, 926–935 CrossRef CAS.
- J. Aqvist, J. Phys. Chem., 1990, 94, 8021–8024 CrossRef.
- C. Hansch, J. Comput.-Aided Mol. Des., 2011, 25, 495–507 CrossRef CAS PubMed.
- M. A. Lill, Biochemistry, 2011, 50, 6157–6169 CrossRef CAS PubMed.
- T. Fraczyk, K. Kubinski, M. Maslyk, J. Ciesla, U. Hellman, D. Shugar and W. Rode, Bioorg. Chem., 2010, 38, 124–131 CrossRef CAS PubMed.
Footnote |
† Electronic supplementary information (ESI) available: PyMOL representation of the binding of a substrate-like molecule to HDAC-8 as found in 2V5W; the PyMOL representation of the binding of an ATP analog and two bromobenzimiazole derivatives to CK2; the PyMOL representation of the best docking poses of compounds 5a to 5h bound to HDAC1 and CK2; , the graphical representation of the number of choline atoms that interact with the vicinity of the Mg2+ atom in the CK2 complexes; and the PyMOL superimpositions of the HDAC1 structure obtained with SWISSMODEL and the crystal structure deposited under PDB code: 4BKX. 1H and 13C NMR spectra of compounds 5a–5h. See DOI: 10.1039/c6ra09717k |
|
This journal is © The Royal Society of Chemistry 2016 |
Click here to see how this site uses Cookies. View our privacy policy here.