DOI:
10.1039/C6RA09677H
(Paper)
RSC Adv., 2016,
6, 57084-57097
One-step synthesis of silver metallosurfactant as an efficient antibacterial and anticancer material†
Received
14th April 2016
, Accepted 6th June 2016
First published on 7th June 2016
Abstract
In this work a silver based double chained metallosurfactant was synthesized and characterized using Fourier transform infrared (FTIR), elemental analyses, X-ray diffraction (XRD), differential scanning calorimetry (DSC), nuclear magnetic resonance (NMR), and thermogravimetry. The rate of decomposition of the Ag metal complex was estimated using the non-isothermal thermogravimetry (TG) method. Similar adducts had been prepared in the past to generate colloidal nanoparticles, however, here we are concentrating on the surface and self aggregation properties of the metal surfactant complex. The emphasis is laid on the aggregation behavior being affected by the presence of metal ions. The formation of metallomicelles was authenticated by transmission electron microscopy (TEM), atomic force microscopy (AFM), conductivity and surface tension measurements. The formed metallosurfactant was analyzed for its binding with calf thymus DNA (CT-DNA) using UV-visible and fluorescence spectroscopy. Furthermore, the potential of the as-synthesized metallosurfactant (CTA–AgB) as an antimicrobial and anticancer agent was evaluated. The anticancer activity was estimated with human leukemia HL-60, pancreatic MIA-Pa-Ca-2 and prostate cancer PC-3 cells using the MTT assay. For antimicrobial activity, a Gram-negative bacterium Escherichia coli and a Gram-positive bacterium Staphylococcus aureus and fungi strains, Aspergillus niger, Aspergillus fumigatus, Cladosporium herbarium, Curvularia lunata, Helminthosporium oryzae were used. Along with the concentration effect, mechanism of action of as-synthesized metallosurfactant against various microbial strains was explored using transmission electron microscopy analyses.
Introduction
Metal ions have a very crucial role in a number of biochemical/biophysical processes.1 Metals show distinctiveness in redox activity, variable coordination modes, variable reactivity and intracellular availability. Apart from this, they are potential candidates as anticancer agents. The driving force towards the applicability of metal-containing compounds as anticancer drugs has been revolutionized with cisplatin.2 However, cisplatin suffered from various disadvantages for example toxicity towards healthy cells, its chemical instability (subject to facile hydrolysis under low Cl− and/or low pH conditions), and its poor water solubility.3,4 Various attempts have been made to increase the therapeutic efficacy of cisplatin and other anti-cancer drugs by solubilizing them in hydrophilic polymers or by embedding in liposomes or functionalized nanoparticles.5–9
To comprehend, the dual functional moieties have raised the interest of researchers, one of such kind are metallosurfactants (metal based surfactants). Metallosurfactants offer advantages of metals which imparts properties such as acid–base, redox and magnetic and also give amphiphilic character to the molecule. The metal based colloidal assemblies itself possesses therapeutic activity and at the same time can act as a carrier if required. Although the property of micellization of conventional anionic surfactants in the presence of divalent metal ions in aqueous solution was known for quite some time but a metallosurfactant containing a surfactant in a solid state was reported by Fallis et al.10 for the first time in 1998. The physicochemical properties, aggregation behavior and biological activity of metallosurfactant depend on the type of metal present and structure of metallosurfactant,11 however, they sometimes suffer from poor water solubility.12 Over the years, metallosurfactants have been synthesized and have found applications in diverse fields such as catalysis, anti-cancer activity, the formation of lamellar superstructures, DNA templates, and templates for metallic mesostructures, etc.11–14 For example, the surfactant–cobalt(III) complex (metallosurfactant) cis-[Co(trien)(C14H29NH2)Cl](ClO4)2 was evaluated for its cytotoxicity on the MCF-7 breast cancer cells.15 It was found that the complex possesses antiproliferative and apoptosis–induction properties and causes damage to DNA. On the other hand, Adawy and Khowdiary reported antimicrobial activities of various cationic metallosurfactants.16 Likewise, other multifunctional materials have also been reported for e.g. a multifunctional block copolymer assembly was fabricated by Osada et al.17 that along with fluorescent imaging of the tumor, possesses an appreciable antitumor activity as well. Zhang et al.18 have prepared antibacterial vesicles from a block copolymer that act as a carrier for an anticancer drug.
The aim of the present work is to optimize a dual functional assembly of metallosurfactant that can self-aggregate and have biological activity. Therefore, in this work we had prepared a silver based metallosurfactant that can self-assemble and can act as a carrier (if required) and also have therapeutic activity (i.e. anticancer). But most importantly it possesses anti-microbial activity so that the enhanced efficacy can be achieved by fighting with the microbial infections during or after chemotherapy, which is a commonly observed. For enhanced activity the choice of metal was crucial and for the purpose silver was chosen because of well known biocidal effects of silver ions, its compounds, colloids, and nanoparticles.19,20 Furthermore, it was extremely important to understand the mechanism of action of silver as metallosurfactant against various microbial strains.
Long chain alkylamines are widely used as the templates to synthesize the mesoporous materials in single or binary solutions because they could self-assemble into different structures.21–23 A cetyltrimethylammonium silver bromide complex was reported by Liu et al., where the reaction of silver nitrate with cetyltrimethylammonium bromide (CTAB) in aqueous solution at room temperature was performed, by controlling the concentration and the molar ratio of CTAB to silver nitrate.24
Thereby, keeping in view the importance, a dual-functionalized double chain cationic silver based water soluble metallosurfactant was synthesized. To achieve this, a straightforward, high yielding protocol was developed using ligand insertion method. The surfactant was characterized using elemental analyzes, FTIR, NMR, TGA, and XRD. Further, the self-aggregation property of the metallosurfactant was determined by using a combined approach, where self aggregation was studied using surface tension, conductivity and morphology with TEM and AFM. The bioactivity profile of this complex was investigated on cancer (pancreatic, prostate and leukemia) and normal cell lines using MTT assay. The antimicrobial properties were also evaluated on different bacteria and fungi samples using well diffusion assay. To account for, its anticancer and antimicrobial properties, its interaction with calf thymus DNA (CT-DNA) was also evaluated spectrophotometrically. The results obtained have shown that the bioactivity was enhanced with the incorporation of the silver counter ion. Moreover, the silver based metallosurfactants are found to be less toxic because four times high IC50 value in normal cells was obtained in comparison to cancer cells.
Material and methods
AgNO3 (99.0%), CTAB (99.0%), calf thymus DNA (purity ≥ 98%), ethidium bromide (EB) (purity ≈ 95%), tetramethylsilane (99.4%) EDTA, DMSO, DCM, tetrazolium dye MTT 3-(4,5-dimethylthiazol-2-yl)-2,5-diphenyltetrazolium bromide, DMEM (Dulbecco's Modified Eagle's Medium), EMEM media containing 5% foetal bovine serum (FBS), NAD(P)H, MEM medium containing 10% FCS trypsin, RPMI-1640, kanamycin, streptomycin and CDCl3 (99.2%) were purchased from Sigma Aldrich. Absolute ethanol (99.9%) was purchased from Changshu Yuang. Nutrient agar and potato dextrose agar were purchased from HiMedia. The fungal strains Curvularia lunata (ITCC 6257) Helminthosporium oryzae (ITCC 5559), Aspergillus fumigates (ITCC 1628), Aspergillus niger (ITCC 302), Cladosporium herbarum (ITCC 3137), used for the present study were procured from the Indian Type Culture Collection (ITCC), Indian Agriculture Research Institute (IARI), New Delhi, India. Escherichia coli (MTCC 40) and Staphylococcus aureus (MTCC 2901) were obtained from IMTECH Chandigarh. Human leukemia HL-60, pancreatic MIA-Pa-Ca-2, prostate cancer PC-3 cells and normal cells (fR2 human breast epithelial cells) were obtained from ECACC, UK.
Precautions were taken while handling Ethidium bromide. The safety data sheets were also consulted for DMSO and DCM.
Procedure for the formation of metallocomplex
The Ag metallocomplex (CTA–AgB) was prepared by refluxing the metal salt (0.5 mmol) and ligand i.e. CTAB (1 mmol) in 1
:
2 ratio. The AgNO3 was dissolved in absolute ethanol (5 mL) with subsequent addition of ligand i.e. CTAB. The mixture was stirred for 2 h at 150 rpm. The product is obtained after evaporating the solvent. Recrystallization of the final powdered product was carried out in methanol.
Physical measurements. Eager Xperience CHN was used for elemental (CHN) analyses. The experiment was performed under inert nitrogen medium. FTIR spectra were recorded on Perkin Elmer (RX1) spectrometer in the far-IR range of 200 to 600 nm and IR range from 600 to 3500 nm. For each spectrum, 24 scans were recorded with a spectral resolution of 4 cm−1 on KBr pellets. Thermogravimetric analyses (TGA) were carried out using SDT-Q-600 (TA instruments). A sample mass (approximately 5–10 mg) was transferred to aluminum crucibles and analyzed from room temperature to 1000 °C at a heating rate of 10 °C min−1 under the nitrogen atmosphere. Differential Scanning Calorimetry (DSC) measurements were done using TA (Q20) in aluminum pans with the blank pan as a reference (maintained at the same temperature). The samples were rapidly cooled in liquid nitrogen up to −100 °C. After equilibration, the samples were heated at 10 °C min−1 to reach the ambient temperatures. X-Ray Diffraction (XRD) spectra were recorded on Panalytical X'Pert Pro X-ray diffractometer equipped with Cu-kα radiation (1.5406 Å) operating at 40 kV, with a scanning speed of 8° min−1 to examine the crystalline phase of the sample. For 1H NMR studies, JEOL FT-NMR, AL 300 was employed with 300 MHz radio frequency and 7.05 tesla magnetic field strength. Tetramethylsilane was used as internal standard and D2O was used as a solvent.
Self aggregation. Critical micellization concentrations (cmc) were determined through conductivity measurements performed on Pico Lab India Digital conductivity meter using doubly distilled water (conductivity less than 5μS cm−1). These studies were carried out for a temperature range of 25–40 °C. The measuring cell and sample were dip into jacket whose temperature (±0.01 °C) was maintained through the thermostat. Surface tension of prepared metal complex was measured at 25 °C with Du Nouy tensiometer (Kruss type 8451) using double distilled water. The surface tension of the used double distilled water was 71 mN m−1 at 25 °C. TEM micrographs of metallomicelles were obtained using Hitachi (H 7500) instrument at an accelerating voltage of 90 kV. Freshly prepared samples were gently placed over 200-mesh formwar copper grid uniformly coated with carbon film. AFM studies were performed using Bruker Nanoscope “V-multimode 8” with an operating frequency of 312.1309 kHz and tapping mode was used for image scanning. Multiply filtered metalomicelles were gently sonicated for 2 h prior to sample preparation for AFM study. An aqueous solution of metallomicelles was gently poured on ultrapure silicon wafer 1 cm × 1 cm and was dried overnight in a vacuum desiccator to obtain a thin homogeneous coating of metalomicelles. UV-visible absorption spectra were recorded on Perkin Elmer spectrophotometer with matched pair of 1 cm quartz cell fitted in thermostatic cell holder. Absorption spectra were carried out between 200 and 800 nm. Fluorimetric studies of CT-DNA were carried out in the presence of a variable concentration of metallosurfactant on Hitachi F-7000 Photo luminescence spectrophotometer. The excitation wavelength was set at 450 nm, with a slit width of 10 nm and range of observation was selected between 500 and 700 nm.
Antimicrobial studies
In vitro antimicrobial tests were carried out using well diffusion assay against different microorganisms.25,26 Bacteria were cultured in nutrient agar broth and fungus was cultured in potato dextrose agar broth media incubated at 37 and 25 °C respectively for 24 h. 60 μL (0.5 mg mL−1, aqueous) of test samples were added to 6 mm well bored on agar plates after homogeneous pouring of freshly cultured microbial stains. After overnight incubation, diameters of inhibition zones were measured in nearest of millimeters and the average value of triplicate measurements was reported. The diameter of the well was excluded from inhibition zone diameter. Effect of concentration on microbial growth was also studied by using multiple dilutions of the test samples. Mechanism of action of synthesized metallosurfactant against various microbial strains was studied using TEM microscopy by checking interaction of metallosurfactant and microbe at regular time intervals.
Cytotoxic studies
Cell culture and treatment. The cells were grown in RPMI-1640, DMEM and MEM medium containing 10% FCS, 100 μg mL−1 kanamycin and streptomycin. Cells were grown in a CO2 incubator (Thermocon Electron Corporation, USA) at 37 °C with 5% CO2 gas environment and 95% humidity. Cells grown in monolayer cultures were trypsinised with trypsin (0.1% w/v)/EDTA (1 mM) solution. Soon after cells were ready to detach, the trypsin/EDTA solution was removed. Cells were dispersed gently by pipetting in complete growth medium, centrifuged at 200 × g, for 5 min. Cells were dispersed in a complete medium in culture flasks and incubated in the CO2 incubator. Cells grown in semi-confluent stage (approx. 70% confluent) were treated with CTA–AgB dissolved in DMSO while the untreated control cultures received only the vehicle (DMSO, <0.2%).
MTT cell proliferation assay. This assay is a quantitative colorimetric method for the determination of cell survival and proliferation. This assay is a colorimetric assay for assessing cell viability. NAD(P)H-dependent cellular oxidoreductase enzymes may, under defined conditions, reflect the number of viable cells present. These enzymes are capable of reducing the tetrazolium dye MTT 3-(4,5-dimethylthiazol-2-yl)-2,5-diphenyltetrazolium bromide to its insoluble formazan, which has a purple color. Tetrazolium dye assays can also be used to measure cytotoxicity (loss of viable cells) or cytostatic activity (shift from proliferation to quiescence) of potential medicinal agents and toxic materials. The assessed parameter is the metabolic activity of viable cells. Metabolically active cells reduce pale yellow tetrazolium salt (MTT) to a dark blue water-insoluble formazan, which can be, after solubilization with DMSO, directly quantified. The absorbance of the formazan directly correlates to the number of viable cells. The cells were seeded at a density of 15
000(HL-60), 6000(PC-3) and 5000(MIA-Pa-Ca-2) plated in 96-well plates. Cultures were incubated with 1, 3, 5, 7 and 10 μM concentrations of test materials and incubated for 48 h. After 44 h, 20 μL of MTT dye was added at a concentration of 2.5 mg mL−1 for 4 h. The supernatant was aspirated and MTT-formazon crystals dissolved in 150 μL of DMSO; optical density was measured at a wavelength of 540 nm (reference wavelength, λ 620 nm) on an ELISA reader (Thermo Laboratories, U.S.A). Cell growth was calculated by comparing the absorbance of treated versus untreated cells.27
Results and discussion
To prepare CTA–AgB complex, 1
:
2 ratio of silver nitrate to CTAB was used, which readily resulted into formation of white colored CTA–AgB complex due to enhanced surface adsorption without formation of silver bromide nanoparticles (yellow in colour).24 Moreover, the formation of nanoparticles occurs at a lower molar ratio of CTAB (for e.g. 1
:
0.5), and it has been reported that for ratio more than 1
:
1.5 only complexation takes place.24 The structure of the complex is given in Fig. 1(a) and characterized with the help of various analytical techniques. The elemental analysis (found: C = 51.20, H = 9.49, N = 4.86) is in good agreement with the calculated value (calcd: C = 50.73, H = 9.35, N = 4.67).
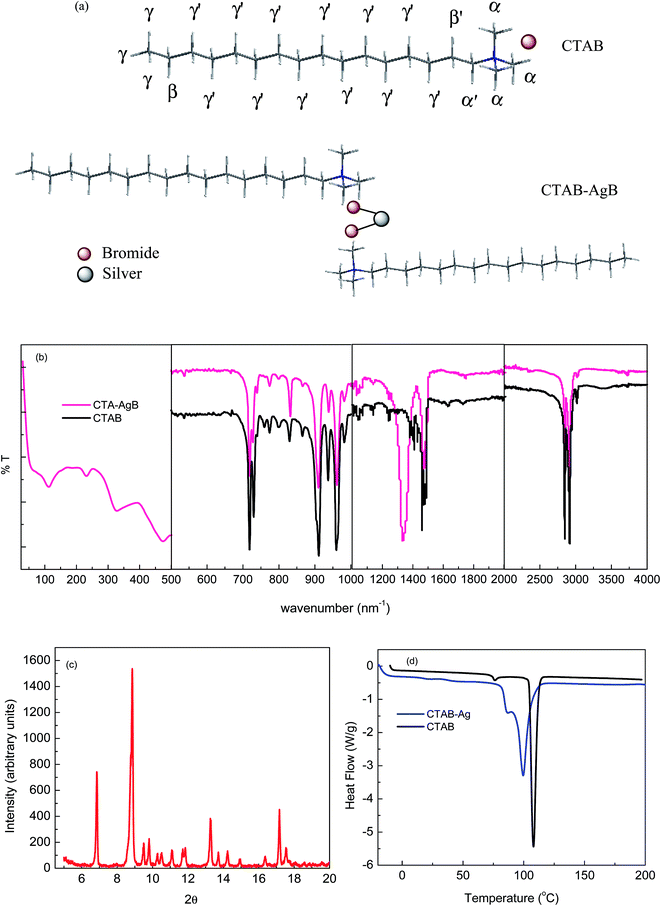 |
| Fig. 1 (a) Structural representation of CTAB and CTA–AgB (b) FTIR (50–4000 cm−1) of CTAB and CTA–AgB (c) XRD spectra of CTA–AgB (d) DSC of CTAB and CTA–AgB. | |
The complexation of ligand to metal salt was confirmed by comparing the FTIR spectra of the pure ligand with metallocomplex. Fig. 1(b) depicts the spectra of pure ligand and the prepared metallocomplex. Table S1† gives the FTIR data of pure ligand and metallocomplex. CTAB has 16 carbon chain, therefore, the two intense bands at around 2915 and 2848 cm−l are assigned to asymmetric and symmetric stretching vibrations of C–CH2 from the methylene chains, respectively. After complexation with a metal salt, no change was observed in the hydrophobic chains of ligand. For CTAB, the bands at 1484 and 1473 cm−1 correspond to asymmetric and symmetric CH scissoring vibrations of CH3–N+. A shift from 1473 to 1464 cm−1 was observed on complexation.28 Bands at 1382 and 1258 cm−1 represent the N–CH3 symmetric stretching vibrations. The complexation of AgNO3 and CTAB is well adjudged from the shifts in peaks from two bands 1407 and 1382 cm−1 to a single peak at 1332 cm−1, whereas 1258 cm−1 disappears completely. No change was observed at 1151, 1037, 959 cm−1 on complexation. Distinctions in the spectra are clearly seen from that of the earlier reported silver capped nanoparticles. The terminal Ag–Br peaks are observed in the far-IR region at 170, 215 and 49 cm−1.29 In the present complex, the bridge Br stretching vibrations at 231 and 112 cm−1 further confirms the complexation (Fig. 1b).30 The metallosurfactant was further characterized by XRD and DSC (Fig. 1(c) and (d)). Similar XRD pattern and DSC curves have been obtained as reported,22,24,31 CTAB exhibited a sharp endothermic peak at 108 °C, whereas, the peak for metallosurfactant appeared at 98 °C which attributed to the melting of the ordered regions of hydrocarbon chains in pure CTAB and CTA–AgB. In NMR spectra of CTAB (Fig. S1(a)†), chemical shift at 3.082 and 3.338 was assigned to α-CH3 and α′-CH2, respectively. For β′-CH2 β-CH2, and γ′-CH2, δ was obtained at 1.671, 1.270, and 1.188, respectively. The terminal methyl, γ-CH3, gave chemical shift at 0.765. The comparison of 1HNMR of both CTAB and metallosurfactant (Fig. S1(b)†) was made and Δδ values (Table S2†) clearly depict the change in the position of protons around the head group i.e. α-CH3 and α′-CH2, and the change of counter ions are clearly evident.21
Further, TGA characterization has been carried out and TG curves of pure CTAB and CTA–AgB are given in Fig. 2(a). The TGA curve of pure CTAB initially shows loss of water around 100 °C. CTAB is known to decompose within 220–350 °C.23 The first step of decomposition was observed at 247.15 °C for CTAB and for CTA–AgB, DTA peak at 275.3 °C was obtained. Another event at 426 °C was observed for silver complex which was assigned to the melting of silver bromide crystals. The rate of decomposition of CTAB and its Ag complex has been estimated using the non-isothermal thermogravimetry (TG) method. The values of activation energy, Ea, and reaction order, n of the thermal decomposition were calculated32 using Coats–Redfern (CR), Madhusudanan–Krishnan–Ninan (MKN), Wanjun–Yuwen–Hen–Cunxin (WYHC), van Krevelen (VK), and Horowitz–Metzger (HM) method. All the above mentioned methods are based on a single heating rate and the details of kinetics equations are reported in details in our previously published work.32 The representative linearisation curves and the best linearity, corresponding to the maximum value of the correlation coefficient obtained by all the five methods for CTAB and CTA–AgB are shown in Fig. S2 and S3.† The results indicate that the values of activation energy and regression by employing different methods are comparable and have been presented in Table 1. The order of reaction is found to be zero. The activation energy of CTA–AgB is found to greater than that of CTAB. In the earlier studies, the metal surfactant is found to have smaller activation energies than pure ionic surfactant, which is due to the fact that lesser energy is required for breaking the weaker coordinate bond present in the complex than the ionic bond of the ligand. However, in the present case the higher activation energy of silver based complex is estimated and implies the formation of stronger bond in the silver complex, that accounts for the higher stability of the complex.
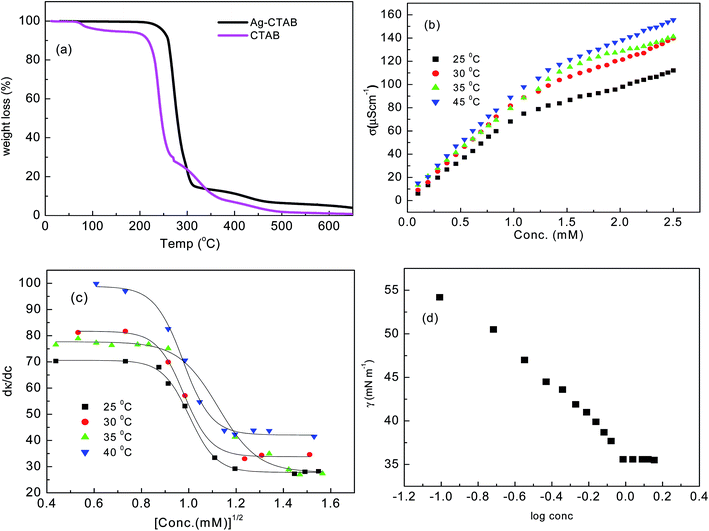 |
| Fig. 2 (a) TGA of CTAB and CTA–AgB (b) variation of specific conductance (c) molar conductance and (d) surface tension with concentration of CTA–AgB. | |
Table 1 TGA data and activation energy using different methods
Complex |
DTG T (°C) |
Methods |
Order |
CR |
MKN |
WYHC |
VK |
HM |
E/kJ mol−1 (R) |
E/kJ mol−1 (R) |
E/kJ mol−1 (R) |
E/kJ mol−1 (R) |
E/kJ mol−1 (R) |
CTAB |
247.15 |
11.81 (0.999) |
11.96 (0.999) |
12.01 (0.999) |
13.85 (0.998) |
15.88 (0.999) |
Zero order |
CTA–AgB |
275.3 |
28.05 (0.999) |
28.21 (0.999) |
28.26 (0.999) |
32.59 (0.999) |
32.58 (0.999) |
Zero order |
Self aggregation
Self aggregation of molecules is outplay of various interactions i.e. hydrophobic interactions (among surfactant tails), electrostatic interactions (repulsive between head groups and attractive with oppositely charged counter ion), hydrogen bonding (among solvent molecules) and sometimes metal ligand interaction (as is the present case).33,34 Micellization is also influenced by other parameters such as temperature, dielectric constant etc.35,36 To understand micellization in the present work, where a metal ion is present as a counter ion having 2
:
1 stoichiometry of hydrophobic chains and counter ions, (which was altered from usual 1
:
1 for CTAB) conductivity and surface tension measurements were performed. Fig. 2(b–d) gives the variation of specific conductance, molar conductance and surface tension with change in the concentration of metallosurfactant. Specific conductance increases with increase in the temperature. The cmc determined by surface tension measurement was in good agreement with the one obtained from conductivity studies. In previously reported work, micellization behavior of metallosurfactants in both aqueous and nonaqueous medium showed a decrease in cmc in comparison to the parent conventional surfactant because of crowding at the interface.32,37,38
Similar behavior was expected for this silver metallosurfactant with AgBr2 as a counter ion because metallic counter ion is bigger in size and is expected to undergo weak hydration, therefore, lower cmc was anticipated but the results obtained were contrary. The cmc of CTAB at 25 °C is reported between 0.84 and 0.97 mM39,40 and CTA–AgB complex displayed almost equivalent cmc values as compared to CTAB except for 40 °C (Table S3†). Jakubowska41 has recently explained counter ion binding with different anionic headgroups, where the types of counter ion (kosmotropic and chaotropic ions) have shown different types of binding to monomers and dimers. Similarly, in this case, Ag being a kosmotropic ion shows strong ion hydration. The kosmotropic ions are usually small, have relatively low polarizabilities, therefore, they lose their water of hydration with great difficulty. These types of ions are strongly screened due to strong ion hydration, the binding of these ions to interfaces by electrostatic forces is weak and is strongly determined by the ion hydration, therefore, results in higher cmc (although slight in this case), than the expected decrease. The increase observed was slight may be because precursor CTAB itself has a large bromide ion, therefore, a significant difference in the cmc values was not observed because of the similar hydration of both the ions.
Fig. S4† gives a variation of different thermodynamic parameters with temperature. The Gibbs free energy (ΔGom), enthalpy (ΔHom) and entropy (ΔSom) of micellization was estimated by phase separation model using eqn (S1)–(S3) given in ESI.† Linear variation was observed for degree of ionization (β), and ΔHom. The surfactant ionization increases with increase in the temperature; this illustrates the decrease in the counter ion binding due to thermally aggravated process. The variations for enthalpy and entropy were quite sensitive to temperature. The value of ΔHom decreases with increase in the temperature and remain negative throughout. However, entropy decreased with temperature and remained positive. ΔGom is the sum of the enthalpic (ΔHom) and entropic (−TΔSom) contributions, with an increase in the temperature, the enthalpic contribution to the free energy increases, whereas the entropic contribution decreases (Fig. S5†), however, the process is overall entropy driven. The Gibbs surface excess at cmc (Γtotmax) was calculated from the linear part of the γ vs. log[Ct] plots, as shown in Fig. 2(d) using eqn (1)
|
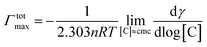 | (1) |
where
n,
R and
T are the number of ionic species per surfactant monomer in solution, the universal gas constant, and absolute temperature, respectively. In this equation, the concentration was used in place of activity since the solutions in use were fairly dilute. The area of exclusion per monomer (
Amin) at the saturated air/solution interface was calculated by using
eqn (2) |
 | (2) |
The surface excess along with minimum area occupied by a head group decides the extent of packing at the air/water interface. In the case of Ag metal surfactant, Γtotmax = ΓCTA + ΓBr− + ΓAg+ + ΓNO3− with a value of 0.75 μmol m−2 and Amin = 221.2 Å2, which in comparison to CTAB (Γtotmax = 1.68 μmol m−2; Amin = 99.2 Å2) was found to be low and this is ascribed to the competitive adsorption between the cationic head group and metallic counter ion. The presence of metal ion at the interface is expected to enhance the steric crowding at the interface. Wherein, micellization in the present case is mainly being controlled by the counter ion hydration.
The micellization of CTA–AgB was viewed using TEM and AFM. Fig. 3 shows the aggregates of metallosurfactant prepared at twice concentration of cmc, with an average size 6 nm (Fig. 3a) and AFM clearly depicted the spherical shape of these aggregates (Fig. 3b). After the characterization of CTA–AgB micelles, its interaction with CT-DNA was estimated using absorption and emission spectroscopy. Fluorescence quenching experiments give us information regarding localization and the mode of binding with DNA. 3,8-Diamino-5-ethyl-6-phenyl-phenanthridinium bromide (EB) a cationic dye is widely used in spectrofluorimetric studies as nondestructive fluorescent intercalator displacement (FID) assay due to fluorescence enhancement it displays upon binding with DNA. Fluorescence enhancement is due to intercalation of the dye into double helix conformation of nucleic acid.42 The continuous decrease in fluorescence intensity with the addition of surfactant (0–45 μM) is indicative of replacement of intercalated EB (4 μM) molecules from DNA (75 μM) (Fig. 3c). It was found that intercalating agents, as well as groove binders, cause a reduction in emission intensity, however, moderately in the present case. Binding affinity was evaluated by applying Stern–Volmer equation and a high Ksv (binding constant) value (3.20 × 104 LM−1) show that metallosurfactant bound strongly to the DNA (Fig. 3d). In UV-visible absorption spectroscopy, with an increase in the concentration of DNA and keeping metallosurfactant at a constant concentration (0.2 mM), hyperchromism effect was observed which indicates the formation of complex with CT-DNA (Fig. 3e). The UV-visible spectra of DNA alone were carried out with increasing concentrations, which did not show any shift (Fig. S6†), thereby confirming hyperchromism effect is due to complexation of DNA with metallosurfactant. This might be because of the electrostatic interactions between metallosurfactant and CT-DNA, which embodies changes in the conformation of CT-DNA. Such increase in absorption intensity is generally due to the exposure of purine and pyrimidine DNA-bases on the binding. Quantitatively binding affinity, as well as intrinsic binding constant Kb, is calculated using the eqn (3)
|
[DNA]/(εa − εf) = [DNA]/(εo− εf) + 1/Kb(εo− εf)
| (3) |
where [DNA] is the concentration of DNA;
εa,
εf and
εo are the apparent, free and fully bound Ag complex extinction coefficients. A plot of [DNA]/(
εa −
εf)
versus [DNA] gives
Kb as the ratio of the slope to intercept. High
Kb values indicate a strong electrostatic interaction between metallosurfactant and CT-DNA (
Fig. 3f).
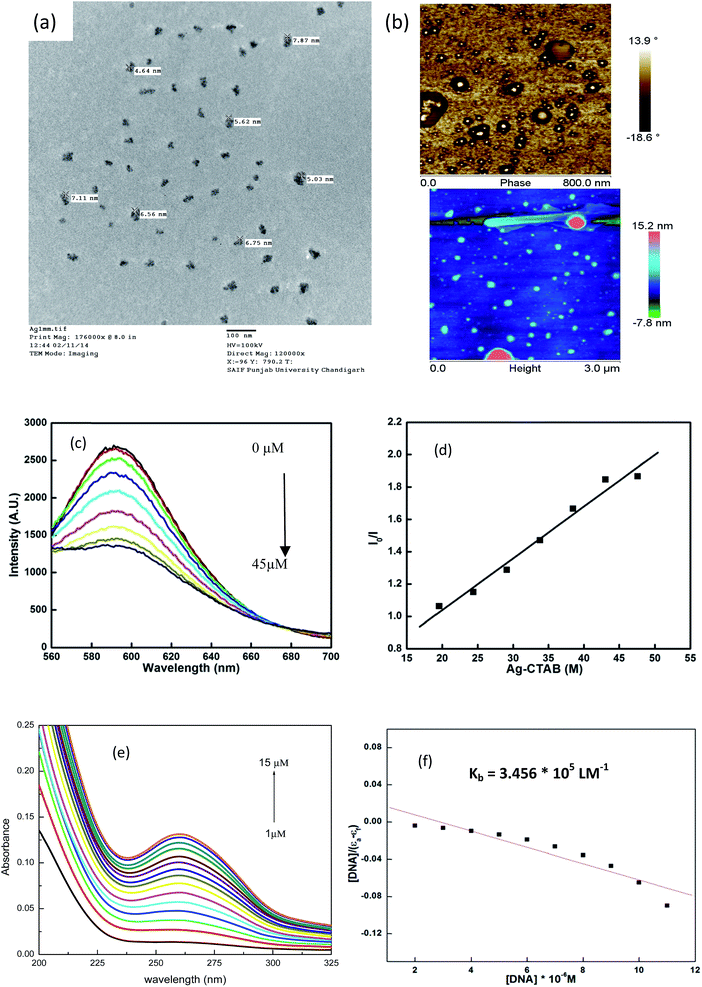 |
| Fig. 3 (a) TEM and (b) AFM of metallomicelles; (c) and (d) CT-DNA interaction with metalomicelles using fluorescence spectroscopy; (e) and (f) using UV-visible spectroscopy. | |
Further, we explored the utility of the silver metallocomplex in killing microbes and cancer cells using in vitro analyses by employing well diffusion and MTT assay, respectively.
Cytotoxicity activity
Nanoscale complexes currently being developed consist of two main components: the nanoparticle itself, which is used as the carrier agent, and the chemotherapeutic drug. Studies using paclitaxel have shown that when compared with the conventional formulation, the nanoparticle formulation of the drug increases both its cytotoxicity profile in cell culture and its therapeutic efficiency in a living animal model. This has been attributed to the nanoparticle formulation having greater bioavailability and a longer sustainable therapeutic time, which allows the drug concentration to remain above the minimum effective value for an extended period of time.43 In the present work we are proposing a dual functional colloidal assembly which itself possess anti-proliferative activity. CTA–AgB complex was tested for anti-proliferative activity in three different cancer cell lines including pancreatic MIA-Pa-Ca-2, prostate PC-3 and human leukemia HL-60 cancer cell lines (Fig. 4), in the concentration range of (0–5 μM/0–4.5 μg mL−1) to find a dose−response relationship. The tested compound had significant cytotoxicity in tested cancer cell lines with IC50 values of 0.45 and 0.3 μM for PC-3 and Mia PaCa-2 cells, respectively. The complex was found to be highly cytotoxic in Mia-Pa-Ca-2 and PC-3 cells with high safety window because it has a four time high IC50 values in normal cells as compare to pancreatic and prostate cancer cells. HL-60 cells were not much effective in comparison to other cancer cell lines used in the present work. However, if the comparison is made with silver Nps used in literature against HL60 cells (Table 2), a much smaller value of IC50 is found using silver metallosurfactant. Similarly, for other cell lines also, a much smaller dose is estimated.44–49
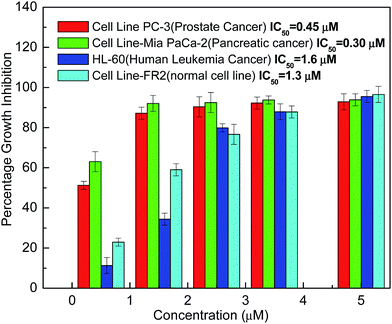 |
| Fig. 4 Percentage growth inhibition of different cancer lines using CTA–AgB as analyzed by MTT assay. | |
Table 2 Comparison of cytotoxicity activity of Ag–metallosurfactant with different Ag nanoparticles (NP) against various cancer cells
Nanoparticles |
Cancer cell lines; assay used |
IC50 or inhibitory dose |
Ref. |
Ag(+) NPs, Ag(−) NPs |
Pancreatic cancer cells (Panc-1); MTS (3-(4,5-dimethylthiazol-2-yl)-5-(3-carboxymethoxyphenyl)-2-(4-sulfophenyl)-2H-tetrazolium, inner salt) assay |
IC50: 720 μg mL−1, 1800 μg mL−1, respectively |
44 |
Ag Nps prepared from extract of Alternanthera sessilis |
Prostate cancer cells (PC3); MTT assay |
IC50: 6.85 μg mL−1, compared to silver ions (14.62 μg mL−1) |
45 |
AgNPs |
Leukemic cells (Jurkat and U937); XTT (2,3-bis[2-methoxy-4-nitro-5-sulfophenyl]-H-tetrazolium-5-carboxyanilide inner salt) assay |
9.8 and 12.6 ppm in Jurkat cells and U937 cells, respectively |
46 |
Ag Nps prepared from extract of Eucalyptus chapmaniana |
Human acute promyelocytic leukemia (HL-60); MTT assay |
IC50: 2 mmol L−1 |
47 |
Nano Ag sol |
Human promyelocytic leukemia cells (HL-60); MTT assay |
Viability cells diminished to 60% at dose of 25 mg L−1 |
48 |
Ag Nps |
Human promyelocytic leukemia cells (HL-60); MTT assay |
Viability cells diminished to 40% at dose of 0.5 mM AgNPs |
49 |
Antimicrobial activity
To access the antimicrobial activity of the CTA–AgB complex, agar well diffusion method was employed. Two bacterial (E. coli; Gram-negative and S. aureus; Gram positive) and five fungal strains (A. niger, A. fumigates, C. herbarium, C. lunata and H. oryzae) were considered for antimicrobial studies. Bacterial strains were grown in nutrient media at a temperature of 37 °C and for fungus, potato dextrose media was used at a temperature of 25 °C. Metallosurfactant in a concentration of 0.5 mg mL−1 were used. Zones of inhibition obtained for this concentration are presented in Table S4,† where the same dose of 0.5 mg mL−1 of silver metallosurfactant gave a comparable average zone of inhibition. Effect of metallosurfactant concentration on microbial growth was also studied by counting visible colonies and obtained results are shown in Table S4† for five different concentrations. At some dilutions the growth of colonies was so vigorous that no margins were observed, such growth was ‘confluent’, represented by ‘C’ in the Table S4.† Moreover, it is also clear from the Table S4† that the primary used concentration (0.5 mg mL−1) is also the MIC (minimum inhibitory concentration) for E. coli species. As far as dilutions of fungi are concerned, lesser growth was observed for A. niger, A. fumigatus than the other fungi used in the study.
Action of synthesized metallosurfactant against microbes
A lot of mechanistic studies have been carried out on the antimicrobial property of silver in different forms i.e. ions,50 nanoparticles, functionalized nanoparticles51–53 etc. Further, to explore the mechanism, TEM analyses were carried out on E. coli (Fig. 5a–e), S. aureus (Fig. 6a–f) and fungi (Fig. 7a–i) on treatment with silver metallosurfactant.
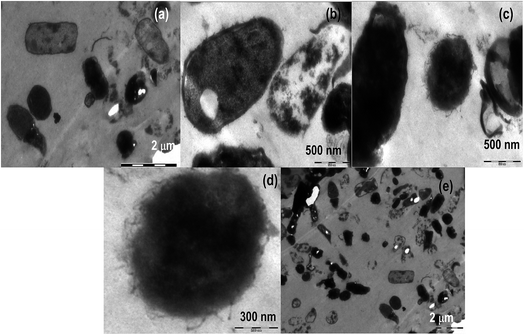 |
| Fig. 5 TEM images of E. coli treated with CTA–AgB metallosurfactant. | |
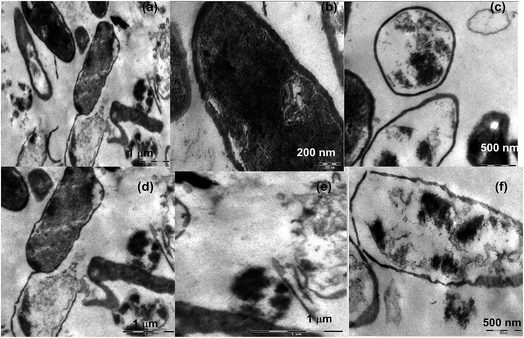 |
| Fig. 6 TEM images of S. aureus treated with CTA–AgB metallosurfactant. | |
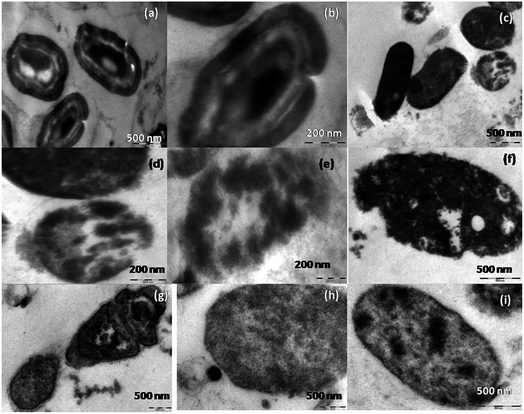 |
| Fig. 7 TEM images of (a and b) A. niger (c–e) A. fumigates (f and g) C. herbarium (h and i) C. lunata with CTA–AgB metallosurfactant. | |
Action of metallosurfactant against E. coli and S. aureus
TEM was used to predict the exact mechanism of action of synthesized metallosurfactant. All microbial species were treated with metallosurfactant and TEM images were captured time to time to see the effect of metallosurfactant on various parts of microbes. In this way, inhibitive mechanism of synthesized metallosurfactant was analyzed and is concluded with the help of TEM images. Electron density in an untreated cell should be homogenous but in treated cells three types of variations are observed. Cells with homogenous light appearance can be seen in TEM images (cells at the upper left border in Fig. 5a). These cells are equivalent to untreated cells and it seems that these cells did not absorb metallosurfactant formulation. This may be due to the fact that these were located in the bulk of cell mass where formulation was unable to access them. Cells with heterogeneous dark appearance are also present in TEM images. These cells have patches of dark appearance, these patches perhaps are cell organelles where formulation got accumulated (left the cell in Fig. 5a and middle cell in Fig. 5a). Cells with homogenous dark appearance can also be seen from TEM images. These cells absorbed the formulation to such an extent that whole of the cell appeared dark and no cell organelles could be seen (left cell in Fig. 5b and 6b). So it can also be inferred that absorption of the formulation is time dependent. Initially, absorption was inside some particular organelles but with the passage of time this control on absorption was lost and formulations got accumulated in the whole of the cell. Location of cells within bulk cell mass during treatment may account the same variation in the localization of formulation. It is clear from the images (Fig. 5a, c and 6c) that the cells are oval in longitudinal section and circular in transverse sections. Membrane perturbation was observed in almost all cells. E. coli is a peritrichous bacterium with flagella all over its surface. These flagella were vanished by the action of metallosurfactant. This happened in a very stepwise manner, as few cells are with small flagella (middle cell in Fig. 5c and d) and many others are without flagella/small flagella. This damage to flagella was also time and location dependent as described above. Further, E. coli with homogenous dark appearance lacked flagella altogether (cells in extreme left in Fig. 5c). All the staphylococcus cells as expected lacked flagellum (Fig. 6a–f). Some cells depicted initial separation of plasma membrane from cell wall while in many others there is complete separation (initial separation in upper cell and complete separation in a lower cell in Fig. 6d). TEM analysis also discerned localized separation. Gram-negative strains (Fig. 5e) were found to be more resistant to metallosurfactant damage than Gram positive bacterial strains (Fig. 6). Metallosurfactant initially came in contact with the cell wall and then subsequently penetrated inside.
Action of metallosurfactant against fungal strains
In the case of fungi strains separation of the cell wall, cell membrane, and nuclear membrane is distinct, unlike bacteria. A large part of cytoplasm did not absorb the metallosurfactant (A. niger in Fig. 7a), perhaps these areas were nuclei as A. niger is supposed to have many nuclei. Cytoplasm had patches but it did not dissolve similar to bacteria whereas cell wall struggled till its last to remain intact and maintained its shape (Aspergillus cells in Fig. 7a and b). In all fungal cells except A. fumigates (Fig. 7c–e) there was no morphological loss to the cell wall. A. fumigates cells were the only fungal cell (of all tested fungal cells) where cell wall gets dissolved and cytoplasm was without a distinct boundary. In C. herbarium (Fig. 7f and g), dividing cells are showing shrinkage in DNA (transverse section of dividing cell in Fig. 7g). In A. niger (Fig. 7b), cell pits are formed, as this cell is appearing heterogeneous dark so it can also be concluded that initially encounter in these cells allowed entry of metallosurfactant by the formation of pits in the cell wall. There is also an accumulation of metallosurfactant at the surface of cells and can be seen from the image (lower A. fumigatus cell in Fig. 7d). These differences in bacterial and fungal cell walls perhaps are due to the different chemical composition of two types of cells. Cytoplasm is less damaged in fungal cells (Fig. 7a–e) than bacterial cells (right cell in Fig. 5c, Fig. 6a, c and d). It is because cytoplasm of fungal cells is more advanced than bacterial cells that maintained the integrity of its cytoplasm even in the presence of metallosurfactant.
Conclusion
A double chained silver based metallosurfactant was synthesized using CTAB as a precursor. After confirming the complexation by various analytical methods, the rate of decomposition of CTAB and its CTA–AgB was estimated using the TGA method. The activation energy of decomposition of CTA–AgB was found to greater be than that of CTAB. The metal based surfactant was evaluated for its surface and self aggregation properties. It was found that cmc remained close to the precursor CTAB even in the presence of silver ions and the formation of metallomicelles is confirmed by TEM, AFM, conductivity and surface tension measurements. The micellization process was thermodynamically favorable with entropy being the driving force and is being influenced by Ag hydration. The binding of metallosurfactant with CT-DNA was estimated using UV-visible and fluorescence spectroscopy, the results reveal a strong electrostatic interaction between the two. CTA–AgB showed potential as an antimicrobial and anticancer agent. Estimation of anticancer activity of metallosurfactant against human leukemia HL-60, pancreatic MIA-Pa-Ca-2 and prostate cancer PC-3 cells using MTT, gave IC50 1.6, 0.3 and 0.45 μM, respectively. Antimicrobial activity was evaluated using bacterial and fungal strains. Although silver over the years has proved to be one of the efficient antimicrobial agents in different forms, however, in this work, it's the dual functionality of silver based metallocomplex which of importance. TEM analyzes were instrumental in understanding the mechanism of antimicrobial activity of the prepared silver metal complex. Gram negative strains were found to be more resistant to damage than Gram positive bacterial strains. Among fungi and bacteria, cytoplasm is less damaged in fungal cells in comparison to bacterial cells. Scheme 1 gives the pictorial representation of the formation of the silver metallosurfactant and its potential applications explored in this work.
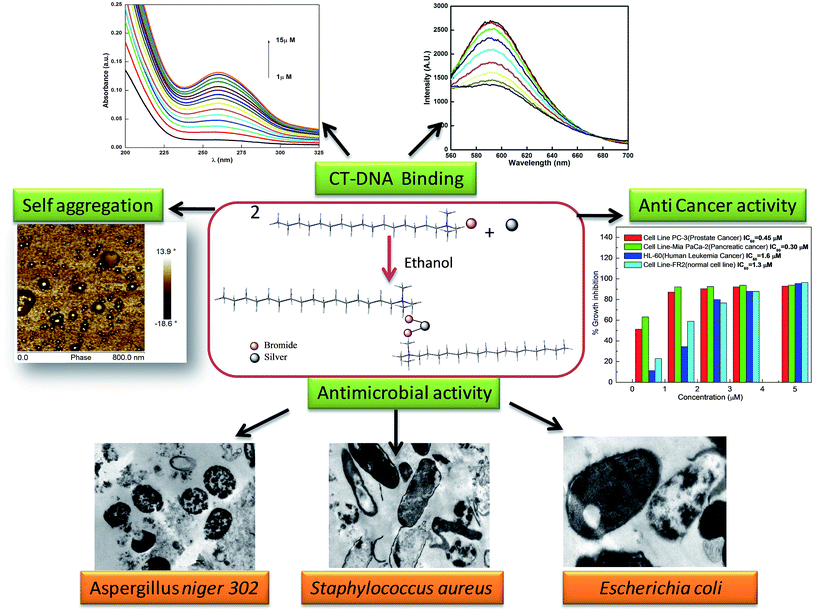 |
| Scheme 1 The pictorial representation of the formation of the silver metallosurfactant and its potential applications. | |
Acknowledgements
GK is thankful to DST for Inspire Faculty award (IFA-12-CH-41) and DST PURSE grant II. Authors from GJUS&T, Hisar thanks Nano Mission, DST, DBT and UGC for infrastructural and research facilities and IIT Ropar for AFM analysis.
References
- T. Dudev and C. Lim, Competition among metal ions for protein binding sites: determinants of metal ion selectivity in proteins, Chem. Rev., 2014, 114, 538–556 CrossRef CAS PubMed.
- I. Warad, A. F. Eftaiha, M. A. Al-Nuri, A. I. Husein, M. Assal, A. A. Obaid, N. Al-Zaqri, T. B. Hadda and B. Hammouti, Metal ions as antitumor complexes-review, J. Mater. Environ. Sci., 2013, 4, 542–557 CAS.
- A. M. Florea and D. Busselberg, Cisplatin as an anti-tumor drug: cellular mechanisms of activity, drug resistance and induced side effects, Cancers, 2011, 3, 1351–1371 CrossRef CAS PubMed.
- C. Xu, B. Wang and S. Sun, Dumbbell-like Au–Fe3O4 nanoparticles for target-specific ciplatin delivery, J. Am. Chem. Soc., 2009, 131, 4216–4217 CrossRef CAS PubMed.
- K. Cheng, S. Peng, C. Xu and S. Sun, Porous hollow Fe3O4 nanoparticles for targeted delivery and controlled release of cisplatin, J. Am. Chem. Soc., 2009, 131, 10637–10644 CrossRef CAS PubMed.
- S. Ramachandran, A. P. Quist, S. Kumar and R. Lal, Cisplatin nanoliposomes for cancer therapy: AFM and fluorescence imaging of cisplatin encapsulation, stability, cellular uptake, and toxicity, Langmuir, 2006, 22, 8156–8162 CrossRef CAS PubMed.
- Y. Zhang, X. J. Wang, M. Guo and H. S. Yan, Cisplatin-loaded Polymer/Magnetite Composite Nanoparticles as Multifunctional Therapeutic Nanomedicine, Chin. J. Polym. Sci., 2014, 32, 1329–1337 CrossRef CAS.
- P. Xu, E. A. VanKirk, W. J. Murdoch, Y. Zhan, D. D. Isaak, M. Radosz and Y. Shen, Anticancer Efficacies of Cisplatin-Releasing pH-Responsive Nanoparticles, Biomacromolecules, 2006, 7, 829–835 CrossRef CAS PubMed.
- M. Oishi, H. Hayashi, I. D. Michihiro and Y. Nagasaki, Endosomal release and intracellular delivery of anticancer drugs using pH-sensitive PEGylated nanogels, J. Mater. Chem., 2007, 17, 3720–3725 RSC.
- I. A. Fallis, P. C. Griffiths, P. M. Griffiths, D. E. Hibbs, M. B. Hursthouse and A. L. Winnington, Solid state and solution behaviour of novel transition metal containing surfactants, Chem. Commun., 1998, 665–666 RSC.
- T. Owen and A. Butler, Metallosurfactants of bioinorganic interest: coordination-induced self assembly, Coord. Chem. Rev., 2011, 255, 678–687 CrossRef CAS PubMed.
- C. R. van den Brom, M. Wagner, V. Enkelmann, K. Landfester and C. K. Weiss, Alkylsulfides of Ag(I) and Au(I) as metallosurfactants, Langmuir, 2010, 20, 15794–15801 CrossRef PubMed.
- S. Veeralakshmi, S. Nehru, G. Sabapathi, S. Arunachalam, P. Venuvanalingam, P. Kumar, C. Anusha and V. Ravikumar, Single and double chain surfactant–cobalt(III) complexes: the impact of hydrophobicity on the interaction with calf thymus DNA, and their biological activities, RSC Adv., 2015, 5, 31746–31758 RSC.
- R. F. P. Pereira, A. J. M. Valente, H. D. Burrows, V. D. Z. Bermudez, R. A. Carvalho and R. A. E. Castro, Structural characterization of solid trivalent metal dodecyl sulfates: from aqueous solution to lamellar superstructures, RSC Adv., 2013, 3, 1420–1433 RSC.
- A. Riyasdeen, R. Senthilkumar, V. S. Periasamy, P. Preethy, S. Srinag, M. Zeeshan, H. Krishnamurthy, S. Arunachalam and M. A. Akbarsha, Antiproliferative and apoptosis induction studies of a metallosurfactant in human breast cancer cell MCF-7, RSC Adv., 2014, 4, 49953–49959 RSC.
- A. I. Adawy and M. M. Khowdiary, Structure and Biological Behaviors of Some Metallo Cationic Surfactants, J. Surf. Deter., 2013, 16, 709–715 CrossRef CAS.
- K. Osada, H. Cabral, Y. Mochida, S. Lee, K. Nagata, T. Matsuura, M. Yamamoto, Y. Anraku, A. Kishimura, N. Nishiyama and K. Kataoka, Bioactive polymeric metallosomes self-assembled through block copolymer–metal complexation, J. Am. Chem. Soc., 2012, 134, 13172–13175 CrossRef CAS PubMed.
- C. Zhang, Y. Zhu, C. Zhou, W. Yuan and J. Du, Antibacterial vesicles by direct dissolution of a block copolymer in water, Polym. Chem., 2013, 4, 255–259 RSC.
- I. Sondi and B. Salopek-Sondi, Silver nanoparticles as antimicrobial agent: a case study on E. coli a model for Gram-negative bacteria, J. Colloid Interface Sci., 2004, 275, 177–182 CrossRef CAS PubMed.
- Z. Liu, Y. Wang, Y. Zu, Y. Fu, N. Li, N. Guo, R. Liu and Y. Zhang, Synthesis of polyethylenimine (PEI) functionalized silver nanoparticles by a hydrothermal method and their antibacterial activity study, Mater. Sci. Eng., C, 2014, 42, 31–37 CrossRef CAS PubMed.
- Z. M. Sui, X. Chen, L. Y. Wang, L. M. Xu, W. C. Zhuang, Y. C. Chai and C. J. Yang, Capping effect of CTAB on positively charged Ag nanoparticles, Phys. E, 2006, 33, 308–314 CrossRef CAS.
- N. Ren, Y. Tang, Y. J. Wang, A. G. Dong and W. L. Yangy, Fabrication of the sponge-like layered silver(I)-alkylamine complexes and their in situ reduction, Chem. Lett., 2002, 372–373 CrossRef CAS.
- Z. Sui, X. Chen, L. Wang, Y. Chai, C. Yang and J. Zhao, An improved approach for synthesis of positively charged silver nanoparticles, Chem. Lett., 2005, 34, 100–101 CrossRef CAS.
- X. H. Liu, X. H. Luo, S. X. Luc, J. C. Zhang and W. L. Cao, A novel cetyltrimethyl ammonium silver bromide complex and silver bromide nanoparticles obtained by the surfactant counterion, J. Colloid Interface Sci., 2007, 307, 94–100 CrossRef CAS PubMed.
- G. Kaur, S. K. Mehta, S. Kumar, G. Bhanjana and N. Dilbaghi, Coencapsulation of hydrophobic and hydrophilic anti-TB drugs in synergistic Brij 96 microemulsions: a biophysical characterization, J. Pharm. Sci., 2015, 104, 2203–2212 CrossRef CAS PubMed.
- S. Kumar, N. Kumar, G. Bhanjana, R. Thakur and N. Dilbaghi, Enhanced Antimicrobial Activity of Antibiotics Mixed with Metal Nanoparticles, AIP Conf. Proc., 2011, 1393, 203–204 CrossRef CAS.
- S. Bhushan, J. Singh, J. M. Rao, A. K. Saxena and G. N. Qazi, A novel lignin composition from Cedrus deodara induces apoptosis and early nitric oxide generation in human leukemia Molt-4 and HL-60 cells, Nitric Oxide, 2006, 14, 72–88 Search PubMed.
- M. M. Husein, E. Rodil and J. H. Vera, A novel approach for the preparation of AgBr nanoparticles from their bulk solid precursor using CTAB microemulsions, Langmuir, 2006, 22, 2264–2272 CrossRef CAS PubMed.
- D. N. Waters and B. Basak, Vibrational spectra of dihalogeno–anions of copper(I) and silver(I) in solution in tri-n-butyl phosphate, J. Chem. Soc. A, 1971, 2733–2735 RSC.
- R. Minkwitz, D. Bernstein and W. Sawodny, Synthesis of Tetrachloroammonium Hexafluoroarsenate, NCl4+AsF6−, Angew. Chem., Int. Ed. Engl., 1990, 29, 181–183 CrossRef.
- M. Chakraborty, F. W. Hsiao, B. Naskar, C. H. Chang and A. K. Panda, Surfactant-assisted synthesis and characterization of stable silver bromide nanoparticles in aqueous media, Langmuir, 2012, 28, 7282–7290 CrossRef CAS PubMed.
- G. Kaur, G. Karir and S. K. Mehta, Studies on thermogravimetric analysis and solvophobic interactions of micellization of Pd(II) complex in non aqueous solvents, Colloids Surf., A, 2013, 434, 25–34 CrossRef CAS.
- E. Fuguet, C. Ràfols, M. Rosés and E. Bosch, Critical micelle concentration of surfactants in aqueous buffered and unbuffered systems, Anal. Chim. Acta, 2005, 548, 95–100 CrossRef CAS.
- E. Dutkiewicz and A. Jakubowska, Effect of electrolytes on the physicochemical behavior of sodium dodecyl sulphate micelles, Colloid Polym. Sci., 2002, 280, 1009–1014 CAS.
- N. Vlachy, B. Jagoda-Cwiklik, R. Vacha, D. Touraud, P. Jungwirth and W. Kunz, Hofmeister series and specific interactions of charged headgroups with aqueous ions, Adv. Colloid Interface Sci., 2009, 146, 42–47 CrossRef CAS PubMed.
- W. Kunz, Specific ion effects in colloidal and biological systems, Curr. Opin. Colloid Interface Sci., 2010, 15, 34–39 CrossRef CAS.
- A. M. Badawi, N. I. Zakhary, S. M. I. Morsy, G. M. Sabry, M. R. Mohamed and A. M. Mousa, Copper(II)–surfactant complex and its nano analog as potential antitumor agents, J. Dispersion Sci. Technol., 2009, 30, 1303–1309 CrossRef CAS.
- G. R. Chaudhary, P. Singh, G. Kaur, S. K. Mehta, S. Kumar and N. Dilbaghi, Multifaceted Approach for the Fabrication of Metallomicelles and Metallic Nanoparticles Using Solvophobic Bisdodecylaminepalladium(II) Chloride as Precursor, Inorg. Chem., 2015, 54, 9002–9012 CrossRef CAS PubMed.
- A. Modaressi, H. Sifaoui, B. Grzesiak, R. Solimando, U. Domanskac and M. Rogalski, CTAB aggregation in aqueous solutions of ammonium based ionic liquids; conductimetric studies, Colloids Surf., A, 2007, 296, 104–108 CrossRef CAS.
- M. M. Islam, M. R. Rahman and M. N. Islam, Micellization behavior and thermodynamic properties of n-alkyl trimethylammonium bromide surfactants in aqueous media at different temperatures, Int. J. Sci. Eng. Res., 2015, 6, 1508–1516 Search PubMed.
- A. Jakubowska, Interactions of univalent counterions with headgroups of monomers and dimers of an anionic surfactant, Langmuir, 2015, 31, 3293–3300 CrossRef CAS PubMed.
- D. L. Boger, B. E. Fink, S. R. Brunette, W. C. Tse and M. P. Hedrick, A Simple, High-Resolution Method for Establishing DNA Binding Affinity and Sequence Selectivity, J. Am. Chem. Soc., 2001, 123, 5878–5891 CrossRef CAS PubMed.
- H. Y. Wang, X. W. Hua, F.-G. Wu, B. Li, P. Liu, N. Gu, Z. Wang and Z. Chen, Synthesis of ultrastable copper sulfide nanoclusters via trapping the reaction intermediate: potential anticancer and antibacterial applications, ACS Appl. Mater. Interfaces, 2015, 7, 7082–7092 CAS.
- R. Hu, K.-T. Yong, I. Roy, H. Ding, S. He and P. N. Prasad, Metallic nanostructures as localized plasmon resonance enhanced scattering probes for multiplex dark field targeted imaging of cancer cells, J. Phys. Chem. C, 2009, 113, 2676–2684 CAS.
- M. J. Firdhouse and P. Lalitha, Biosynthesis of silver nanoparticles using the extract of Alternanthera sessilis—antiproliferative effect against prostate cancer cells, Cancer Nanotechnol., 2013, 4, 137–143 CrossRef PubMed.
- C. Parnsamut and S. Brimson, Effects of silver nanoparticles and gold nanoparticles on IL-2, IL-6, and TNF-α production via MAPK pathway in leukemic cell lines, Genet. Mol. Res., 2015, 14, 3650–3668 CrossRef CAS PubMed.
- G. M. Sulaiman, W. H. Mohammed, T. R. Marzoog, A. A. A. Al-Amiery, A. A. H. Kadhum and A. B. Mohamad, Green synthesis, antimicrobial and cytotoxic effects of silver nanoparticles using Eucalyptus chapmaniana leaves extract, Asian Pac. J. Trop. Biomed., 2013, 3, 58–63 CrossRef CAS PubMed.
- A. Barbasz, M. Oćwieja and J. Barbasz, Cytotoxic activity of highly purified silver nanoparticles sol against cells of human immune system, Appl. Biochem. Biotechnol., 2015, 176, 817–834 CrossRef CAS PubMed.
- G. M. Sulaiman, E. H. Ali, I. I. Jabbar and A. H. Saleem, Synthesis, characterization, antibacterial and cytotoxic effects of silver nanoparticles, Dig. J. Nanomater. Biostruct., 2014, 9, 787–796 Search PubMed.
- Q. L. Feng, J. Wu, G. Q. Chen, F. Z. Cui, T. N. Kim and J. O. Kim, A mechanistic study of the antibacterial effect of silver ions on Escherichia coli and Staphylococcus aureus, J. Biomed. Mater. Res., 2000, 52, 662–668 CrossRef CAS PubMed.
- J. Tang, Q. Chen, L. G. Xu, S. Zhang, L. Z. Feng, L. Cheng, H. Xu, Z. Liu and R. Peng, Graphene Oxide-Silver Nanocomposite as a Highly Effective Antibacterial Agent with Species-Specific Mechanisms, ACS Appl. Mater. Interfaces, 2013, 5, 3867–3874 CAS.
- M. Nocchetti, A. Donnadio, V. Ambrogi, P. Andreani, M. Bastianini, D. Pietrellac and L. Latterinia, Ag/AgCl nanoparticle decorated layered double hydroxides: synthesis, characterization and antimicrobial properties, J. Mater. Chem. B, 2013, 1, 2383–2393 RSC.
- Y. Xia, C. Cheng, R. Wang, C. Nie, J. Denga and C. Zhao, Ag-nanogel blended polymeric membranes with antifouling, hemocompatible and bactericidal capabilities, J. Mater. Chem. B, 2015, 3, 9295–9304 RSC.
Footnote |
† Electronic supplementary information (ESI) available. See DOI: 10.1039/c6ra09677h |
|
This journal is © The Royal Society of Chemistry 2016 |