DOI:
10.1039/C6RA08641A
(Review Article)
RSC Adv., 2016,
6, 67449-67480
Controlled biodegradation of polymers using nanoparticles and its application
Received
4th April 2016
, Accepted 20th June 2016
First published on 28th June 2016
Abstract
The disposal of non-degradable plastics has increased exponentially as a result of poor recycling efficacy. The development of biodegradable plastics has become indispensable over the last two decades because of their origin from renewable resources. The potential interest in biodegradable plastics comes from the eco-friendly obliteration via microbial action which transforms the plastics into carbon dioxide and water resulting in a pollution-free natural system. Even though the lucrative interest lies in multiple areas, some of the properties such as brittleness, and poor thermal, mechanical and low gas barrier properties restrict their practical uses. The incorporation of nanoparticles in the polymer matrix or the fabrication of nanocomposites overcomes the shortcomings of the biodegradable polymers. To improve the aforementioned properties further, several approaches have been utilized, especially the incorporation of nanoparticles in the polymer matrix to prepare nanocomposites. One of the great advantages of nanoparticles is the ability to tune the rate of biodegradation (it is possible to both increase and decrease the rate as compared to that of the pure polymer) depending upon the need. Thus, chemical, physical and biological properties of the biodegradable polymers can be modified and controlled for sustainable applications in medicine and other areas. This review considers the major concerns of biodegradable polyesters and their nanocomposites, great details about the mechanism of their biodegradation, factors influencing their biodegradation and their applications in biomedical and packaging industries.
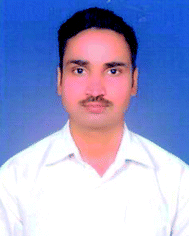 SunilKumar | Sunil Kumar is currently working as Research Associate in the School of Materials Science and Technology, Indian Institute of Technology (Banaras Hindu University), Varanasi. He received his Master's degree in Biotechnology from University of Calicut and Ph. D. from Motilal Nehru National Institute of Technology, Allahabad. He has awarded with Research Associate (RA) fellowship from Council of Scientific and Industrial Research (CSIR), New Delhi (India). He has published eight research articles in high impact peer reviewed journals with total citation of 60. His main research is focused on controlled degradation of polymer by enzyme in presence of nanoparticles. |
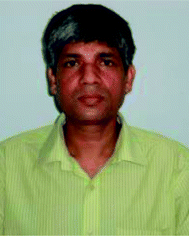 PralayMaiti | Pralay Maiti is currently a professor in the School of Materials Science and Technology, Indian Institute of Technology (Banaras Hindu University), Varanasi. He did his Master from IIT Kharagpur in Chemistry and Ph. D from Indian Association for the Cultivation of Science, Kolkata. He served as COE researcher, postdoctoral fellow and visiting scientist at Hiroshima University, Toyota Technological Institute, Japan and Cornell University, USA for nearly seven years. He is actively working on polymer hybrid systems, biomaterials, fuel cell membrane, controlled drug delivery and polymer based energy materials. He has published hundred papers in high impact peer reviewed journals with total citation of ∼5000. Prof. Maiti's work has been recognized with “Prof. M. Santappa Silver jubilee award” by the Society of Polymer Science, “Young Scientist award” by the Asian Polymer Association and “National Award for Technology Innovation” by the Ministry of Chemicals and Fertilizers, Government of India. |
1. Introduction
Over the past few decades, non-biodegradable plastics such as polyethylene (PE), polypropylene (PP), polystyrene (PS), poly(vinyl chloride) (PVC) and poly(ethylene terephthalate) (PET) have been widely used due to their excellent performance. Most of these plastics persist for many years after the expiration of their service-time, producing huge amounts of plastic waste. The total global amount of commodity plastic production increased dramatically from 1.5 million tons in 1950 to 245 million tons in 2008 with an annual growth rate of 9%.1 It is reported that 435
000, 214
000 and 1
686
000 tons of PVC waste was produced in the USA, Japan and China, respectively.2 Approximately 140 million tons of different kinds of petroleum-derived synthetic plastics are produced every year and a considerable amount of these plastics are introduced into the environment as industrial waste.3 A report on the production and recycling of plastics has been documented by the US Environmental Protection Agency (EPA) and European Bioplastics Association as shown in Fig. 1A.4 However, these conventional plastics are resistant to microbial attack and end up in landfill burial sites for thousands of years, causing a shortage of landfill space and a negative impact on wildlife through ingestion and entrapment.5,6 The shortage of landfill space enforces the adoption of non-scientific processes for incineration that cause air pollution resulting in one of the prominent causes of climate change. Oceans are recognized as a major sink for the deposition of plastics and this results in microscopic granular or fiber-like fragments known as ‘microplastics’ progressively accumulating in the ocean bed. The uptake of microplastics by marine organisms leads to bio-magnification on a higher level through the food chain. Unfortunately, this affects the endocrine system, impairs reproduction, or causes cancer.7 For instance, polystyrene is a common material used for the packaging of food items and is made up of styrene which is considered as a neurotoxin (it impairs the central and peripheral nervous systems) and a carcinogen (it causes cancer and chromosomal aberrations).8 Vinyl chloride (VC) is an industrial solvent primarily used to manufacture poly(vinyl chloride). The toxicity of vinyl chloride has been evaluated in aquatic organisms Daphnia magna at the gene, cellular and life-history levels. The exposure of 0.1, 1, and 10 μg L−1 of VC significantly increases the activity of juvenile hormone esterase in D. magna causing premature reproduction and metamorphosis.9 Phthalates are well-known plasticizers considered as endocrine disrupting compounds causing oxidative stress and alterations in cytokine expression leading to allergies and asthma.10 Accumulation of all these wastes in the environment is being treated as a threat to the balance of ecological hierarchy. Therefore, the inclination towards the production of biodegradable polymers has increased to overcome these environmental problems due to the disposal of non-degradable plastics, especially to circumvent environmental pollution.
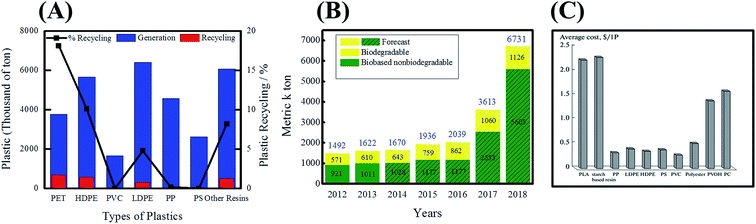 |
| Fig. 1 (A) Plastic generation and recycling;4 (B) global production of bioplastics;11 (C) cost comparison between biodegradable and traditional polymers (this figure has been reproduced from ref. 25 with permission from Springer). | |
1.1 General introduction and current status of biodegradable polymers
Biodegradable polymers are the sustainable alternative to non-degradable synthetic plastics. Globally, bioplastics represent less than 1% of the 181 million metric tons of synthetic plastics that the world produces every year. However, the bioplastic market is growing by 20–30% each year, but not enough to meet the global need.3 According to the European Bioplastics Association, the global production of bioplastics amounted to 1.6 million tonnes in 2013 and is expected to increase to approximately 6.7 million tonnes by 2018 (Fig. 1B).11 Therefore, biodegradable plastics such as poly(butylene succinate) (PBS), polyhydroxyalkanoates (PHAs), polyhydroxyvalerate (PHV), poly(butylene succinate-co-adipate) (PBSA), poly(ε-caprolactone) (PCL), and poly(lactic acid) (PLA) have been synthesized commercially to replace the synthetic plastics. Biodegradable plastics, especially polyesters, have received much attention for their potentially hydrolysable ester bonds that can be fragmented to their respective monomer or oligomer units under bioactive environments. Thus, biodegradable plastics could be used in the same manner as conventional plastics under ordinary conditions and can be degraded in the presence of microorganisms after disposal. The biodegradability of PCL and PLA samples in compost under controlled conditions was measured using a Microbial Oxidative Degradation Analyzer (MODA) according to ISO 14855-2 in which nine tests for the biodegradation of plastics were standardized according to TC61/SC5/WG22.12 Amongst the Asian countries, Japan is the leader in biotechnology providing the information on biomass-based plastics and biodegradable plastics. The Japan Bioplastic Association (JBPA)13 classified bioplastics into two groups: (i) biodegradable plastics, referring to biodegradable synthetic polymers with an average molecular weight of at least 1000 Da. This includes chemically modified starch and poly(amino acid) based biodegradable high polymers. Green PLA is the first biodegradable plastic which is microbiologically mineralized to carbon dioxide and water in the natural environment; (ii) biomass-based plastics which are produced using biomass as the raw material. They have been produced either chemically or biochemically using renewable organic materials. Biomass-based plastics are further characterized into two groups: (a) totally biomass based plastics and (b) partially biomass based plastics. The JBPA has aimed to replace 20% of petrochemical plastics with biomass based plastics. Comparable ideas on bioplastic production and degradation have also been reported by the European Bioplastics Association i.e. bioplastics are derived either from renewable resources (bio-based) or from the plastics which are biodegradable and compostable. The European Bioplastics Association proclaimed that one hundred percent of bio-based plastics might be non-biodegradable but 100 percent of fossil-based plastics may be biodegradable. Similarly, the differential substrate hydrolysis of various polyesters like PLA, PHB, PCL and their copolymers was studied by Lim et al.14 and they suggested that biodegradation does not depend on the starting material of a particular polymer, but is rather related to its chemical structure and composition.
An overview of aliphatic polyesters having similar characteristics and being comparable to conventional plastics is summarized in brief. PLA and PHAs are considered as natural polymers while PCL and PBS are petroleum based synthetic and biodegradable polymers. PLA is a biodegradable and biocompatible polyester that is produced through microbial fermentation using sustainable resources like corn. PLA has received the most attention because of its low cost and improved properties. It can also be synthesized either by condensation of lactic acid or by ring opening polymerization of lactide in the presence of a catalyst. This polymer exists in three stereoisomeric forms: (1) poly(L-lactide), (2) poly(D-lactide), and (3) poly(DL-lactide) depending on the proportion of enantiomers. Polyhydroxyalkanoates (PHAs) are bacterial polymers, produced intracellularly as a by-product during biochemical cycles and stored as carbon and energy reserves in many bacteria. PHAs are composed of diverse monomer structures with over 120 variations depending on the bacterial species and growth conditions. These bacterial polymers include poly(3-hydroxybutyrate), poly(3-hydroxybutyrate-co-3-hydroxyvalerate) and derivatives of its copolymer. Poly(3-hydroxybutyrate-co-3-hydroxyvalerate) is marketed under the name of Biopol™ and has a melting temperature between 120 and 180 °C depending on the composition. PCL is a synthetic biodegradable polyester prepared through the ring-opening polymerization of ε-caprolactone and has a low melting point (60–65 °C). The degradation rate of PCL is dependent on its molecular weight and degree of crystallinity. In the family of synthetic biodegradable polyesters, poly(butylene succinate) (PBS) is another promising biopolymer owing to its desirable properties including excellent thermal and chemical resistance, and better toughness than other biodegradable polymers. It is chemically synthesized by polycondensation of aliphatic dicarboxylic acids (adipic and succinic acid) and glycols (ethylene glycol and/or 1,4-butanediol).
1.2 Life cycle assessment of polymeric materials
The environmental impact of polymeric waste materials may be evaluated in terms of a life cycle assessment (LCA) starting from the raw materials, the manufacturing process, selling them in a store, using them in the workplace or at home and disposing of them or recycling them into new products.15,16 The parameters considered in the LCA are global warming, acidification, eutrophication, photochemical oxidant formation, solid waste, emission of dioxin, ozone layer depletion, abiotic depletion and cumulative non-renewable energy demand.2,17 The LCA methodology has been standardized under the ISO-14040 series and it distinguishes four phases: goal and scope definition, inventory analysis, impact assessment, and interpretation.1 The main goal of a waste policy should be zero waste discharge accompanied by minimum waste production (reduction), re-use of products, recycling of materials, and recovery of energy. In 2008, Kim and Dale18 conducted an LCA evaluation of corn grain derived PHB which offers environmental advantages over petroleum derived polymers in terms of non-renewable energy consumption and greenhouse gas emission. The custom use of PHB reduces greenhouse gas emissions as compared to petroleum-derived polymers. More sustainable practices in corn cultivation could reduce the environmental impacts of using PHB by up to 72%. Life cycle analyses demonstrate that bioplastics can significantly reduce CO2 emissions as compared to conventional plastics.
1.3 Advantages and challenges of biodegradable polymers
The most promising advantage of biodegradable polymers is the ability to sustain a number of environmental factors during their use while they have to be biodegradable in the presence of bioactive agents under disposal conditions. Biodegradable polymers have important roles at present due to their progressive applications with respect to environmental safety, biomedical implementation, packaging and agricultural uses. Poly(lactic acid) (PLA) is one of the most commercially accessible and frequently used polyesters because of its highly hydrolysable ester bonds and low production cost.19 Rasal et al.20 reported that PLA requires 25–55% less energy for production as compared to petroleum-based polymers which might be improved further in the near future. Therefore, the interest in the fermentative production of lactic acid has increased to use renewable resources instead of petrochemicals. The production of optically active compounds, either pure L-(+)- or D-(−)-lactic acid, could be controlled by tuning the physicochemical parameters, yielding stereo-specific copolymers. Thus, the biotechnological production of lactic acid offers several advantages compared to chemical syntheses.21 Since biodegradable polyesters are compostable and derived from sustainable sources, they can be thought of as promising materials to minimize the solid waste disposal problem. Their low immunogenic characteristics, being biological in origin, have highlighted these polyesters as ideal materials for food packaging and for other consumer products.22
Although biodegradable polymers show profitable uses in multiple areas, some of their properties such as brittleness, low-heat distortion temperature, low-melt viscosity, poor thermal and mechanical resistance, restricted gas barrier properties and slow degradation rate limit their use in industrial sectors.23,24 The above drawbacks can be overcome by enhancing the thermo-mechanical properties through copolymerization, blending and using a filled system. The preparation of nanocomposites using nanometer scale particles confers an effective way to alter the structural and functional properties of biopolymers. Over the last few decades, the incorporation of nanoparticles into polymers has affected multiple properties of the polymers. For instance, 1–5 wt% layered silicate nanocomposites exhibit distinctly improved degradation behavior, thermal and mechanical properties, barrier and flame retardance features in comparison to the unfilled matrix.23 Another major limitation of biodegradable polymers is cost effective production (Fig. 1C)25 and recovery of biopolymers through a fermentation process. It is limited to the cell’s ability to utilize inexpensive carbon sources especially agricultural byproducts. The sluggish growth rate of microorganisms in low cost media (agricultural byproducts) lowers the production of polymers which subsequently increases the downstream processing costs.1,21,25 In this regard, isolation and screening of potent and efficient microorganisms (especially fungal and bacterial strains) from the natural environment are necessary in order to utilize low cost feed stock. Raw materials account for 30–40% of the total cost of PHB.26 Therefore, research has been focused on cultivation strategies to design growth media using inexpensive carbon substrates in order to reduce the production cost and procure maximum productivity under optimized conditions. Moreover, the physicochemical parameters of fermentation are widely dependent on the pH, temperature and incubation period of the medium which exerts an influence on the metabolism of the microbes that accelerate the production or accumulation of the biopolymer. Bora27 studied Bacillus megaterium bacteria in the production of PHB and it was found to vary from 24 to 48% (w/w) of the dry cell weight. Box Bohn design was used in studying the interactive effect of four variables on cell growth and PHB production. The integrative approach of statistical methods and response surface methodology (RSM) were employed for the optimization of the fermenting media that helped in understanding the interactions between various physicochemical parameters involved in PHB production. This study has not only enhanced the production of PHB but also reduces the number of experiments in spite of multiple variables and exposes its possible application for scale up studies. However, with recent advances in recombinant technology and genetic engineering, the PHA genes are to be cloned and expressed in different recombinant bacteria to improve PHA production. Therefore, extensive research is required to understand the genetic profile of microorganisms and their biochemical pathways involving the production of polymers. All the processes and technologies help to overcome the limitations related to the production of biopolymers.
1.4 Biodegradable polymers, bioplastics, biomass derived plastics and biodegradation
Biodegradation is a biochemical phenomenon involving the hydrolytic cleavage of chemical bonds under certain environmental conditions by microorganisms or their secreted products like enzymes and surfactants following a definite path. Depending on the mode of degradation, it may be aerobic or anaerobic.28 Biodegradation is distinguished from common degradation, where disintegration of the polymer into small pieces takes place under harsh conditions in the absence of microorganisms as mentioned earlier e.g. photo degradation, thermal degradation, weathering and chemical degradation etc. The term “biodegradable” refers to the materials that can be decomposed or mineralized into ultimate end products of carbon dioxide and water on being exposed to certain microbial environments. The polymers being degraded in this manner are known as biodegradable polymers and this strongly depends upon the chemical structure of the polymers instead of their raw materials.29
Bio-derived plastics or bio-based plastics are polymers obtained entirely or partially from renewable carbon resources (especially biomass) fulfilling the concept of “carbon neutrality” to reduce the emission of greenhouse gases (CO2) and the dependency on fossil resources. Accordingly, when bio-based plastics are burned after their final use, the generated carbon dioxide is again converted into biomass by photosynthesis i.e. they are carbon neutral. It does not matter whether bio-based plastics are biodegradable or not. Therefore, the use of bio- or renewable carbon as opposed to petro- or fossil carbon for the manufacture of bio-based plastic reduces the carbon footprint.30,31 According to European Bioplastics Association, 100% of bio-based plastics may be non-biodegradable and 100% of fossil based plastics can be biodegradable. For instance, bio-based poly(hydroxyl alkanoates) are biodegradable while polyethylene derived from the chemical dehydration of ethanol is not.32 Moreover, materials or products are acknowledged to be biodegradable while further information about the time frame, level of biodegradation, and required surrounding conditions must be provided. Therefore, a plastic material is defined as a bioplastic if it is either bio-based, biodegradable, or features both properties. Bioplastic materials mainly consist of three groups:
(i) Bioplastics directly extracted from biomass, such as polysaccharides (chitosan, starch, and cellulose), proteins (gluten and soy) and lipids. Starch can be made thermoplastic through destructurization in the presence of specific amounts of plasticisers (water and/or poly-alcohols).33 Others are soy-based bioplastics,34 pea protein-based bioplastics35 and cellulose-based bioplastics.36
(ii) Bioplastics obtained from biomass derived monomers require subsequent chemical processing for their conversion to bioplastics e.g. the monomeric intermediate of lactic acid, obtained from fermentation feed stock of corn starch, requires a chemical transformation for its conversion to poly(lactic acid) (PLA).37
(iii) Microbial bioplastics such as poly(hydroxyl alkanoates) (PHA), PHB, poly(butylene succinate) (PBS) and their derivatives produced by natural or genetically modified micro-organisms which accumulate intracellularly in the form of storage granules. Bioplastics differ in their monomer composition, macromolecular structure and physical properties depending on their microbial origin, survival rate, stress conditions, type of microorganisms, media ingredients, fermentation conditions, modes of fermentation (batch, fed-batch, continuous) and downstream processing.38 A neutral pH showed higher PHA accumulation (pH, 7; 56%), followed by basic (pH, 8; 46%) and acidic (pH, 6; 39%) conditions.39 Currently, the main limitations for the bulk production of bioplastics are their high production and recovery costs.38
2. Types of polymer degradation
Degradation is an irreversible phenomenon where the decrease of molecular weight of the polymer takes place due to photo-oxidation, chemical erosion, or thermal and biological agents leading to mechanical failure (Table 1). As a consequence, the object becomes brittle and the half-life of the material is reduced.40 The process of degradation occurs mainly through the scission of the backbone/side chains of the polymer. In environmental conditions, the degradation of polymers is prompted by the coexistence of biotic and abiotic factors. Biotic systems include microbes, enzymes or a combination of both which perform biological activity for their growth employing polymers as the carbon source. On the other hand, abiotic factors such as pH, temperature, humidity, salinity, and the presence or absence of oxygen stimulate the benign growth of the microbial population and also influence their metabolic activity. Therefore, bioreactors are designed in such a manner that mimics the environmental conditions during degradation. The biodegradability of different plastics under aerobic conditions significantly depends on the type of biodegradable plastic.41 Apart from the biological processes, chemical and physical actions such as thermal activation, oxidation, hydrolysis, photolysis or radiolysis cause the degradation of polymeric materials.
Table 1 Brief overview of the different types of degradation of polymersa
Type of degradation |
Causative agents |
Degradation: degradation is an irreversible phenomenon where the deterioration of the properties of the polymer takes place. |
Photo-degradation caused by electromagnetic radiation |
• High energy radiation: X-ray, α, β, γ rays |
• Photo-oxidation: O2 + UV and visible light |
Thermal degradation initiated via heat and temperature |
• Thermal oxidation: O2 + temperature |
• Thermal hydrolysis: H2O + temperature |
Chemical degradation |
• Hydrolytic degradation: H2O |
• Etching: acid, alkali, salt or reactive gases |
Weathering |
• Physical wear: cracking and erosion |
• Abrasive force: stress and fatigue |
Biodegradation mediated by biological agents |
• Enzymatic degradation: lipase, proteinase |
• Microbial degradation: bacteria or fungi |
2.1 Weathering
The aging behavior of polyesters is often affected by abiotic factors such as temperature, pH, soil texture, oxygen, salinity, radiation and rainfall under environmental conditions. The structural changes during the aging of polyesters are accredited to the series of chain scission and crosslinking reactions. Both reactions lead to the loss of the physical properties of the aged polymers. Thus, aging properties are important factors to decide the expiry date of polymeric products.42 A poly(3-hydroxybutyrate-co-3-hydroxyvalerate)/poly(butylene adipate-co-terephthalate) blend with a halloysite nanoclay film was subjected to photooxidative weathering in a climatic chamber under UV exposure and showed a greater colour change and a reduction in the transparency of the nanocomposite film as compared to the unfilled polymer due to the changes in the molecular structure (hydrolysis of the ester linkage, multiple chain scissions and crosslinking as observed through FTIR analyses).43 Photo aging is considered as an oxidative process involving chain scission and recombination. The two mechanisms compete with each other and one of them is usually predominant and characterizes the overall behavior of the polymer under weathering.44 Fang et al.42 prepared bio-based anti-aging polyester elastomer (BEE) nanocomposites using carbon blend (CB) to investigate their aging behavior and mechanism through tensile testing and glass transition temperatures at different temperatures showing initial crosslinking phenomena followed by the domination of chain scission at the later stage. Similarly, the formation of insoluble polymeric gels (crosslinking) due to photooxidation has been shown by Stloukal et al.45 and this phenomenon is pronounced for copolyesters with higher aromatic content. On the other hand, PLA exhibits chain scission predominantly over crosslinking.45 The weathering of sugar palm fibres (SPF) and sugar palm starch biocomposites (SPS) in a xenon arc chamber after 72 h (ASTM G155) show a loss of tensile strength to the tune of 78% and 53.7%, respectively.46 Effects of climatic conditions and soil factors on the degradation of polymers were also analyzed through a weight loss method by placing poly(3-hydroxybutyrate-co-valerate) (PHBV), poly(ε-caprolactone) (PCL), poly(butylene succinate) (PBS), poly(butylene succinate and adipate) (PBSA), and polylactide (PLA) in soil at different sites showing the degradation order of PBSA = PHBV = PCL > PBS > PLA.47
2.2 Photo degradation
Light induced degradation of polymers is a physical phenomenon caused by the irradiation of high frequency electromagnetic radiation, especially ultraviolet and gamma rays. Absorption of photons causes chain scission in polymeric molecules leading to a reduction in the molar mass. A prerequisite for the initiation of any photochemical reaction is the presence of chromophoric groups within the molecules which absorb a quantum of light. The well-known singlet oxygen mechanism has been proposed to explain the photo oxidation of polymers and certain sensitizers/inducers are blended into the polymer matrix which induces the transfer of energy in a subatomic level of the polymer matrix to generate singlet oxygen (1O2) arising from the quenching of the excited triplet state.48 The blending of certain organic and inorganic molecules in the polymer is considered to facilitate free radicals in the presence of light leading to the formation of hydroperoxide (Fig. 2A).23,49
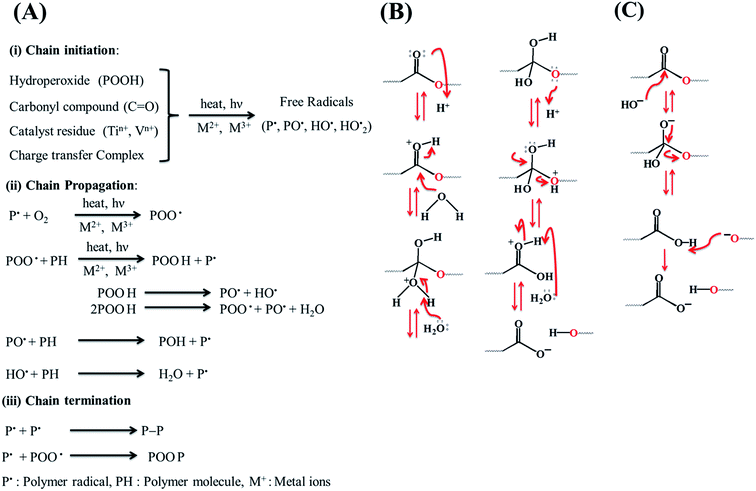 |
| Fig. 2 Degradation mechanisms: (A) general oxidation and photo-oxidation in polymers (this figure has been reproduced from ref. 48 with permission from Springer Open); (B) acidic hydrolysis; and (C) basic hydrolysis. | |
2.3 Thermal degradation
High temperatures increase the thermal energy of a system and, therefore, accelerate the process of degradation. Hyon et al.50 observed that PLA filaments were found to be intact for 6 months at 37 °C in phosphate buffer while they degraded by 50% after 30 h at 100 °C. Fukushima et al.19 investigated the effect of temperature on PLA degradation in phosphate buffer showing a considerably faster degradation at 58 °C than the control at 37 °C. The biodegradation of poly(L-lactide) (PLLA) was found to be increased at 52 °C in contrast to that at 37 °C under both anaerobic and aerobic conditions.51 The degree of mineralization is ∼60% after 40 days at 52 °C while a similar fraction of degradation occurs at 37 °C after 100 days of incubation in the anaerobic aquatic test. The degradation rate of PLLA increases considerably at temperatures close to the glass transition (55 °C).51 The activation energies for the degradation of poly(lactic-co-glycolic acid) (PLGA) at temperatures below and above Tg are found to be different52 even though the degradation of PLLA proceeds through the same mechanism.53 The thermal degradation of oligo-L-lactide at 200 °C under a nitrogen atmosphere involves a series of reactions like intra- and intermolecular ester exchange, cis elimination, radical and concerted non-radical reactions, and selective Sn-catalyzed depolymerization via the generation of various carbon- and oxygen-centred macro-radicals.54 However, molecular deterioration occurs at elevated temperatures due to overheating and the hydrolysis of polyesters while the mechanism of polyester degradation is much more complex, involving several processes.55
2.4 Hydrolysis
The process of hydrolytic degradation of a polymer matrix induces a change in the refractive index of the sample as a consequence of water absorption.56 Any factor which raises the hydrolytic tendency of a polymer matrix could be a principle accelerator for hydrolytic degradation. The presence of hydroxyl groups in the silicate layers or clays induces hydrolysis of the ester groups of the PLA matrix.57,58 The hydrolytic degradation of PLA in aqueous media proceeds faster in the amorphous region as compared to the crystalline zone leading to the formation of lactic acid oligomers which increase the carboxylic acid end group concentration in the medium. These carboxylic groups catalyze the hydrolytic degradation inherently. Therefore, the hydrolytic degradation of PLA has been reported to take place mainly in the bulk of the material, not on its surface. The decrease in the amount of lactic acid oligomer and the formation of degradation products was found to be dependent on the pH and dielectric constant of the medium. In acidic pH, hydrolysis was shown to proceed by chain-end scission whereas in alkaline media, lactoyl lactate splits.59 Depolymerization of PLA by a random alkaline attack on the carbon of the ester group takes place followed by the hydrolysis of the polymer.60 Thus, chain end degradation of PLA is explained by an intramolecular transesterification under alkaline conditions while the protonation of the hydroxyl end-group forms an intramolecular hydrogen bond in acidic environments. Basic hydrolysis is considered to be faster than acidic hydrolysis especially for polyesters. The hydrolytic mechanism under basic and acidic conditions is shown in Fig. 2B and C. Increasing concentrations of lactic acid, hydrolyzed oligomers, and alkali decomposition products have been reported by Dopico-García et al.61 as a function of hydrolysis time using HPLC-UV.
2.5 Biodegradation
Biodegradation is a biochemical process in which organic wastes are transformed back into their original chemical species or other inorganic moieties. This phenomenon is carried out either in the presence of microbes or enzymes. Biodegradation of high molecular weight polymers are mediated mainly by two phases: (1) depolymerization to diffuse across the cell membrane, and (2) cellular metabolism to assimilate the monomeric components. In the first phase, extracellular enzymes, secreted by the microbial cells, facilitate the depolymerization through hydrolysis in which complex polymers break down into dimers or oligomers and ultimately to simple monomeric components. In the second step, cellular metabolisms are to be started when the molecular weight of the polymer becomes low enough to diffuse across the cell membrane where they can be metabolized through various processes to produce CO2 and H2O. Biodegradable plastics, especially polyesters, have received much attention for their potential hydrolysable ester bonds which convert into H2O and CO2 under aerobic conditions and CH4 is produced under anaerobic conditions in addition to H2O and CO2 (Scheme 1). Further, there are three different routes for the degradation of biopolymer, namely: (a) compost, (b) microbial, and (c) enzymatic.
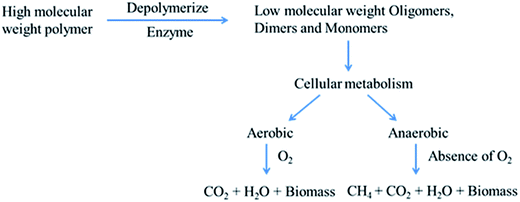 |
| Scheme 1 Scheme of polymer degradation in the presence and absence of oxygen. | |
Singh et al.62 carried out a comparative biodegradation study of poly(hydroxybutyrate-co-valerate) and its nanohybrids with layered silicate in terms of percentage weight loss using compost media, microorganisms (Pseudomonas stutzeri) and pure enzymes (Fig. 3A). The rate of biodegradation was found to be higher significantly in enzymatic media (proteinase-K and lipase) as compared to compost for a similar set of samples. Further, the rate of degradation can be tuned using various nanoclays i.e. the 15A nanoclay increases the degradation rate while the 30B nanoclay reduces the rate of degradation as compared to the rate of biodegradation of pure PHBV and this relative rate remains similar in compost, microorganism and enzymatic media. Microorganisms rapidly convert lactic acid and lactoyl lactic acid into the ethyl ester of lactoyl lactic acid in the biotic environment while it is not detected in abiotic media.63 Lactic acid and lactoyl lactic acids are rapidly assimilated from the polymer films to produce new degradation products such as the ethyl ester of lactoyl lactic acid, acetic acid and propanoic acid in a biotic medium as observed through gas chromatography mass spectrometry (Fig. 3B). The degradation of L- and DL-lactic acid oligomers in the presence of Fusarium moniliforme and Pseudomonas putida was investigated and found lactic acid, lactyllactic acid dimers and higher oligomers in liquid culture. The fate of these compounds was monitored using HPLC. Under the selected conditions, DL- and L-lactic acids were totally used by the two microorganisms regardless of the enantiomer composition.64 Codari et al.65 employed an HPLC calibration procedure to quantify both the production of PLA by polycondensation and its corresponding degradation as shown in Fig. 3C and proposed the mechanism for the hydrolytic degradation of cyclic lactide into the linear dimer which further hydrolyzed into the monomer.
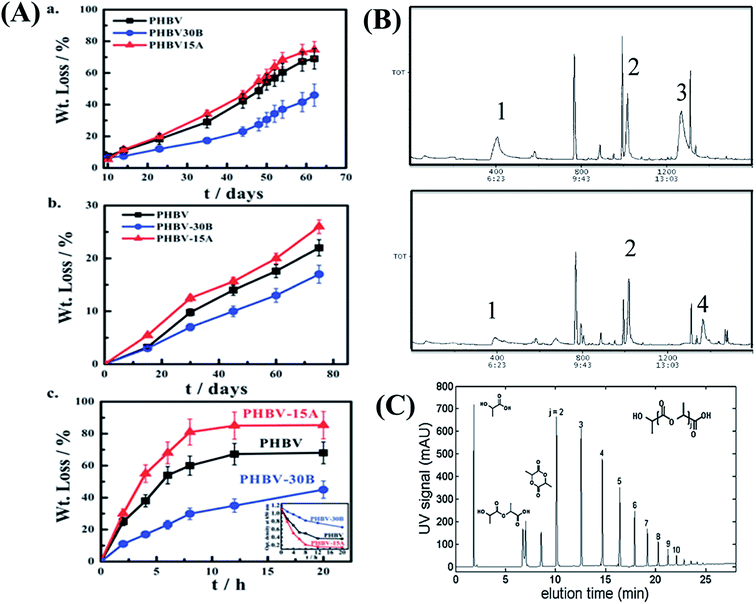 |
| Fig. 3 Degradation in different media and analysis of the degradation products. (A) Percentage weight loss of PHBV and its nanohybrids during biodegradation in different media: (a) compost, (b) microorganism (Pseudomonas stutzeri), and (c) enzyme media (extracted from Pseudomonas stutzeri) showing lower and higher biodegradation rates as compared to pure PHBV using two different nanoclays.62 (B) The GC-MS chromatograms of (top) unaged PLLA and, (bottom) the system after 21 days of incubation in biotic media (this figure has been reproduced from ref. 63 with permission from John Wiley and Sons). Peak 1 is lactic acid, peak 2 is lactide, peak 3 is lactoyl lactic acid, and peak 4 is the ethyl ester of lactoyl lactic acid. (C) Characterization of low molecular weight PLA and its monomer and oligomers using HPLC after biodegradation (this figure has been reproduced from ref. 65 with permission from John Wiley and Sons). | |
2.5.1 Composting. Composting is a biological decomposition and transformation process for treating and recycling biodegradable organic waste into a humus-like substance. Decomposition of waste requires a source of microbial inoculum such as bacteria, fungi, algae etc. and a mineral salt solution in an anaerobic or aerobic environment. Moreover, the real composting conditions are governed by the type of compost and the parameters such as temperature, moisture, and pH of the medium. Elevated temperature, high moisture content and an abundance of microorganisms in the compost is proven to be the most suitable environment for the biodegradation of PLA.66,67 The influence of microorganisms present in the compost during the biodegradation process of PLA and PLA/a-PHB is verified through 1H NMR and ESI-MS analysis of the compost extracts as a function of time.68 Satisfactory disintegration of plastic waste is considered to occur when 90% of its original dry weight can pass through a 2.0 mm sieve.69 Currently available compostable plastics are usually certified by various agencies like European standard EN 13432 (2000) and their counterparts ASTM D 6400-04, ASTM D 6868 (US), AS 4736 (Australia), ISO 17088 and ISO 18606 (worldwide) and BNQ-9011-911/2007 (Canada).68 Biodegradation of poly(hydroxybutyrate-co-hydroxyvalerate) in compost media shows a remarkable enhancement of the biodegradation rate in the presence of clay or hydroxyapatite.70 The surface morphology of pure biopol and its nanocomposites shows a gradual increase in surface roughening during biodegradation. Biodegradation in compost media causes surface roughening due to the removal of polymer molecules whose extent depends on the nanoparticle used following the degradation kinetics.58 The biodegradation in compost media occurs mainly via two steps: (a) the hydrolysis of high molecular weight to lower molecular weight oligomers by using acids, bases or moisture, and (b) consumption of the oligomers by the microorganisms in the compost producing CO2, H2O and humus.70
2.5.2 Microbial degradation. The biodegradation of polymers occurs by the action of a wide variety of microorganisms. The extent of the microbial degradation of polymers depends on the distribution, abundance, diversity, activity and adaptation of microbiota along with the chemical and physical characteristics of the polymers. The techniques employed for the screening of polyester degrading microbes are the plate count method and the clear zone technique on emulsified polyester agar plates (Fig. 4). This is an efficient approach to evaluate the diversity and potency of polymer-degrading microorganisms in the environment.71 The population of aliphatic polyester-degrading microorganisms decrease in the order of PHB = PCL > PBS > PLA.72,73 Gram negative rod-shaped mesophilic bacteria, enriched through the clear-zone method, are active for the degradation of low as well as high molecular weight PLA by attacking both the crystalline and amorphous zones.74 However, different microbial species require either intracellular enzymes or extracellular enzymes which assimilate the degradation products as the sole carbon source. Therefore, the evaluation of the microbial degradation process requires a fundamental understanding of the enzymatic mechanism and will be explained in the enzymatic section. A thermophilic bacterium, Ralstonia sp. (MRL-TL), isolated from hot spring water, can degrade more than 50 wt% of PCL film within 10 days showing esterase activity in the culture broth and the same strain is effective for the degradation of other polyesters such as PES, PLA, PHB and PHBV even under harsh conditions.75
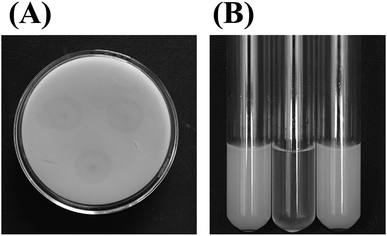 |
| Fig. 4 (A) Colonies and clear zones on a PBS agar plate in the presence of Fusarium sp. FS1301 and (B) degradation of the PBS emulsion by the culture supernatant: uninoculated PBS emulsion (left tube); PBS emulsion incubated with the culture supernatant (middle tube); PBS emulsion incubated with the culture supernatant heated at 100 °C for 5 min (right tube) (this figure has been reproduced from ref. 71 with permission from Elsevier). | |
2.5.3 Enzymatic degradation and its mechanism. Enzymes are biological catalysts, and offer a well-balanced option for the management of plastic waste and thereby develop sustainability. The enzymatic degradation of aliphatic polyesters involves two steps. The first step is the adsorption of the enzyme onto the surface of the substrate through surface-binding domains resulting in the formation of an enzyme–substrate complex, and the second step is the hydrolysis of the ester bonds leading to the formation of soluble degradation products. PHB depolymerase, PCL depolymerase, proteinase K, cutinase, serine proteases, trypsin, elastase esterase and lipases are the extensively studied enzymes used for biopolymer degradation. The stereochemistry of the polymer plays an important role in its degradation e.g. proteinase K degrades the various stereo-isomers of PLA in the following order: L–L > L–D > D–L > D–D at 37 °C and pH 8.6.76 Similarly, PLA50-mes degrades at a faster rate than PLA50-rac due to the higher water uptake ratio which facilitates enzymatic attack.76 The recombinant enzyme DL-PLA depolymerase has the ability to catalyze different kinds of biodegradable polyesters such as PLA, PBS, PBSA, PES and PCL.77 Further, nanoparticles with high surface areas have the advantage of adsorption of the bacterial protease (proteinase K) onto single-walled carbon nanotubes (SWCNTs) resulting in intrinsically high catalytic turnover owing to a greater enzyme loading and a low mass transfer resistance.78 Therefore, bulk modification of biopolymers and surface functionalization of nanoparticles are the prominent choices in the future for the degradation of polymers through hydrolysable ester bonds.Typically, all lipases share highly conserved α/β hydrolase folds consisting of the catalytic triad serine, histidine and aspartate/glutamate as the nucleophilic elbow. The acid residue aspartate/glutamate interacts with histidine to readjust its imidazole ring through shifting hydrogen in such a way that enhances the nucleophilicity of serine. The hydrolysis of polyesters by lipase is divided into two consecutive phases: first is the nucleophilic attack of serine on the ester bond that forms the tetrahedral acyl-serine intermediate which is eventually hydrolyzed by the second attack of the nucleophile i.e. a water molecule.79 The activity of lipase depends on the donating capability of the hydroxyl proton towards the cleavage of the ester linkage as depicted in Fig. 5A. It is pertinent that the pH of the media strongly influences the activity of the enzyme for a particular substrate through a possible alteration of the active site at different pH values as reported.80,81 In other words, the pH of the medium changes the conformation of the enzyme and alters the conformations responsible for varying the hydrolysis rate and, thereby, alters the biodegradation rate of polymers in enzyme media.82 The depolymerase activities of the lipase Pseudomonas cepacia towards PCL, PLA and PU are very different and it exhibits its highest activity in acidic media (pH ∼ 6.5), almost neutral conditions (pH ∼ 7.2) and basic conditions (pH ∼ 7.7) for PU, PLA and PCL, respectively (Fig. 5B). Interestingly, three organically modified nanoclays, namely C18, 15A and 30B, when dispersed in water have pH values close to the peak positions in the curves of depolymerase activity vs. pH.82 The rate of biodegradation was reduced significantly using the other two nanoclays having a different pH. A model of the varied conformation of the enzyme was proposed at different pH values for a particular polymer chain as shown in Fig. 5C.82 For instance, Fusarium solani cutinase exhibits greater degradation behavior at pH 8 (50% weight loss after 6 h) while it loses its activity at pH 3 leading to a meagre weight loss over a similar time period. The overall surface charge around the active site becomes more positive in highly acidic conditions resulting in a loss of cutinase activity for PCL degradation. In contrast, cutinases derived from Humicola insolens show significant weight loss (60%) at pH 3 after 6 h demonstrating the loss of enzyme activity as a function of pH. The electrostatic surface potentials of Fusarium solani (FsC) at pH 5 and 3 are calculated to be 4.4 and 13.3, respectively, while Humicola insolens (HiC) exhibits values of 1.1, 5.6, 15.8 at pH 8, 5.0 and 3.0, respectively.83 Hence, engineering the surface charge and adding appropriate disulfide bonds could lead to an improvement of the function and stability of the enzyme over a range of pH values. The protonation of all ionizable side chains at low pH leads to charge–charge repulsion and consequently protein unfolding leading to the loosening of side chain packing, and the exposure of hydrophobic groups to the solvent which abolishes both the structure and function of the enzyme.84 The enzymatic degradation of poly(butylene succinate-co-hexane succinate) (PBS-co-HS) is found to be higher than that of poly(butylene succinate-co-butylene adipate) (PBS-co-BA), arising from the isomerism effect of the copolymer backbone. The enzymatic degradation of PBS-based copolymers has been investigated using MALDI-TOF-MS and the m/z values correspond to linear monomers and ring oligomers. In addition, the mechanism of the enzymatic degradation and enzyme substrate complex formation has been studied through molecular docking. The docking of the substrate containing hexane succinate (HS) in the active site of Pseudomonas cepacia (PC) lipase is more stable than for other substrates. Since the ester bonds react with the catalytic residue of Ser87 in the active pocket, the carbonyl groups were attached with the oxygen anion residues of Leu17 and Gln88 which facilitates a smooth catalytic process (Fig. 5D). It is worth noting that the introduction of linear hexa-monomers increases the hydrophobicity of PBS copolymers and, thereby, enhances the catalytic degradation ability. During coupling to the PC lipase enzyme, PBS-co-HS can reach the bottom of the active pocket to bind the active residue and, hence, increase the catalytic efficiency by improving the degradation performance.85 The degradation of poly(butylene succinate) (PBS) and its three copolymers, the PBS and ε-caprolactone copolymer (PBS-co-CL), the binary copolymer of PBS and 1,4-cyclohexane dimethanol (PBS-co-CHDM), and the ternary copolymer of PBS, PCL, and 1,4-cyclohexane dimethanol (PBS-co-CL-co-CHDM), are catalyzed by immobilizing lipase in a mixed organic solvent containing a small amount of water. The extent of the degradation is found to be 40, 43, 53 and 54% in PBS, P(BS-co-CL), P(BS-co-CHDM), and P(BS-co-CL-co-CHDM), respectively. Degradation products from PBS-co-CL are confirmed through NMR analysis (Fig. 5E).86
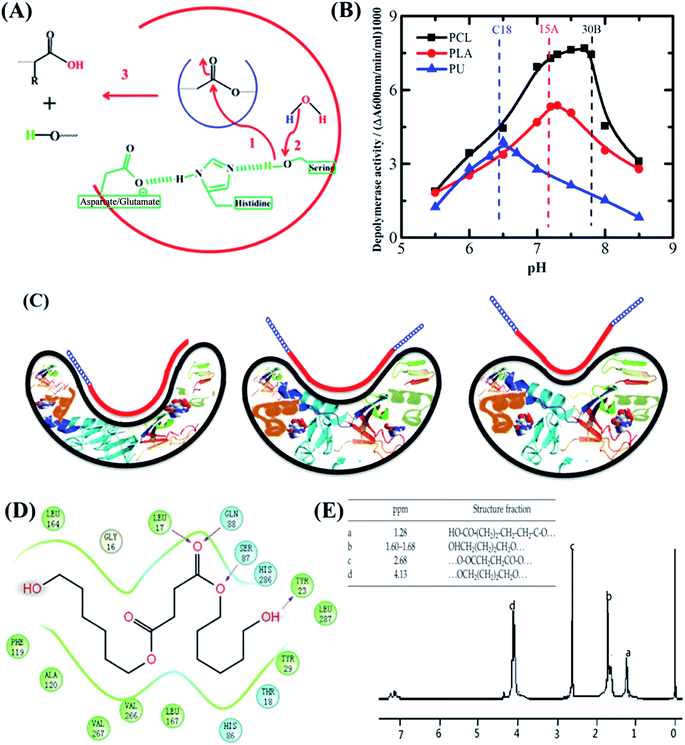 |
| Fig. 5 Mechanism of enzymatic degradation: (A) the proposed mechanism of the catalytic degradation of polymers using a specific amino acid series and complexation;82 (B) the depolymerase activity of lipase Pseudomonas cepacia towards three different polymers as a function of the pH of the medium. The vertical lines indicate the pH of the nanoclay dispersion which also matches with the highest degradation rate of the three separate polymers;82 (C) models depicting the conformational changes of the enzyme at various pH values which are responsible for the varying biodegradation rate of an enzyme at different pH values (these figures have been reproduced from ref. 82 with permission from Elsevier). (D) Ester group interaction with the amino acid residue of the active site (this figure has been reproduced from ref. 85 with permission from Elsevier) and (E) NMR spectrum of the degradation products of PBS-co-PCL showing various byproducts as mentioned (this figure has been reproduced from ref. 86 with permission from John Wiley and Sons). | |
3. Factors influencing the biodegradability of polymers
In this section, the parameters which influence the degree of biodegradability for different polymers have been taken into account. The biodegradability depends on the chemical and physical characteristics of the polymers along with biotic and abiotic factors (Table 2). Abiotic parameters such as pH, temperature, humidity, salinity, presence or absence of oxygen and the supply of essential nutrients not only affect the polymers to be degraded but also influence the biotic factors such as microbial diversity, population and action potential of enzyme activity. Moreover, the extent of degradation also depends on the characteristics of the polymer including morphology, crosslinking, purity, diffusivity, porosity, chemical reactivity, mechanical strength, thermal tolerance, and resistance to electromagnetic radiation.3 The surface morphology (surface area, shape, size, hydrophilicity, water permeability, and hydrophobic characteristics), intrinsic properties (monomeric composition, presence of terminal carboxyl or hydroxyl groups, type of enantiomer, alignment of groups and their crosslinking, and molecular weight and its distribution), high order properties (glass transition temperature, melting temperature, modulus of elasticity etc.) and processing conditions (ratio of the crystalline and amorphous regions, copolymerization, choice of additives and organic/inorganic fillers) of the polymers play important roles in determining the degradation kinetics and influence the mechanism of biodegradation.73,87 Aliphatic polyesters show greater susceptibility to lipolytic enzymes and microbial degradation in comparison to aromatic polyesters and polyurethanes owing to their monomeric composition. The rate of biodegradation is reduced with the increase of molecular weight e.g. low molecular weight PCL degrades more rapidly compared to high molecular weight PCL using lipase. Further, the low molecular weight oligomers degrade fully regardless of enantiomeric composition contrary to the faster degradation of high molecular weight L-PLA as compared to D-PLA.3,73 Moreover, the morphology of the polymers greatly influences their rate of biodegradation.88 The fractions of crystalline and amorphous domains play an important role in determining the biodegradability. The polymer chains within the crystal structure are placed in an orderly fashion making it more resistant against degradation while the amorphous domains are loosely packed and thereby susceptible towards microbial attack and enzyme catalysis. The biodegradation rate has been significantly reduced for samples crystallized at 52 °C as compared to room temperature quenched samples for pure PCL due to the greater amount of crystallinity as evident from the higher heats of fusion and bigger size of spherulites for the crystallized sample vis-à-vis the quenched one.81 The rate of degradation of PLA decreases with an increase in the crystallinity of the polymer. In general, a higher melting temperature (Tm) lowers the biodegradation of the polymer because of interactions amongst the polymer chains which affect the heat of fusion (ΔH) value and the internal rotational energies corresponding to the rigidity (or the flexibility) of the polymer molecules.89 Low molecular weight L-PLA and their copolymers such as DL-PLA, poly(L-lactide-co-glycolide) and poly(D-lactide-co-glycolide) are hydrolyzed by lipase but D-PLA, PGA, and high molecular weight L-PLA are not easily hydrolysable and are resistant to enzymatic degradation due to their high crystallinity and high melting temperatures (Tm).73 Hence, the physical properties and biodegradation behavior can be tuned through copolymerization to make them more susceptible to biodegradation by lowering the crystallinity and Tm compared to those of the homopolymers. Fillers embedded in the polymer matrix also play an important role in the control of the rate of biodegradation of the polymer and a good dispersion of filler (exfoliated nanostructure) degrades the polymer at a faster rate as compared to a composite having an intercalated nanostructure or the pure polymer.90
Table 2 Factors affecting the biodegradation of polymers
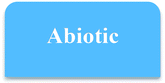 |
pH, temperature, moisture, electromagnetic radiation, salinity |
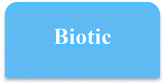 |
Saprophytic diversity (bacteria and fungi), type of enzymes and their concentrations |
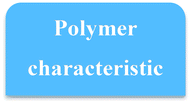 |
Molecular weight, monomeric composition, pattern of crosslinking, morphology (structure, shape and size), route of processing, percentage of crystal and amorphous content, presence of active functional groups, type of copolymer and composite, hydrophobicity |
4. Techniques and methods being assessed for polymer degradation
The most inexpensive method used to measure the extent of biodegradation is the weight loss or mass loss of the test specimen which provides real evidence of degradation. The high throughput analytical techniques used for the molecular characterization of the products formed after degradation are chromatography techniques such as GC, GPC and HPLC which may be coupled with mass spectrometry and NMR. Chromatography is a non-destructive separation technique used to fractionate the mixtures according to molecular size or partition coefficient primarily used in the determination of molecular weights in polymer science. The surface topology revealing surface roughening or pitting of degraded materials is observed through either scanning electron microscopy (SEM), atomic force microscopy (AFM) or optical microscopy. The extent of crystallinity as a result of weight loss of the polymer is most frequently studied using X-ray diffraction (XRD) and differential scanning calorimetry (DSC). Melting (Tm) and glass transition (Tg) temperatures of polymers also play a role in the varying degrees of biodegradation. The comparative study of tensile strength before and after biodegradation provides useful information about the mechanical stability of the degraded materials.60,69,91,92 An expensive technique used to elucidate the metabolic disposition and elimination of CO2 is radiolabelling using 14C labeled test materials that evolve 14CO2 during degradation which is then estimated by a scintillation counter. The polyester urea–urethane labeled with 14C-labeled ethylene diamine was susceptible to enzymatic degradation monitored by the radiolabel released.93 The short-lived isotopes such as 18F, 68Ga and 13N have been used in pre-targeting concepts which offer a great flexibility regarding the time point of imaging and demonstrate the fundamental importance of the initial few hours.94 The major drawback of this technique is waste disposal management.
Different standards that have been developed by authorized organizations to account for the extent of degradation are ASTM (USA), DIN (Germany), JIS (Japan), ISO (international standards), and CEN (Europe). The biodegradation studies of different polymers have been tested using standard methods ISO 14851 (for aerobic conditions), ISO 14853 (for anaerobic conditions), ASTM G 21-90 and ASTM G 22-76 and NF X 41-514 etc.95 To evaluate the efficiency of the biodegradation, quantitative parameters such as mass variation, oxygen uptake, pressure variation, biogas generation and composition, and biodegradation percentages were estimated after 28 days of degradation.95 The microbial oxidation of polymers requires oxygen for their biodegradation. The kinetics of oxygen consumption as functions of biochemical oxygen demand (BOD) and CO2 exhaust are good indicators for polymer degradation and are determined by respirometric tests and Sturm test methods, respectively. Pressure built in the test bottles under anaerobic conditions was calculated as a volume of biogas produced during the degradation of a starch/polycaprolactone blend (Mater-bi ∼ MB) and was found to be 9.4% CO2 and 23% CH4 at the end of 28 days of incubation. Biodegradation of MB under aerobic conditions was found to be approximately 45% but under anaerobic conditions only 25% was degraded.95 Kasuya et al.96 measured the weight-loss and biochemical oxygen demand of biosynthetic poly(3-hydroxybutyrate-co-14% 3-hydroxyvalerate) films which were found to be 100% and 78 ± 8% after 28 days, respectively. Calil et al.97 compared the biodegradation of poly(ε-caprolactone) (PCL), cellulose acetate (CA) and their blends as a function of carbon dioxide (CO2) production using the Sturm test method. The 40PCL/60CA blend showed faster biodegradation than the other blend because of its higher CO2 production in aerobic media (Sturm test). Blending PCL with CA reduces the melting temperature (Tm) of PCL and increases the Tm of CA indicating that the immiscibility of the polymers facilitates the decrease in crystallinity and increase in the amorphous region which in turn favors biodegradation.97 CO2 produced during biodegradation was measured using the Sturm test (OECD, 1992) and was further verified using gas phase chromatography and infrared spectroscopy.98 The degradation of PLGA was evaluated at two different pH conditions through HPLC, GC and SEM. SEM images reveal surface pitting and pore formation (heterogeneous degradation) in degraded specimen with varying pH of the media. This heterogeneous degradation resulted in the random formation of channels within microspheres which was not observed in those samples degraded at different pH values of ∼ 2.4. However, the local micro-environmental pH within the microspheres has been reported to vary considerably due to a buildup of acidic oligomers at different pH values.99
Different plots of molecular weight and nth-order mass defects (up to n = 3) have been proposed for assessing the structure of the repeating units and estimating their absolute and relative number per molecule as quantified through reversed-phase HPLC and high resolution mass spectrometry. Tandem MS was used to identify the end groups and the possible distribution of the repeating units. Reversed-phase HPLC enabled the separation of the homologous series of individual polymer molecules on the basis of increasing numbers of the nonpolar repeating units.100 The degradation profile of glycolide and lactide linkages was evaluated using HPLC revealing faster degradation of PLGA microspheres compared to that of PDLLA particles due to higher hydrophilicity.101 Schliecker et al. studied the degradation rate and degree of crystallinity in oligomer-containing films and found initial mass loss and water absorption. The number average molecular weight (Mn) of the oligomer-containing films decreased more than the weight average molecular weight (Mw).102 Using X-ray diffraction, PLA50 was characterized initially to be amorphous showing three diffraction peaks after 28 days (2θ = 12.0°, 20.8° and 24.0°) corresponding to the crystalline structure of a PLA stereo complex. The value of Tg initially was reported to be 46.4 °C which decreased to 29.2 °C after degradation. Further, a small melting peak Tm ∼ 115.4 °C was detected after 21 days of biodegradation showing the formation of crystalline residues.103 The emergence of low molecular weight oligomers as a result of the degradation of the poly(fumaric-co-sebacic anhydride) [P(FA:SA)] copolymer was characterized using area calculations for the carboxylic acid and anhydride carbonyl peaks through Fourier-transform infrared spectroscopy (FTIR), a decrease in the molecular weight using gel permeation chromatography (GPC), thermal analysis using differential scanning calorimetry (DSC) and the detection of peaks from XRD measurements. DSC thermograms showed that melting emerged at temperatures above the polymer melt and below the monomer melting indicating the formation of oligomeric material after degradation correlated with the decrease of relative crystallinity of the copolymer. The weight-average molecular weight (Mw) rapidly decreased while the number-average molecular weight remained fairly constant throughout the experiment strongly suggesting the formation of stable oligomeric chains that are more resistant to hydrolytic degradation than larger copolymer chains. The emergence of a peak at 30° during X-ray diffraction is combined with the data from FTIR and DSC to show that the biodegradation of polymers produces oligomers but not monomers.104 The hydrogen bonded interaction between PHBV and the hydroxyl group in the gallery of silicate layers of organically modified montmorillonite (OMMT) enhances the weight loss in nanocomposites.105 The biodegradation of poly(L-lactide–glycolide–trimethylene carbonate) after 10 weeks of implantation retains approximately 1/10 of the initial sample mass and the remaining material is resorbed into the body. The SEM micrograph reveals the creation of the characteristic manifestations of heterogeneity in its volume as a result of the progressive penetration of the physiological medium (liquid) during biodegradation.106
The degradation of polymeric blends of corn starch with poly(ethylene-vinyl alcohol) copolymer and poly(ε-caprolactone), designated by SEVA-C and SPCL, respectively, were incubated in buffer solution containing enzymes for 6 weeks. The degraded materials were characterized using a gravimetric measurement, tensile testing, scanning electron microscopy (SEM), and Fourier transform infrared-attenuated total reflectance (FTIR-ATR). α-Amylase catalyzes the glycosidic linkage and causes a significant decrease in moduli of about 65% and 58% in SEVA-C and SPCL, respectively, as opposed to the control (samples incubated in buffer only). FTIR spectra confirmed a decrease of the band corresponding to the glycosidic linkage (–C–O–C–) of starch. Furthermore, high performance anion exchange chromatography with pulsed amperometric detection (HPAEC-PAD) identified glucose as a major product of the enzymatic degradation.107 Biodegradation of polyesters such as poly(butylene succinate) (PBSu), poly(butylene adipate) (PBAd) and poly(butylene succinate-co-adipate) (PBSA) were catalyzed by three enzymes. The maximum efficiency of the weight loss of the homopolymers and copolymers after 15 days was found to follow the order of P. fluorescens cholesterol esterase > R. delemar lipase > C. cylindracea lipase. SEM micrographs clearly showed the formation of a rough surface with large holes.108 The surface topology demonstrated heterogeneous degradation (from surface to core) for high molecular weight PLA microspheres that maintain their shape even after hydration and incubation at 37 °C in phosphate-buffer for a prolonged period.109
The subtle molecular structure of biodegradable PHB and its synthetic analogues, formed through anionic ring-opening polymerization (ROP) of β-substituted β-lactones, was characterized using mass spectrometric methods (ESI-MS). The assessment of the polymer degradation products having characteristic end groups was revealed and differentiated using the application of modern MS techniques.110 Mäder et al. employed the non-invasive real time techniques, magnetic resonance imaging (MRI) and electron paramagnetic resonance spectroscopy (EPRS), to monitor the polymer erosion, shape and size of the implant and their consequence on the physiological responses. The polymer erosion from biodegradable P(FAD–SA)
:
polyanhydride (50
:
50) was almost entirely absorbed after 44 days whereas an encapsulated residue was found for the 20
:
80 composition even after 65 days. In vivo responses such as edema, bioresorption and deformation of the implant were observed using MRI.111 Woo et al. studied the degradation of drug embedded polymers incubated in the solutions of cholesterol esterase (CE). The extent of biodegradation was assessed using high-performance liquid chromatography (HPLC), mass spectrometry (MS) and 14C radiolabel release. The radiolabel release with enzymes was initially high within the first ten days and was followed by a slower but increasing release of product with time showing specific radioactivity of the drug.112 The degradation due to aging can lead to the changes in the polymer segmental motion as well as the chemical structure, both of which can be monitored using nuclear magnetic resonance (NMR) spectroscopy. Somers et al. stated that the degree of degradation was reciprocally related to the 1H NMR transverse relaxation time while the 13C spectra showed considerable peak broadening, indicative of decreased mobility with an increased level of degradation.113 The metabolic disposition and elimination process of the anhydride copolymer poly[1,3-bis(p-carboxyphenoxypropane) ([14C]CPP):sebacic acid (SA)] implanted in rats accounted for approximately 40% of the radioactivity in the expired CO2, 10% in the urine and ∼2% in the faeces, and about 10% remained in the device after 7 days of implantation. Thus, the biodegradable polymer can provide much information about the specific delivery of drugs into a targeted organ and the continuation of drug release of over a considerable time period.114 Wolf extensively reviewed the radiolabeled polymers containing covalently bound 3H and 14C materials as a benchmark tool for in vivo and in vitro studies for the detection of polymer degradation, the elucidation of the mechanistic approach and the bio-incorporation approach, and drug delivery.115
5. Biodegradation of different polymers and the influence of nanoparticles
5.1 Poly(lactic acid) and its copolymers
Poly(lactic acid) (PLA) is an aliphatic polyester made up of lactic acid (2-hydroxy propionic acid). It is produced by animals, plants and microbes biologically through fermentation under oxygen limited conditions. Synthetically, it can be derived from the intermediates of renewable materials (e.g. ethanol, acetylene or acetaldehyde).116 PLA is a compostable polymer and, thereby, alleviates solid waste disposal problems. Two main simulated composting methods have been used to assess the biodegradability of PLA bottles showing similar results of biodegradation with mineralization: (i) cumulative measurement respirometric (CMR) systems, and (ii) gravimetric measurement respirometric (GMR) systems.117 The modulation of the degradation period has been done by coating PLA with PCL for possible use in biomedical devices.118 PLLA coated with PCL shows a slower degradation rate than an uncoated sample suggesting the usefulness of hydrophobic coating to control the degradation of polymeric devices. The enzymatic degradation of PLLA fiber-reinforced PCL (FRP) and PCL/PLLA blend films has been investigated using Rhizopus arrhizus lipase and proteinase K.119 In vitro degradation of poly(lactic acid)/phosphate glass fiber composites shows a bimodal pattern above 37 °C and the peaks shift toward low molecular weights at higher temperatures. A significant increase in crystallinity is observed after degradation at higher temperatures due to the predominant degradation of the amorphous regions of the polymer chain.120 However, poor toughness and hydrophobicity of PLA restrict its application in various fields which is usually improved by preparing its nanocomposite using nanometer scale particles embedded in the polymer matrix. The nanoparticles have advantages over their micrometer size counterparts as better interactions can be achieved through the higher specific surface area of the nanoparticles. The nanoparticles act as nucleating agents, and thereby control the spherulite dimensions of the matrix which further control the rate of biodegradation. Better interaction leads to an increase in the amorphous zone and also improves the degradation of the polymer matrix. Polylactide layered silicate nanocomposites (PLA-30B) showed a considerable improvement in biodegradation as compared to the neat polymer matrix. The presence of excess hydroxyl groups in the organic modifier of the 30B nanoclay accelerates the hydrolytic decomposition.58 A greater interaction between PLA and functionalized MWCNTs (PLA–FMWCNTs) results in the smallest spherulitic dimension in the PLA–FMWCNTs which then becomes susceptible to enzymatic hydrolysis due to the larger amorphous zone. The morphology of pure PLA and its nanohybrids before and after biodegradation as observed using SEM is shown in Fig. 6A to compare the relative rates of biodegradation showing a greater loss of material from the 30B nanocomposite as compared to pure PCL and the nanocomposite containing the 15A nanoclay.58 Similar biodegradation behavior in the presence of various nanoparticles (e.g. MAE, MEE nanoclay) demonstrates a faster rate by controlling the amorphous content or size of spherulites of PLA.121
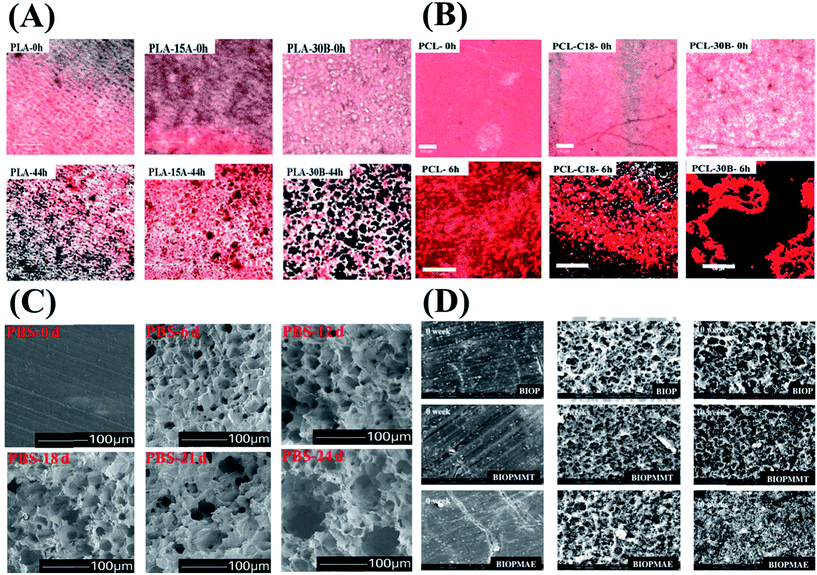 |
| Fig. 6 Comparative degradation using surface images of PLA, PCL, PBS and Biopol: (A) laser scanning confocal microscopy images of PLA using 15A and 30B nanoclays (this figure has been reproduced from ref. 58 with permission from John Wiley and Sons). (B) Laser confocal microscopy images of PCL and its indicated nanocomposites using C18 and 30B nanoclays (this figure has been reproduced from ref. 81 with permission from American Chemical Society). (C) SEM micrographs of different degraded PBS films (this figure has been reproduced from ref. 71 with permission from Elsevier). (D) SEM images of the biopol and its nanocomposite sample surfaces before and after 5 and 10 weeks of biodegradation using MMT and MAE nanoclays.70 | |
PLGA is a type of copolymer that is synthesized via ring-opening polymerization of lactide and glycolide. Tuning of the properties of polymers is done by varying the ratio of monomers. Higher ratios of hydrophilic PGA increase the degradation rate of PLGA.122 Comparative degradation studies of biodegradable microspheres of poly(DL-lactide-co-glycolide) with poly(ethylene glycol) derivatives are reported.123 PLGA is the most utilized polymer in the biomedical arena due to its biocompatibility and controlled degradation behavior. It is degraded by the hydrolysis of its ester bonds yielding nontoxic lactic acid and glycolic acid that can be removed from the body by normal metabolic pathways.124 The synthesis of PEG–PLGA–PEG triblock copolymers forms a free-flowing sol at room temperature which becomes a gel at body temperature. The gel undergoes degradation by hydrolysis and shows preferential mass loss of the PEG-rich segment from the in situ gel.125 Chang et al. used the non-ionic amphiphilic biodegradable PEG–PLGA–PEG copolymer to enhance the gene delivery efficiency in rat skeletal muscle.126
5.2 Poly(ε-caprolactone)
Poly(ε-caprolactone) (PCL) is a synthetic aliphatic polyester produced via ring-opening polymerization. Even though it is synthetic in origin, it is known for its biodegradability and non-toxicity. Penicillium oxalicum (DSYD05-1) degrades PCL in 4 days producing water-soluble 6-hydroxyhexanoic acid as observed through mass spectrometry, which suggests an exo-type chain-end scission by the enzyme.127 A graphene/polycaprolactone composite is recognized as a promising biodegradable material showing its non-toxic nature through cell proliferation.128 Fine-tuning of the biodegradation rate of the nanocomposite, either to increase or decrease with respect to the natural degradation rate of the pure polymer, is possible by controlling the pH of the medium using different nanoparticles. Therefore, the greater enzyme (PCL-depolymerase) activity in a slightly alkaline nanocomposite causes more biodegradation in the presence of nanoparticles. It should be mentioned here that the pH of the PCL solution is ∼7.0. A more basic pH in the PCL-30B nanocomposite explains the higher biodegradation rate as compared to that for the PCL-C18 nanocomposite. The enhanced enzymatic activity in the nanocomposites is due to the alkaline pH together with the greater amorphous content as compared to pure PCL. The relative morphology as observed through scanning confocal images (Fig. 6B) also reflects the highest enzymatic degradation in PCL-30B and the lowest rate in pure PCL as evident from the relatively roughened surface.81 Allı et al.129 demonstrate the enzymatic degradation of various grafted PCLs (PLina-g-PCL and PLina-g-PSt-g-PCL copolymers) showing controlled degradation in the presence of Pseudomonas lipase depending on the nature of the comonomer and its extent. Multicomponent composites of PCL (filled with the fibers of PHB) with cationic (Cloisite) or anionic (Perkalite) nanoclays are reported with varying degrees of biodegradation. The type and amount of clay and fibers in the PCL matrix control the rate of biodegradation. The filled PHB fibers allow the modulation of the properties of the thermal stability of PCL and prevents the biodegradation process.130 The effect of two different multiwall carbon nanotubes, namely pristine (p-MWNTs) and amine functionalized (a-MWNTs) nanotubes, on the biodegradation of PCL has been studied by Pawar et al.131 using lipase and it has been shown that the rate is sluggish in the presence of the p-MWNT composite while the biodegradation is similar in presence of a-MWNTs with respect to the natural degradation of pure PCL.
5.3 Poly(butylene succinate)
Poly(butylene succinate) (PBS) is one of the most promising biodegradable aliphatic polyesters, invented in the early 1990s by Showa Highpolymer in Japan. It is a competitive material against other biodegradable plastics due to its superior mechanical and thermal properties, high chemical resistance and good processability. However, certain properties of PBS such as gas-barrier properties, softness, and melt viscosity for further processing are insufficient for various applications.132 Biodegradable nanocomposites of PBS with organo montmorillonite (OMMT) have been prepared using maleic anhydride grafted PBS (PBS-g-MA) as a compatibiliser, which show a slower weight loss (biodegradation) than neat PBS due to the enhanced barrier properties on addition of OMMT. Biodegradation of poly(butylene succinate) (PBS)/jute composites in compost-soil shows ∼62% weight loss after 180 days, considerably higher rate as compared to that in pure PBS. The rate of biodegradation of PBS increases with an increasing jute percentage in the composites. Cotton fiber (CF) is used as a filler in PBS to improve the biodegradation rate of the composites in addition to strengthening the polymer matrix. Carbon fiber (CF) treated with a silane coupling agent further increases the rate of biodegradation.133 Bacteria (e.g. Burkholderia cepacia PBSA-1 and Pseudomonas aeruginosa PBSA-2, isolated using the enrichment culture and the clear zone test) were used to degrade poly(butylene succinate-co-butylene adipate) (PBSA) showing a very high activity (78% weight loss of PBSA) using an initial concentration of 0.01%.134 The biodegradation behavior of PBS films in the presence of Fusarium was characterized through differential scanning calorimetry and scanning electron microscopy (Fig. 6C) showing a gradually roughened surface over the time period of biodegradation.71 The main degradation products are found to be 1,4-succinate, succinate–butane diol, succinate–butane diol–succinate and succinate–butane diol–succinate–butane diol as determined by mass spectrometry.135
5.4 Poly(hydroxy alkanoate)
Poly(hydroxy alkanoate)s (PHAs) are natural occurring biodegradable and biocompatible polymers which are attractive biomaterials in clinical applications owing to their microbial origin. Biodegradable polymers of microbial origin are desirable especially for bio-implants to avoid the need for another surgery to remove non-degradable polymers. Since the poly(hydroxy butyrate) (PHB) homopolymer is hard and brittle, it is not suitable for a broad range of applications. The copolymer of 3-hydroxyvalerate (HV) with 3-hydroxybutyrate (HB), commercially known as ‘Biopol’, has been designed to improve the physical properties. So far, only a few PHAs including poly(3-hydroxybutyrate) (PHB) and copolymers of 3-hydroxybutyrate and 3-hydroxyvalerate (PHBV), poly(4-hydroxybutyrate) (P4HB), copolymers of 3-hydroxybutyrate and 3-hydroxyhexanoate (PHBHHx) and poly-3-hydroxyoctanoate (PHO) are available for medical research.62 The blend film of poly(hydroxybutyrate-co-valerate) (PHBV) and poly(ε-caprolactone) (PCL) PCL/PHBV (4
:
1) is completely biodegradable in 30 days and the PHBV film is more susceptible to microbial attack than the PCL film.135 The biodegradation of the natural polyesters poly(β-hydroxybutyrate) (PHB) and poly(β-hydroxybutyrate-co-hydroxyvalerate) (PHBV) is studied in two anaerobic sludges exhibiting significant degradation over a time scale of 6–10 weeks as monitored by weight loss and biogas formation. PHB degrades more rapidly in anaerobic environments as compared to its copolyester PHBV and the degradation rate is relatively slow in aerobic conditions.136 The introduction of two-dimensional nanofillers into PHA has been shown to give a PHA-based bionanocomposite with improved properties. The nanoparticles improve the physical properties and cause a controlled biodegradation within a desirable time frame under specific physiological conditions for the explicit needs of biological applications. Singh et al.62 tuned the biodegradation rate of PHBV using two different organically modified nanoclays (30B and 15A). A greater rate was observed in the presence of the 15A nanoclay while the rate was dramatically sluggish in the 30B containing composite as compared to the degradation rate of pure PHBV. SEM images of the biopol and its nanocomposite sample surfaces before and after 5 and 10 weeks of biodegradation are shown in Fig. 6D indicating greater biodegradation using MMT vis-à-vis the MAE nanoclay amongst nanocomposites for a similar amount of nanoclay.70 It should be mentioned that the surface morphologies of all the cases mentioned correlate with the relative biodegradation rates as observed through their kinetics.
5.5 Others polymers
5.5.1 Cellulose. Cellulose is a linear polymer composed of D-glucose subunits linked by β-1,4-glycoside bonds forming cellobiose molecules. It appears in either an organized or non-organized chain form, called crystalline or amorphous cellulose, respectively. Cellulose is susceptible to enzymes or microbes (mostly eubacteria and fungi) with the establishment of a synergistic interaction with non-cellulolytic species leading to the complete degradation of cellulose.137 Fungi like Trichoderma reesei and Phanerochaete chrysosporium secret cellulase enzymes for the biodegradation of cellulose after hydrolyzing the backbone linkages, the β-1,4-glycosidic bonds of cellulose.138 Enzymes efficiently degrade both crystalline and amorphous cellulose. Out of the two classes of cellulose, endoglucanases (EGs) hydrolyze the internal bonds of amorphous cellulose releasing new terminal ends while cellobiohydrolases (CBHs) act on the endoglucanase generated chain ends. Thus, CBHs and EGs release cellobiose molecules which are further hydrolyzed to glucose molecules by the β-glucosidases enzyme.137
5.5.2 Starch. Starch is composed of two distinct macromolecules (1) amylose: a linear α-D-glucopyranosyl unit linked with α-1,4 bonds, and (2) amylopectin: a highly branched fraction of α-1,4 linked glucan chains connected by α-1,6 linkages, and it is considered as a major energy reserve for a large variety of green plants.139 Starch-based biodegradable plastic is one of the most economic, abundant and renewable biomaterials widely used for biomedical and disposal purposes. Chemically or physically modified starch-based materials are frequently studied to understand degradation kinetics and drug delivery. In vitro enzymatic degradation by fungal-amylase is used to hydrolyze starch films with different molecular, crystalline and granular types in order to understand the effect of different structures on enzymatic degradation.140 Degradation of cassava starch based composite films is investigated using the indoor soil burial method. Increased water sorption promotes the entry of soil microorganisms and the starch films are utilized as a source of energy for their growth. The loss of matrix components of the films is monitored by the reduction in weight and the morphology is studied through scanning electron microscopy.141
5.5.3 Gelatin. Gelatin is derived from the fibrous insoluble protein called collagen by chemical denaturation and is comprised of three polypeptide chains having a repeating Gly–X–Y sequence (glycine–proline–hydroxyproline) arranged in a triple helix, stabilized through hydrogen and hydrophobic bonds. Changes in pH and temperature, or presence of the denaturing chemicals can cause a disruption of the gelatin structure arising from the loss of the triple helix conformation. The processing of gelatin needs to be carefully controlled to achieve a high gelling strength avoiding the extensive degradation of the peptide structure.142 The degradation of gelatin and its composite with MMT by lysozyme and proteinase K has a much faster rate in CPX–gel (ciprofloxacin loaded) as compared to CPX–MMT–gel or MMT–gel mainly due to their 3D structures.143 The CPX–gel degrades rapidly because of the large number of hydrophilic amino and carboxyl groups and the physical structure of the CPX–gel scaffold has a higher porosity and leaner pore walls. Thus, the gelation and biodegradation are the two key factors that affect the cellular behavior and tissue regeneration and ultimately control the in vivo drug delivery.144
5.5.4 Alginate. Alginate, a water-soluble linear polymer obtained from brown algae, is composed of (1-4)-β-D-mannuronic acid (M) and (1-4)-α-L-guluronic acid (G) units in the form of homopolymeric (MM- or GG-blocks) and hetero-polymeric sequences (MG- or GM-blocks).145 The degradation kinetics of alginate are controlled by varying the molecular weight, chemical structure and crosslinking. The modulation of the degradation rate of alginate gels is done via an oxidation reaction using uronic acid residues by inducing hydrolytically labile acetal-like groups within the alginates.146 The oxidation of alginate leads to the cleavage of the carbon–carbon bond of the cis-diol groups. However, the oxidation makes the gel more malleable leading to an inverse relation between degradation rate and gel stiffness.
5.5.5 Chitosan. Chitosan is one of the most important biodegradable and biocompatible polymers, composed of β-(1,4) linked 2-deoxy-2-amino-D-glucopyranose and partially of β-(1,4) linked 2-deoxy-2-acetamido-D-glucopyranose and is obtained by the alkaline deacetylation of chitin which is the major component of the exoskeleton in crustaceans.147 Chitosan is hydrolyzed (chitosanolysis) by specific enzymes (chitinase/chitosanase) and non-specific enzymes (cellulases, pectinases, papains, lipases) as reported by Xia.148 Brzezinska et al.149 isolated the chitinase producing actinomycete Streptomyces rimosus for the biodegradation of chitinous substances. The lysozyme loaded chitosan films exhibit a ∼21% mass loss after 4 weeks of implantation in rats compared to the control that suffers only a 7% mass loss. The degradation products of monomers and oligomers of glucosamine and N-acetyl-glucosamine have been detected using capillary electrophoresis-mass spectroscopy.150
5.5.6 Guar gum. Guar gum is a polygalactomannan obtained from the seeds of Cyamopsis tetragonalobus, found in the north western region of India. Guar gum (GG) is a non-ionic, water soluble and biodegradable hetero-polysaccharide, composed of a β-(1 → 4) D-mannose backbone randomly linked with α-(1 → 6) D-galactose units.151 The enzymatic hydrolysis of guar gum significantly affects its physicochemical and rheological characteristics which become useful for its incorporation in food products as dietary fiber.151
5.5.7 Agar. Agar is a mixture of heterogeneous galactans, mainly composed of 3,6-anhydro-L-galactoses (or L-galactose-6-sulfates), D-galactoses and L-galactoses alternately linked by β-(1,4) and α-(1,3) linkages.152 Many microorganisms that can hydrolyze and metabolize agar as a carbon and energy source have been identified in seawater and marine sediments. Agarolytic microorganisms such as Saccharophagus degradans and Streptomyces coelicolor commonly produce α-agarase, β-agarase and β-porphyranase enzymes which catalyze the hydrolysis of agar.152 During the photo degradation process, temperature and humidity fluctuations promote a decrease in agar’s mechanical properties caused by a reduction in molecular size and a decrease in the number of sulfate groups. These variations alter agar’s crystallinity and lead to the formation of micro-fractures and embrittlement and promote microbial attack.153
5.6 Conventional fillers and their effect on biodegradation
5.6.1 Metal salt based fillers. The weight loss of silica-filled polylactide (PLA) nanocomposites prepared through melt compounding has shown a linear variation during enzymatic degradation. For neat PLA, the biodegradation rate is around 0.055 mg cm−2 h−1; however, the biodegradation rate is increased to be around 0.36 mg cm−2 h−1 for the sample with 9 wt% nanosilica (PLA9). Fig. 7A shows the faster biodegradation of PLA nanocomposites as compared to that of the neat polymer since silica is hydrophilic which leads to a facile attack of the enzyme molecule toward the ester groups of the PLA chains.154 The hydrolytic degradation of the poly(lactic acid) (PLA)/TiO2 nanocomposites was investigated in phosphate buffer solution at pH 7.4 and 37 °C to elucidate the effect of nanofillers on the degradation of PLA for biomedical and environmental applications.155 The composite containing 20 wt% osteoconductive β-tricalcium phosphate and 80 wt% bioabsorbable poly-L,D-lactide (LL/DD dimer ratio of 96/4) was synthesized using a twin-screw extruder and the self-reinforcing technique. The presence of the β-tricalcium phosphate modified the degradation of the polymer matrix. The degradation rate of the self-reinforced composites was slower than the degradation rate of the self-reinforced polymer matrix because of the neutralization of the acidic degradation products of the polymer matrix by the alkaline degradation products of the β-TCP particles, therefore the autocatalytic effect of the polymer matrix was eliminated.156 Sobkowicz et al. have prepared clean and green bioplastic composites of PLA using two environmental friendly fillers, carbon nanospheres (CNS) derived from cellulose and calcium sulfate anhydrite (CaSO4). The CaSO4 is reported as a logical choice of filler for cost reduction as per the perspective of green chemistry as well as economics.157 However, among the various fillers, calcium carbonate (CaCO3) is used as an important reinforcing filler in the thermoplastic industry.158 PLA composites with precipitated calcium carbonate coated with stearic acid (PCC) and halloysite natural nanotubes (HNTs) were compared to study the crystallization behavior, mechanical properties and morphology of the composites. PCC nanoparticles showed a better nucleating effect which decreased both the glass transition and the cold crystallization temperatures. The morphological analysis of the fracture surface of the PLA nanocomposites showed a good dispersion of fillers, a larger plastic deformation and a formation of micro voids in the presence of PCC particles.159
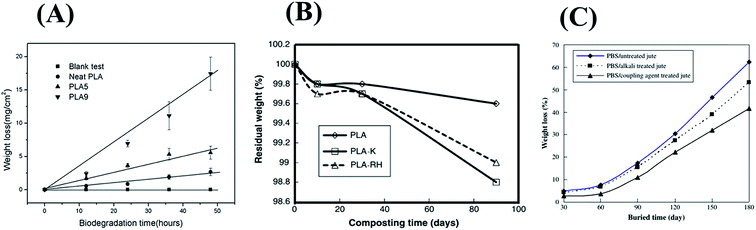 |
| Fig. 7 (A) Weight loss profile of neat PLA and PLA–silica nanocomposite films as function of time during enzymatic degradation (this figure has been reproduced from ref. 154 with permission from John Wiley and Sons); (B) relationship between the weight loss of PLA and its composites, namely PLA–kenaf (PLA–K) and PLA–ricehusk (PLA–RH) (this figure has been reproduced from ref. 162 with permission from Springer); (C) weight loss of PBS and composites with different surface modifications, namely PBS/untreated jute, PBS/alkali treated jute and PBS/coupling agent treated jute (this figure has been reproduced from ref. 165 with permission from Elsevier). | |
5.6.2 Plant derived fillers. The use of plant fibers as fillers to prepare composites of biodegradable polymers is very attractive because of their ample availability at low cost. In addition, natural fibers in composites enhanced the mechanical strength of the biopolymers and improved the thermal properties. It is good approach to substitute the existing petroleum based resources with natural plant derived materials. The composites of aliphatic polyesters like poly(ε-caprolactone) (PCL), poly(3-hydroxybutyrate-co-3-hydroxyvalerate) (PHBV), poly(butylene succinate) (PBS), and poly(lactic acid) (PLA) with 10 wt% untreated and acetic anhydride-treated (AA-) abaca fibers were prepared to investigate their biodegradability through the soil-burial test. The weight loss of the polymer matrices was found to follow the order PCL > PHBV > PBS > PLA.160 PLA showed good mechanical and thermal properties with an excellent biodegradability capacity with various natural fibers such as flax, jute, bamboo, banana fiber, kenaf and hemp. Okra (lady’s finger, Abelmoschus esculentus) fibers (5–10 mm) were also introduced into the PLA matrix to observe the disintegrability rate of PLA. The introduction of 10 and 20 wt% of both treated and untreated fibers increased the disintegrability rate of the PLA matrix due to the presence of hydroxyl groups existing in the cellulose structure that enhance the hydrolysis of the polyester groups. The disintegrability rate was more evident in the case of composites based on 10 and 20 wt% of both treated and untreated okra fibers after 20 days of incubation.161 Yussuf et al.162 compared the performances of poly(lactic acid) (PLA)/kenaf (PLA–K) and PLA/rice husk (PLA–RH) composites in terms of their biodegradability, and mechanical and thermal properties. Kenaf has a significant effect on the biodegradation rate as compared to rice husk after 90 days of incubation (Fig. 7B). The biodegradation of renewable resource-based biocomposites of PLA and various surface treated banana fibers using NaOH and various silanes viz. 3-aminopropyltriethoxysilane and bis-(3-triethoxy silyl propyl)tetrasulfane (Si69) was examined through morphological and weight loss studies in Burkholderia cepacia media.163 Poly(lactic acid) (PLA) and NPK fertilizer with empty fruit bunch (EFB) fibers were blended to produce bioplastic fertilizer (BpF) composites for the slow release of fertilizer. Fungal degradation studies indicated that increased fungi exposure times of BpF composites give higher values of degradation as compared to PLA/NPK.164 Jute is a lignocellulosic fiber with the highest specific strength and modulus and was used to prepare a PBS/jute composite. The weight loss of the PBS/jute composite was found to be higher than that of the pure PBS film and bulk jute fiber, and decreased with increasing fiber content and fiber diameter. The weight loss of the PBS/jute composite (10 wt%) was 62.5% after 180 days. Fiber surface modification also plays an important role towards biodegradation and the order of weight loss was found to be PBS/untreated jute > PBS/alkali treated jute > PBS/coupling agent treated jute as shown in Fig. 7C.165
5.7 Effect of filler dimension on biodegradation
Engineering of polymer composites is extensively investigated to improve chemical and physical properties and to regulate the biodegradability compared to that of the neat polymer. However, some of the polymer composites do not favor the biodegradability of the polymer after disposal. Therefore, research is focused on the design of biodegradable composites by incorporating nano-fillers of different dimensions within the polymer matrix. It is important to mention that nanofillers are preferred over their counterparts on the micro- or macro-scale because of their large specific surface area (surface area per unit volume).165 Composites with metal ions corresponding to zero dimensional fillers have shown remarkable biodegradability. The degradation rate of g-MgO/PLLA nanocomposites was found to increase gradually with the incorporation of surface modified magnesia (g-MgO) nanoparticles in pure PLLA (Fig. 8A). Addition of modified MgO nanoparticles improved the mechanical properties of the polymer enabling the adsorption of protein. The improved mechanical properties, faster biodegradation rate and protein adhesion behavior of the g-MgO/PLLA nanocomposites were advantageous for biomedical applications. The lactic acid resulting from the degradation of PLLA was neutralized effectively by the surface modified magnesia.166 Silica nanoparticles were found to be effective reinforcing fillers interacting with the reactive groups of the polymers either through hydrogen bonding or covalent bonds during the preparation of the polymer nanocomposites.167 The rate of enzymatic degradation of P(3HB-co-4HB) has enhanced significantly in nanocomposites of poly(3-hydroxybutyrate-co-4-hydroxybutyrate) [P(3HB-co-4HB)]/silica. Moreover, the silica was hydrophilic in nature resulting in an accelerated enzymatic degradation rate of P(3HB-co-4HB)/silica nanocomposites with increasing silica content due to a facile attack of the enzyme molecule toward the ester groups of the copolyester chains.168 The degradation due to weight loss of PCL with increasing content of Fe3O4 was investigated using gel fraction and molecular weight analysis. A complex system consisting of hydrophilic Fe3O4 and a hydrophobic polymeric matrix has a greater tendency to absorb the aqueous medium than the pure polymer. Thus, Fe3O4 can facilitate the infiltration of the degradation medium into the composite and promote its degradation.169 Thus, the increasing content and dispersion of zero dimension fillers in the composite accelerates the degradation of the polymer matrix. Further, the emergence of nanoscience and nanotechnology has expanded the exploration of one dimensional and two dimensional nano-fillers. One dimensional (CNT) and two dimensional (layered silicate and LDH) nano-fillers are to be defined based on the confinement of one or two axes, respectively, and exhibit intercalated or exfoliated patterns in nanocomposites. The nanoparticles have advantages over their micrometer size counterparts as better interactions can be achieved through the higher specific surface area of nanoparticles. The interfacial interaction between the matrix and the nanofiller improved the mechanical, thermal and biological properties of the neat polymer matrix as opposed to both micro- and macro-composites. The nature of the interaction between nanoclays and the polymer matrix depends on the organic modifiers which act as nucleating agents, and therefore control the dimension of the spherulite in the matrix.
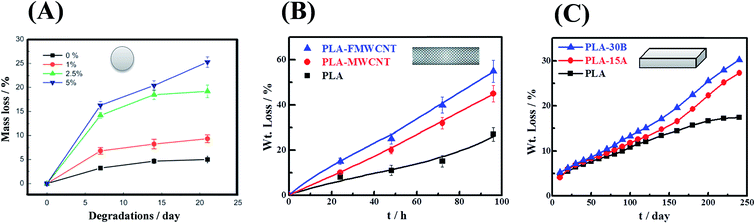 |
| Fig. 8 Comparison of biodegradation in the presence of filler with varying dimensions: (A) mass loss of PLLA/g-MgO nanocomposites vs. degradation time at pH 7.4 and 37 °C (this figure has been reproduced from ref. 166 with permission from Elsevier); (B) percentage weight loss of PLA and its indicated nanohybrids during enzymatic degradation (this figure has been reproduced from ref. 170 with permission from American Chemical Society); and (C) percentage weight loss of PLA and its indicated nanocomposites in compost media (this figure has been reproduced from ref. 58 with permission from John Wiley and Sons). | |
Carbon nanotubes (CNTs) are allotropes of carbon categorized as one dimensional nanoparticles and may act as excellent reinforcing fillers substituting conventional nanofillers in the fabrication of advanced multifunctional polymer nanohybrids. They are subcategorized into single-wall carbon nanotubes (SWCNTs), multi-wall carbon nanotubes (MWCNTs), double-wall carbon nanotubes (DWCNTs) and functionalized MWCNTs (FMWCNTs). The CNT-induced biodegradation, crystallization, and mechanical behavior of PLA were reported with excellent properties.170 The weight loss of PLA–MWCNTs and PLA–FMWCNTs was 45% and 55%, respectively, after 96 h of enzymatic degradation against a minimum weight loss of 25% observed for pure PLA (Fig. 8B). It is reported that two dimensional layered silicates have layered thicknesses of 1 nm and very high aspect ratios (50–1000) in addition to dipolar interactions between the –OH group of the organic modifier and the ester group of PLA. Polylactide (PLA)/organically modified layered silicate nanocomposites showed improved materials properties and crystallization behavior with a simultaneous improvement in biodegradability as compared to neat PLA.171 The changes in weight loss owing to the biodegradation in compost manure are quite slow for pristine PLA (maximum 17% weight loss in 240 days) while in the presence of nanoclays 15A and 30B, the rates of biodegradation were enhanced significantly to 27 and 31 wt% loss, respectively (Fig. 8C), in addition to the fact that organically modified surfaces can further induce the compatibility with the polymer matrix.58
6. Applications
6.1 Implantation and tissue engineering
Biodegradable polyesters are considered as bio-friendly materials. Therefore, they are being studied extensively in medical fields for tissue engineering, wound management, orthopedic and dental devices, cardiovascular and reconstructive surgery, and drug delivery systems. Biodegradable polymers simultaneously exhibit biological compatibility and degradability which allows them to be approved for practical use by the Food and Drug Administration.172 Therefore, the interest in biodegradable and biocompatible polymers and their composites has increased owing to their remarkable matching in mechanical strength and minimum risk of inflammatory complications. Poly(ε-caprolactone) (PCL)/poly(glycolic acid) (PGA) scaffolds exhibit optimal pore size for tissue engineering and chondrocyte growth. The compressive modulus of the scaffold matches the compressive modulus of human articular cartilage.173 A number of potential medical devices such as bioresorbable surgical sutures (Fig. 9A), biodegradable screws and plates (Fig. 9B), biodegradable membranes (Fig. 9C) and surgical meshes (Fig. 9D) using PHB have been developed for wound covering, cartilage and bone fixation, periodontal treatment and hernioplastic surgery, respectively.174 DePuy Synthes175 manufactured the ORTHOMESH resorbable graft containment system using 85
:
15 poly(L-lactide-co-glycolide) copolymers which retains approximately 85% of its initial bending strength after 8 weeks (Fig. 9E). The clinical advantages of this polymer were that it was biocompatible, bio-absorbable, and not affected by radiation therapy. This resorbable graft polymer is hydrolyzed into lactic acid and glycolic acid, which are metabolized in the form of water and CO2 without toxic tissue accumulation and it does not need any postoperative surgery.175 Meinig176 implanted resorbable microporous polylactide membranes (US based Synthes product) for the healing of a sheep’s tibia bone as shown in Fig. 9F.
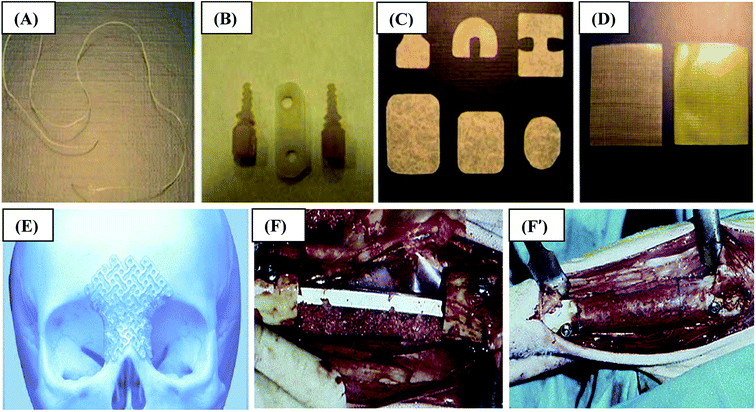 |
| Fig. 9 (A) Bioresorbable surgical suture, (B) biodegradable screws and plate for cartilage and bone fixation, (C) biodegradable membranes for periodontal treatment, (D) surgical meshes with PHB coating for hernioplastic surgery (this figure has been adapted from ref. 174 with permission from Formatex Research Center). (E) ORTHOMESH resorbable PLA membrane175 and (F) implantation of polylactide membranes (US based Synthes product) for the wound healing of a sheep’s tibia pre, and (F′) post-operative surgery (this figure has been adapted from ref. 176 with permission from Elsevier). | |
The effect of poly(lactic acid) (PLA) on the middle ear mucosa and cochlea meant that it could be employed as a film barrier for postoperative adhesion prevention in the middle ear of twenty-one albino guinea pigs and did not show any ototoxic side effects.177 A bifurcated scaffold is designed and constructed using a resorbable nanocomposite of polyhedral oligomeric silsesquioxane/poly(ε-caprolactone) urea urethane (POSS–PCL) demonstrating its potential as a tissue-engineered solution to paediatric tracheal replacement. Quantum dot imaging illustrates adequate cell penetration throughout the scaffold, which is further confirmed using scanning electron microscopy.178 Porous biodegradable polymer scaffolds are widely utilized for bone tissue engineering but are not osteoconductive like calcium phosphate scaffolds. Biomineral coatings in bone regeneration have been studied using poly(L-lactic acid) (PLLA) and poly(ε-caprolactone) (PCL) scaffolds and show significant fibrous tissue in coated scaffolds (both PLLA and PCL) as compared to uncoated scaffolds after ∼10 weeks.179 The scaffolds not only provide a physical support but also play a key role in processing the biological signals for cell differentiation and tissue regeneration. The 3D nanofiber-based scaffolds mimic the extracellular matrix of healthy bones. They support cell growth and offer great benefits for the promotion of cell breeding and osteoblast differentiation.180 A scaffold consisting of three-dimensional and porous networks of poly(L-lactic acid)/hydroxyapatite/bone morphogenetic protein-2 (PLLA/HA/BMP-2) has been designed, similar to the structure of the extracellular matrix of healthy bones, which promotes bone tissue formation.181 The bone losses due to trauma or disease often yield poor healing due to the complex anatomy and physiology of bone tissue. To restore the structure and function of bone wounds, a biodegradable scaffold system was designed to carry out osteogenic cell and growth factors which provide a platform to repair and regenerate damaged bone. Additionally, biomaterials are also degraded in a controlled manner without causing significant inflammatory responses.182 Poly(methyl methacrylate) (PMMA) based bone cement (BC) and its nanohybrid with the NK75 nanoclay (BC–NK75) material are compared in vivo in rabbit tibias and complete healing is seen in just 30 days using the nanohybrid against a healing time of 90 days for pure bone cement.183 However, polyesters, especially PLA, PCL and PBS, are good sources for making bio-scaffolds due to their processability, mechanical properties, biocompatibility, and consistent degradation and mineralization in an appropriate time frame. Three-arm stereo complex PLA-organic field effect transistors (tascPLA-OFETs) are designed for the demonstration of artificial skin-like temperature sensor arrays.172 The biocompatibility of the device has been examined through cell co-cultures and MTT assays to study the cytotoxicity of the tascPLA-OFETs. Poly(L-lactide) (PLLA) and poly(4-hydroxybutyrate) (P4HB) as potential coating matrices for cochlear implants (CI) have been used for the electrode–nerve interface due to their excellent coating adhesion/stability and optimal degradation behavior.184 Orozco et al.185 designed and fabricated multilayer assemblies of biodegradable self-reporting nanocomposite films of poly(lactic acid) nanoparticles by engineering a layer-by-layer assembly based on hydrogen-bonding or electrostatic interactions. The nanocomposite coatings of PLA nanoparticles are enzymatically degraded by α-chymotrypsin and these nanocomposites of ultra-thin PLA nanoparticle layered films are being employed in biomedical and bionanotechnology applications through real-time monitoring of biodegradation processes.
To facilitate the cell proliferation and to control the diffusion of biomolecules, the porosity and morphology of the scaffold has been manipulated as per the tissue texture. High porosity and pore interconnectivity are the key prerequisites to increase the specific surface area for cell attachment and tissue ingrowth that facilitate the uniform distribution of cells and the satisfactory mass transfer of nutrients and cellular waste products.186 Therefore, the rate of tissue ingrowth could be controlled by tuning the porosity and pore size of the scaffold by changing the polymer/solvent ratio. The biodegradation rate of micro and macro-structures can also be controlled by selecting different polymeric materials and processing parameters like crystallinity, and quenching and crystallization temperatures. Whang et al. regulated the porosity, pore size and specific pore area of the scaffold, depending on the processing parameters or by employing an emulsion freeze-drying technique. Scaffolds with a porosity greater than 90%, and medium pore sizes ranging from 15 to 35 μm with larger pores greater than 200 μm were fabricated.187 Highly porous biodegradable foams with the function of controlled release were also fabricated using a phase separation technique. Foams with pore sizes ranging from 20 to 500 μm of poly(L-lactic acid) (PLLA), poly(bisphenol A–phenylphosphonate) (BPA/PP) and its copolymer with poly[bis(2-ethoxy)-hydrophosphonic terephthalate] (PP/PPET) were synthesized to check the release study of sulforhodamine B and alkaline phosphatase. Alkaline phosphatase was released from the BPA/PP and PLLA foams at a steady rate of 0.32 ± 0.04 and 0.49 ± 0.13 mg per day per g, respectively.188 The controlled degradation of the PLA–PGA implant was reported in different pH media in the vicinity of degrading implants. Agrawal and Athanasiou189 controlled the pH near biodegradable PLA–PGA implants by using calcium carbonate (pH value between 7.4 and 6.3), calcium hydroxyapatite (pH values between 6.9 and 4.3) and sodium bicarbonate (pH values between 8.2 and 4.5) and concluded that basic salts prevent the decrease in pH near the implant site during degradation.189 Challenges in scaffold fabrication for tissue engineering are the surface functionalization and incorporation of biomolecules in their active form. Stankevich et al.190 modified the surface of high molecular weight PLA with a toluene/ethanol mixture and subsequent exposure in brilliant green dye (BGD) and performed the inflammatory response and biocompatibility assessments against CD14+ monocytes as a test system.190
6.2 Drug delivery
During the last few decades, many of the poorly controlled diseases such as cancer and diabetes have been treated using biodegradable polymeric nanoparticles via drug delivery systems which overcome the problems related to the stability and targeted release profile of the drugs. Bio-based polymeric nanoparticles have the potential to act as carriers of drugs at target sites by controlling their release rates and protecting them from the physiological environment.191 Polyesters are widely used as carriers for drug delivery systems due to their desirable biodegradable and biocompatible characteristics along with their approval with the Food and Drug Administration (FDA) for clinical use. The biocompatibility and cytotoxicity of PLA and its nanohybrids, PLA–MWCNT (multi-walled carbon nanotube), and PLA–FMWCNT (functionalized multi-walled carbon nanotube), were investigated using a MTT assay against platelets at different concentrations (2–100 μg mL−1). None of the polymer nanohybrids induced cell death, even when incubated at a concentration of 100 μg mL−1 and they were found to be well within the permissible limits. Furthermore, in terms of the RBC membrane integrity, the PLA based MWCNT/FMWCNT nanohybrids exhibited a hemolytic activity of less than 5%, even when the concentration was increased to 100 μg mL−1, so could be used as potential biomaterials for biomedical applications like biosensors, cell imaging and drug delivery. Apart from biocompatibility, the tuned biodegradation of PLA using MWCNTs/FMWCNTs was reported. A weight loss of 30 and 60% occurs for PLA–MWCNT and PLA–FMWCNT, respectively, and was reported against the weight loss of ∼25% for pure PLA after 96 h of enzymatic degradation. A higher degradation rate in the PLA–FMWCNT composite was due to a greater interaction between the PLA matrix and the FMWCNTs and a larger amorphous region.170 Quercetin (Qt) embedded poly(lactic acid) (PLA) nanoparticles (PLA–Qt) showed a sustained release of the drug revealing a novel vehicle for the treatment of cancer. Delayed diffusion and a stronger interaction in PLA–Qt caused the sustained delivery of Qt from the polymer matrix. In vitro cytotoxicity studies indicate the killing of ∼50% of the breast cancer cells in two days.191 PLA has no potential cytotoxic and genotoxic effects in the CHO-K1 cell line and does not disrupt the replicative cell homeostasis.176 The physicochemical, thermal and photoluminescence properties of PLA films were also evaluated before and after the contact with cells as well as their ability to absorb and release europium(III) complexes for further pharmaceutical purposes.192 For the control of anti-inflammatory drug loading and release, dexamethasone (DEX)-loaded poly(L-lactic acid) (PLLA) and poly(lactic-co-glycolic acid) (PLGA) nanofibers were deposited onto a functional nerve cuff electrode using the electrospinning method, which can control the weight of DEX loading on the functional nerve cuff electrode. DEX release rates have been found to increase from 16 to 28% (PLLA-loaded nanofibers) and from 68 to 87% (PLGA-loaded nanofibers) as observed using high performance liquid chromatography (HPLC) due to the increased diffusion rate of DEX after a certain time. The differential release rate is due to the lower molecular weight of PLGA than PLLA, along with the tendency to be degraded more easily.193 The subcutaneous injection of PEG–PLGA–PEG (550–2810–550) triblock copolymers into rats is shown in Fig. 10A and a 30% mass loss over the time of one month was revealed. The decrease in molecular weight from 3300 to 1900 is due to the preferential mass loss of PEG as examined via gel permeation chromatography.125 The co-assembly of an active targeting amphiphilic polymer e.g. cyclic (Arg-Gly-Asp-D-Phe-Lys) c(RGDfK) functionalized poly(ethylene oxide-b-ε-caprolactone) (cRGD-PEO-b-PCL), and a pH sensitive drug conjugate, e.g. benzoic-imine linked PEGylated doxorubicin (PEG–DOX) has been reported to provide combinatorial functions to the delivery system. PEG–DOX is cleavable at the extracellular pH of a solid tumor; the characteristics of the mixed micelles turn from “stealthy” to cancer cell-affinitive due to the detachment of PEG from the micelle surface and hence allow the interaction of c(RGDfK) in cRGD-PEO-b-PCL with the cancer cell membrane.194 Thus, taking the advantages of various pH environments and ionic strengths, polymer particles mediate the controlled delivery of drugs at a target site.
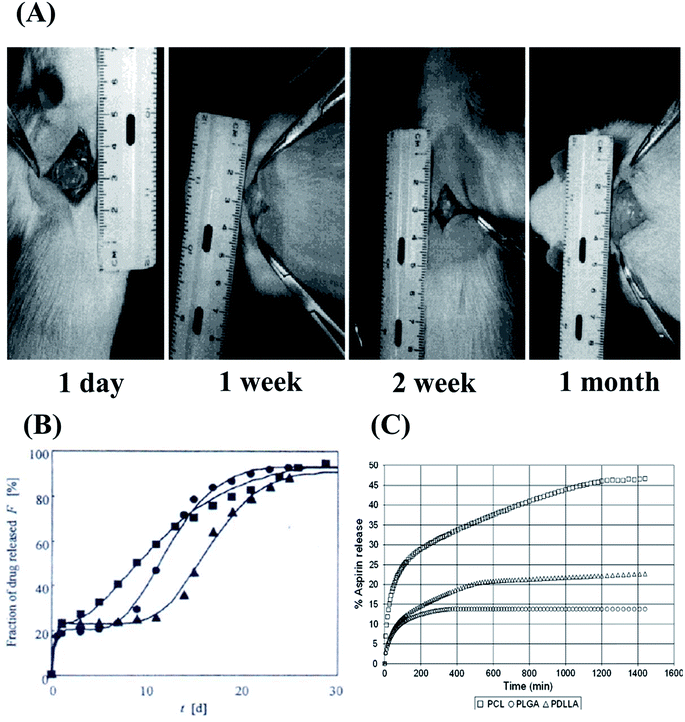 |
| Fig. 10 (A) The subcutaneous injection of the PEG–PLGA–PEG copolymer in rats showed a decrease in tumor size with time (this figure has been reproduced from ref. 125 with permission from John Wiley and Sons). (B) Comparison of the experimental release profiles of phosphorothioate oligo deoxynucleotide; the molecular weights of PLGA are (■) 10 000, (●) 15 000, (▲) 20 000 with a 75/25 ratio of PLA/PGA.202 (C) Drug release curves for thin film polymer drug matrices of PCL, PLGA and PDLLA (this figure has been reproduced from ref. 204 with permission from Springer). | |
Oral drug delivery is one of the preferred routes because of good patient compliance. However, most peptide/protein drugs are potentially not absorbed in the gastrointestinal tract because of poor enzymatic catalysis and low permeability across the biological membranes. To overcome these barriers, lipid based formulations and polymer-based particulate drug delivery systems have been proposed.195 A blend of biodegradable PCL and polycationic acrylic polymers in the form of nanoparticles are being used as drug carriers for the oral administration of insulin to diabetic rats. Nanoparticles of insulin–PCL increase serum insulin levels and improve the glycemic response to oral glucose for a prolonged period. Polymeric nanoparticles allow the preservation of insulin’s biological activity and the muco-adhesive properties of the polycationic polymer allow the intestinal uptake of insulin.196 A novel lovastatin (LVT)-loaded poly(lactic acid) microsphere suitable for oral administration has also been developed for in vitro and in vivo studies indicating well-controlled release efficacy using LVT-loaded PLA microspheres.197 The impact of nano-encapsulation of various disease related drugs using biodegradable polymers such as PLGA, PLA and PCL as drug delivery vehicles is highlighted with respect to the controlled release and improvement of the therapeutic value of nano-encapsulated drugs.198
The drug release profiles from biodegradable polymers were explored as a main tool to control the release rate of drugs in two ways: (i) diffusion kinetics: slow, continuous and sustainable diffusion of drugs over an extended duration; (ii) polymer erosion kinetics: burst release of drugs due to the erosion of the polymer surface.199,200 The above described drug delivery has to be explained on the basis of diffusion kinetics, in contrast to bulk erosion which takes place due to the cleavage of a polymeric bond after a certain period of time. A fraction of the drug incorporated in the polymer matrix is swiftly released from the surface of the polymer and then the rest of the drug in the polymer matrix is liberated due to the hydration of the biodegradable polymer i.e. a biphasic profile of drug release. Thus, the biodegradable polymers may cause remarkably high rates of drug release after some time due to the burst effect. Yamakawa et al.201 observed the biphasic release profile of phosphorothioate oligodeoxynucleotide from PLGA rods with molecular weights ranging from 10
000 to 20
000. To describe the biphasic profile of drug delivery, a model of a typical biphasic drug release phenomenon has been reported as an initial bursting release due to the dissolution of the surface drug molecules and the late bursting caused by the bulk erosion of the biodegradable polymers, known as the “dissolution-bulk erosion model of drug release from biodegradable polymer rods” (Fig. 10B).202 The rational design of biomedical devices is based on the surface-eroding phase-separation of polymers which requires a detailed understanding of the mechanisms of erosion.203 McDonald204 studied the drug diffusion and the reduction in the weight of the polymer to understand the mechanisms of drug delivery. PCL releases approximately 46.6% of the drug and the high level of drug release was accounted for during the burst period of the polymer matrices (Fig. 10C).
6.3 Food packaging
Currently, non-biodegradable plastics are commercially used for the packaging of beverages, biscuits, food, and medicines, and in agricultural sectors and are being disposed of without recycling. Therefore, substantial research efforts have been focused on the use of biodegradable plastics to reduce the environmental impact. PLA has been approved for its intended use in food packaging by the Food and Drug Administration (FDA). PLA-based packaging products for items like water, juice, and yogurts are widely used in the supermarkets of Europe and North America and meet the food standards, maintain aroma and freshness after processing and are also resistant to microbial stains. Therefore, PLA is gradually moving towards becoming a ‘green’ food packaging material for goods under different trade names such as Biota™, Noble™, Dannon™ etc.205 Kale et al.66 showed the biodegradation of a PLA bottle in compost media after 30 days of treatment (Fig. 11). Danone206 is the first company in Europe to replace packaging made from polystyrene with PLA for yogurt products (Fig. 12A). Food borne diseases have become an increasingly relevant health and safety concern in the food industry. In order to inhibit the growth of unwanted microbes on foods, antimicrobial packaging offers a means to potentially extend the shelf life of perishable foods to maintain quality. Qin et al.207 incorporated antimicrobial substances like cinnamaldehyde in poly(lactic acid)/poly(trimethylene carbonate) (PLA/PTMC) films to find out better ways to extend the shelf-life of foods. Biocompatible and biodegradable ε-poly-L-lysine (EPL)/poly(ε-caprolactone) (PCL) copolymers are made to self-assemble into monodispersed nanoparticles (NPs) which show a broad spectrum of antibacterial activity against Escherichia coli, Staphylococcus aureus and Bacillus subtilis.208 The self-assembled NPs induce changes in the bacterial osmotic pressure resulting in cell invagination to form holes and create a leakage of cytoplasm.208 The organically modified MMT (Cloisite 30B) exhibited antimicrobial activity against Gram-positive bacteria (Listeria monocytogenes and Staphylococcus aureus) presumably due to the quaternary ammonium group present in the modified organoclay, while the natural MMT did not show any such antimicrobial activity.209 Antimicrobial PCL-based bio-nanocomposites using quaternary ammonium modified montmorillonites (OMMTs) have been prepared for packaging.210 The performance of the composite films against Staphylococcus aureus (Gram positive) and Escherichia coli (Gram negative) have been evaluated from the number density of bacteria in the sample. When 7.5 log cells of E. coli O157:H7 were inoculated in orange juice, a significant reduction to 3.5 log units in the E. coli cell population was observed after 72 h when PLA/nisin was used whereas the control had decreased to about 6 log units. This suggests that nisin incorporation into PLA films was an effective inhibitor of E. coli O157:H7 in orange juice at a ratio of 2.08 mL of liquid per cm2 of exposed polymer surface. The agar diffusion test was investigated to monitor the antimicrobial activity of PLA/nisin films for solid food packaging. The antimicrobial activity of the film was expressed in terms of the inhibition zone (Fig. 12B). The formation of an inhibition zone around the PLA/nisin film showed potential for antimicrobial packaging in food applications.211
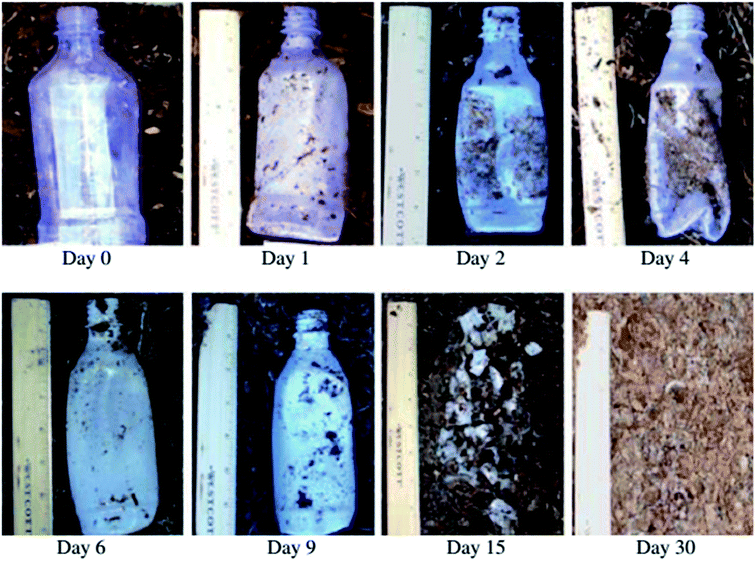 |
| Fig. 11 Pictorial view of PLA bottles exposed to 30 days of compost conditions (this figure has been adapted from ref. 66 with permission from Springer). | |
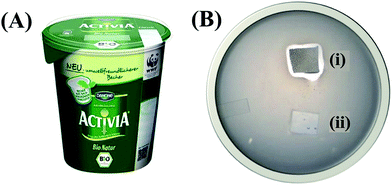 |
| Fig. 12 (A) PLA based yogurt packaging developed by Danone206 and (B) agar diffusion test of (i) PLA/nisin and (ii) PLA film against L. monocytogenes (this figure has been adapted from ref. 211 with permission from John Wiley and Sons). | |
Industrial uses of biopolymer-based packaging materials are limited owing to water sensitivity, brittleness, and poor mechanical and water vapor barrier properties. These intrinsic deficiencies are generally removed using nanotechnology by dispersing nanometer scale particles in the polymer matrix, forming so called ‘nanocomposites’.210,212 Poly(ethyleneimine) (PEI)/hexadecyl trimethyl ammonium bromide (CTAB)-modified graphene oxide (GO) layers on a PLA substrate increase the water contact angle of the multilayer PLA film and decrease the water vapor permeability significantly as compared to a pure PLA film of similar dimensions. CTAB modified GO enhances the barrier performance of the PLA films and raises the usability of coated PLA in packaging applications.213 The traditional approach towards barrier property enhancement of poly(lactic acid) is the incorporation of sheet-like fillers such as nanoclays and graphene with a trade-off in biocompatibility and degradability. In order to avoid these problems, the confined flaking technique has been employed to establish the degradable nanolaminar poly(butylene succinate) (PBS) on PLA films based on PLA/PBS in situ nanofibrillar composites.214 With the creation of compact polymer “nano-barrier walls”, a dramatic decrease (∼86%) of oxygen permeability is observed for the PLA/PBS (80/20) film as compared to pure PLA in addition to the increase in tensile strength and stiffness of the composite films. The unusual combination of barrier and mechanical performances establish a fully degradable system which represents specific properties required for the green packaging of beverages, food and medicines. The addition of PHB strongly increases the oxygen barrier while decreasing the wettability of PLA and the blends are being used for food packaging.215 Thus, biodegradable and renewable polyesters play a decisive role in the preservation and packaging of all types of food particularly from oxidative and microbial spoilage which in turn extends the shelf life of the food products.
7. Conclusion
In this review, we have summarized the need for, the advantages and the challenges of biodegradable polymers for green applications. The classification of biopolymers and biodegradable polymers has been emphasized and light has been shed on the role of individual biodegradable polymers in their typical applications. Different types of degradation have been discussed with their appropriate reasons and particular mechanisms. The rate of biodegradation in different media (compost, microbes and enzyme) has been compared and the tuning of the biodegradation rate (both increased and decreased with respect to pure polymer degradation) has been illustrated using organically modified layered silicate or carbon nanosheets. Enzymatic degradation has been studied under varying environments to elucidate the activation of enzymes at different conditions. Finally, the exploitation of biodegradable and biocompatible polymers along with their nanohybrids in tissue engineering, implants, drug delivery and packaging has been reported.
Acknowledgements
The author (S. Kumar) gratefully acknowledges the financial support from Council for Scientific and Industrial Research (CSIR), New Delhi, in the form of a CSIR (RA) fellowship. The authors also acknowledge the receipt of research funding from Science and Engineering Research Board (SERB), New Delhi, Government of India (Project No. EMR/2015/001409).
References
- S. Chanprateep, Current trends in biodegradable polyhydroxyalkanoates, J. Biosci. Bioeng., 2010, 110, 621–632 CrossRef CAS PubMed.
- Y. Wu, G. Wang, Z. Wang, Y. Liu, P. Gu and D. Sun, Comparative study on the efficiency and environmental impact of two methods of utilizing polyvinyl chloride waste based on life cycle assessments, Front. Environ. Sci. Eng., 2014, 8, 451–462 CrossRef CAS.
- K. M. Nampoothiri, N. R. Nair and R. P. John, An overview of the recent developments in polylactide (PLA) research, Bioresour. Technol., 2010, 101, 8493–8501 CrossRef PubMed.
- US EPA, 2007 data http://www.sustainableplastics.org/problems/plastics-recycling-remains-low.
- A. Esmaeili, A. A. Pourbabaee, H. A. Alikhani, F. Shabani and E. Esmaeili, Biodegradation of low-density polyethylene (LDPE) by mixed culture of Lysinibacillus xylanilyticus and Aspergillus niger in soil, PLoS One, 2013, 8(9), e71720 CAS.
- T. F. Ojeda, E. Dalmolin, M. M. Forte, R. J. Jacques, F. M. Bento and F. A. Camargo, Abiotic and biotic degradation of oxo-biodegradable polyethylenes, Polym. Degrad. Stab., 2009, 94(6), 965–970 CrossRef CAS.
- D. Y. Bang, M. Kyung, M. J. Kim, B. Y. Jung, M. C. Cho and S. M. Choi, et al., Human Risk Assessment of Endocrine-Disrupting Chemicals Derived from Plastic Food Containers, Compr. Rev. Food Sci. Food Saf., 2012, 11, 453–470 CrossRef CAS.
- E. Dare, R. Tofighi, L. Nutt, M. Vettori, M. Emgård and A. Mutti, et al., Styrene 7, 8-oxide induces mitochondrial damage and oxidative stress in neurons, Toxicology, 2004, 201, 125–132 CrossRef CAS PubMed.
- M. Houde, M. Douville, P. Gagnon, J. Sproull and F. Cloutier, Exposure of Daphnia magna to trichloroethylene (TCE) and vinyl chloride (VC): evaluation of gene transcription, cellular activity, and life-history parameters, Ecotoxicol. Environ. Saf., 2015, 116, 10–18 CrossRef CAS PubMed.
- M. L. North, T. K. Takaro, M. L. Diamond and A. K. Ellis, Effects of phthalates on the development and expression of allergic disease and asthma, Ann. Allergy, Asthma, Immunol., 2014, 112, 496–502 CrossRef CAS PubMed.
- European Bioplastics, Institute for Bioplastics and Biocomposites, nova-Institute (2014) http://www.corbion.com/media/203221/eubp_factsfigures_bioplastics_2013.pdf.
- M. Funabashi, F. Ninomiya and M. Kunioka, Biodegradability evaluation of polymers by ISO 14855-2, Int. J. Mol. Sci., 2009, 10, 3635–3654 CrossRef CAS PubMed.
- Japan BioPlastics Association http://www.jbpaweb.net/english/english.htm.
- H.-A. Lim, T. Raku and Y. Tokiwa, Hydrolysis of polyesters by serine proteases, Biotechnol. Lett., 2005, 27, 459–464 CrossRef CAS PubMed.
- P. L. Bishop, Pollution prevention: fundamentals and practice, McGraw-Hill, Boston, Mass, 2000 Search PubMed.
- F. Giudice, G. La Rosa and A. Risitano, Product design for the environment: a life cycle approach, CRC press, 2006 Search PubMed.
- M. D. Tabone, J. J. Cregg, E. J. Beckman and A. E. Landis, Sustainability metrics: life cycle assessment and green design in polymers, Environ. Sci. Technol., 2010, 44, 8264–8269 CrossRef CAS PubMed.
- S. Kim and B. E. Dale, Energy and greenhouse gas profiles of polyhydroxybutyrates derived from corn grain: a life cycle perspective, Environ. Sci. Technol., 2008, 42, 7690–7695 CrossRef CAS PubMed.
- K. Fukushima, D. Tabuani, M. Dottori, I. Armentano, J. Kenny and G. Camino, Effect of temperature and nanoparticle type on hydrolytic degradation of poly(lactic acid) nanocomposites, Polym. Degrad. Stab., 2011, 96, 2120–2129 CrossRef CAS.
- R. M. Rasal, A. V. Janorkar and D. E. Hirt, Poly(lactic acid) modifications, Prog. Polym. Sci., 2010, 35, 338–356 CrossRef CAS.
- R. P. John, K. M. Nampoothiri and A. Pandey, Solid-state fermentation for L-lactic acid production from agro wastes using Lactobacillus delbrueckii, Process Biochem., 2006, 41, 759–763 CrossRef CAS.
- L.-T. Lim, R. Auras and M. Rubino, Processing technologies for poly(lactic acid), Prog. Polym. Sci., 2008, 33, 820–852 CrossRef CAS.
- A. P. Kumar, D. Depan, N. S. Tomer and R. P. Singh, Nanoscale particles for polymer degradation and stabilization—trends and future perspectives, Prog. Polym. Sci., 2009, 34, 479–515 CrossRef CAS.
- K. Fukushima, D. Tabuani and G. Camino, Nanocomposites of PLA and PCL based on montmorillonite and sepiolite, Mater. Sci. Eng. C, 2009, 29, 1433–1441 CrossRef CAS.
- K. Hamad, M. Kaseem, Y. G. Ko and F. Deri, Biodegradable polymer blends and composites: an overview, Polym. Sci., Ser. A, 2014, 56, 812–829 CrossRef CAS.
- B. S. Kim and H. N. Chang, Production of poly(3-hydroxybutyrate) from starch by Azotobacter chroococcum, Biotechnol. Lett., 1998, 20, 109–112 CrossRef CAS.
- L. Bora, Polyhydroxybutyrate accumulation in Bacillus megaterium and optimization of process parameters using response surface methodology, J. Polym. Environ., 2013, 21, 415–420 CrossRef CAS.
- L. S. Nair and C. T. Laurencin, Biodegradable polymers as biomaterials, Prog. Polym. Sci., 2007, 32(8), 762–798 CrossRef CAS.
- http://www.bpf.co.uk/plastipedia/polymers/polymer-bio-based-degradables.aspx.
- T. Iwata, Biodegradable and Bio-Based Polymers: Future Prospects of Eco-Friendly Plastics, Angew. Chem., Int. Ed., 2015, 54(11), 3210–3215 CrossRef CAS PubMed.
- J. M. Lagaron and A. Lopez-Rubio, Nanotechnology for bioplastics: opportunities, challenges and strategies, Trends Food Sci. Technol., 2011, 22(11), 611–617 CrossRef CAS.
- http://www.plastice.org/fileadmin/files/biobased.pdf.
- A. K. Mohanty, M. Misra and G. Hinrichsen, Biofibres, biodegradable polymers and biocomposites: an overview, Macromol. Mater. Eng., 2000, 276(1), 1–24 CrossRef.
- L. Fernández-Espada, C. Bengoechea, F. Cordobés and A. Guerrero, Thermomechanical properties and water uptake capacity of soy protein-based bioplastics processed by injection molding, J. Appl. Polym. Sci., 2016, 133(24) DOI:10.1002/app.43524.
- V. Perez-Puyana, M. Felix, A. Romero and A. Guerrero, Effect of the injection moulding processing conditions on the development of pea protein-based bioplastics, J. Appl. Polym. Sci., 2016, 133(20) DOI:10.1002/app.43306.
- http://www.appg-agscience.org.uk/linkedfiles/HGCABioplastics_web28409.pdf.
- M. Finkelstein and B. H. Davison, Applied biochemistry and biotechnology, Spring, 1998, vol. 70–72, ISSN 0273–2289 Search PubMed.
- T. Keshavarz and I. Roy, Polyhydroxyalkanoates: bioplastics with a green agenda, Curr. Opin. Microbiol., 2010, 13(3), 321–326 CrossRef CAS PubMed.
- K. Amulya, M. V. Reddy, M. V. Rohit and S. V. Mohan, Wastewater as renewable feedstock for bioplastics production: understanding the role of reactor microenvironment and system pH, J. Cleaner Prod., 2016, 112, 4618–4627 CrossRef CAS.
- J. K. Pandey, K. R. Reddy, A. P. Kumar and R. Singh, An overview on the degradability of polymer nanocomposites, Polym. Degrad. Stab., 2005, 88, 234–250 CrossRef CAS.
- D. Adamcova and M. Vaverkova, Biodegradation of degradable/biodegradable plastic material in controlled composting environment, Pol. J. Environ. Stud., 2014, 23, 1465–1474 Search PubMed.
- B. Fang, H. Kang, R. Wang, Z. Wang, W. Wang and L. Zhang, Aging behavior and mechanism of bio-based engineering polyester elastomer nanocomposites, J. Appl. Polym. Sci., 2014, 131 DOI:10.1002/app.40862.
- P. Scarfato, D. Acierno and P. Russo, Photooxidative weathering of biodegradable nanocomposite films containing halloysite, Polym. Compos., 2015, 36, 1169–1175 CrossRef CAS.
- S. Commereuc, H. Askanian, V. Verney, A. Celli, P. Marchese and C. Berti, About the end life of novel aliphatic and aliphatic–aromatic (co) polyesters after UV-weathering: structure/degradability relationships, Polym. Degrad. Stab., 2013, 98, 1321–1328 CrossRef CAS.
- P. Stloukal, V. Verney, S. Commereuc, J. Rychly, L. Matisova-Rychlá and V. Pis, et al., Assessment of the interrelation between photooxidation and biodegradation of selected polyesters after artificial weathering, Chemosphere, 2012, 88, 1214–1219 CrossRef CAS PubMed.
- J. Sahari, S. M. Sapuan, E. S. Zainudin and M. Maleque, Degradation characteristics of SPF/SPS biocomposites, Fibres Text. East. Eur., 2014, 22, 94–96 Search PubMed.
- A. Hoshino, H. Sawada, M. Yokota, M. Tsuji, K. Fukuda and M. Kimura, Influence of weather conditions and soil properties on degradation of biodegradable plastics in soil, Soil Sci. Plant Nutr., 2001, 47, 35–43 CrossRef.
- E. Yousif and R. Haddad, Photodegradation and photostabilization of polymers, especially polystyrene: review, SpringerPlus, 2013, 2, 398 CrossRef PubMed.
- J. F. Rabek and B. Ranby, Role of singlet oxygen in photo-oxidative degradation and photostabilization of polymers, Polym. Eng. Sci., 2004, 15, 40–43 Search PubMed.
- S. H. Hyon, K. Jamshidi and Y. Ikada, Polymers as biomaterials, Plenum, New York, 1984, pp. 51–65 Search PubMed.
- M. Itavaara, S. Karjomaa and J. F. Selin, Biodegradation of polylactide in aerobic and anaerobic thermophilic conditions, Chemosphere, 2002, 46, 879–885 CrossRef CAS PubMed.
- C. M. Agrawal, D. Huang, J. P. Schmitz and K. A. Athanasiou, Elevated temperature degradation of a 50
:
50 copolymer of PLA–PGA, Tissue Eng., 1997, 3(4), 345–352 CrossRef CAS. - N. A. Weir, F. J. Buchanan, J. F. Orr, D. F. Farrar and G. R. Dickson, Degradation of poly-L lactide. Part 2: increased temperature accelerated degradation, Proc. Inst. Mech. Eng., Part H, 2004, 218(5), 321–330 CrossRef CAS.
- F.-D. Kopinke, M. Remmler, K. Mackenzie, M. Möder and O. Wachsen, Thermal decomposition of biodegradable polyesters—II.Poly(lactic acid), Polym. Degrad. Stab., 1996, 53, 329–342 CrossRef CAS.
- F. Carrasco, P. Pagès, J. Gámez-Pérez, O. Santana and M. L. Maspoch, Processing of poly(lactic acid): characterization of chemical structure, thermal stability and mechanical properties, Polym. Degrad. Stab., 2010, 95, 116–125 CrossRef CAS.
- S. Li and S. McCarthy, Further investigations on the hydrolytic degradation of poly(DL-lactide), Biomaterials, 1999, 20(1), 35–44 CrossRef CAS PubMed.
- K. Fukushima, C. Abbate, D. Tabuani, M. Gennari and G. Camino, Biodegradation of poly(lactic acid) and its nanocomposites, Polym. Degrad. Stab., 2009, 94, 1646–1655 CrossRef CAS.
- N. K. Singh, B. P. D. Purkayastha, M. Panigrahi, R. K. Gautam, R. M. Banik and P. Maiti, Enzymatic degradation of polylactide/layered silicate nanocomposites: effect of organic modifiers, J. Appl. Polym. Sci., 2013, 127, 2465–2474 CrossRef CAS.
- S. De Jong, E. R. Arias, D. Rijkers, C. Van Nostrum, J. Kettenes-Van den Bosch and W. Hennink, New insights into the hydrolytic degradation of poly(lactic acid): participation of the alcohol terminus, Polymer, 2001, 42, 2795–2802 CrossRef CAS.
- N. Lucas, C. Bienaime, C. Belloy, M. Queneudec, F. Silvestre and J.-E. Nava-Saucedo, Polymer biodegradation: mechanisms and estimation techniques–a review, Chemosphere, 2008, 73, 429–442 CrossRef CAS PubMed.
- S. Dopico-García, A. Ares-Pernas, J. Otero-Canabal, M. Castro-López, J. M. López-Vilariño and V. González-Rodríguez, et al., Insight into industrial PLA aging process by complementary use of rheology, HPLC, and MALDI, Polym. Adv. Technol., 2013, 24, 723–731 CrossRef.
- N. K. Singh, B. P. D. Purkayastha, J. K. Roy, R. M. Banik, P. Gonugunta and M. Misra, et al., Tuned biodegradation using poly(hydroxybutyrate-co-valerate) nanobiohybrids: emerging biomaterials for tissue engineering and drug delivery, J. Mater. Chem., 2011, 21, 15919–15927 RSC.
- M. Hakkarainen, S. Karlsson and A. C. Albertsson, Influence of low molecular weight lactic acid derivatives on degradability of polylactide, J. Appl. Polym. Sci., 2000, 76, 228–239 CrossRef CAS.
- A. Torres, S. Li, S. Roussos and M. Vert, Degradation of L- and DL-lactic acid oligomers in the presence of Fusarium moniliforme and Pseudomonas putida, J. Environ. Polym. Degrad., 1996, 4, 213–223 CrossRef CAS.
- F. Codari, D. Moscatelli, G. Storti and M. Morbidelli, Characterization of Low-Molecular-Weight PLA using HPLC, Macromol. Mater. Eng., 2010, 295, 58–66 CrossRef CAS.
- G. Kale, R. Auras and S. P. Singh, Degradation of commercial biodegradable packages under real composting and ambient exposure conditions, J. Polym. Environ., 2006, 14, 317–334 CrossRef CAS.
- G. Kale, R. Auras and S. P. Singh, Comparison of the degradability of poly(lactide) packages in composting and ambient exposure conditions, Packag. Technol. Sci., 2007, 20, 49–70 CrossRef CAS.
- W. Sikorska, M. Musiol, B. Nowak, J. Pajak, S. Labuzek and M. Kowalczuk, et al., Degradability of polylactide and its blend with poly[(R,S)-3-hydroxybutyrate] in industrial composting and compost extract, Int. Biodeterior. Biodegrad., 2015, 101, 32–41 CrossRef CAS.
- A. A. Shah, F. Hasan, A. Hameed and S. Ahmed, Biological degradation of plastics: a comprehensive review, Biotechnol. Adv., 2008, 26, 246–265 CrossRef CAS PubMed.
- P. Maiti and J. P. Prakash Yadav, Biodegradable nanocomposites of poly(hydroxybutyrate-co-hydroxyvalerate): the effect of nanoparticles, J. Nanosci. Nanotechnol., 2008, 8, 1858–1866 CrossRef CAS PubMed.
- H. Mao, H. Liu, Z. Gao, T. Su and Z. Wang, Biodegradation of Poly(butylene succinate)by Fusarium sp. FS1301 and Purification and Characterization of Poly(butylene succinate) Depolymerase, Polym. Degrad. Stab., 2015, 114, 1–7 CrossRef CAS.
- H. Pranamuda and Y. Tokiwa, Degradation of poly(L-lactide) by strains belonging to genus Amycolatopsis, Biotechnol. Lett., 1999, 21, 901–905 CrossRef CAS.
- Y. Tokiwa and B. P. Calabia, Biodegradability and biodegradation of poly(lactide), Appl. Microbiol. Biotechnol., 2006, 72, 244–251 CrossRef CAS PubMed.
- M. N. Kim and S. T. Park, Degradation of poly(L-lactide) by a mesophilic bacterium, J. Appl. Polym. Sci., 2010, 117, 67–74 CAS.
- A. A. Sha, A. Nawaz, L. Kanwal, F. Hasan, S. Khan and M. Badshah, Degradation of poly(ε-caprolactone) by a thermophilic bacterium Ralstonia sp. strain MRL-TL isolated from hot spring, Int. Biodeterior. Biodegrad., 2015, 98, 35–42 CrossRef.
- S. Li, M. Tenon, H. Garreau, C. Braud and M. Vert, Enzymatic degradation of stereocopolymers derived from L-, DL- and meso-lactides, Polym. Degrad. Stab., 2000, 67, 85–90 CrossRef CAS.
- Y. A. Shigeno, T. Teeraphatpornchai, K. Teamtisong, N. Nomura, H. Uchiyama, T. Nakahara and T. N. Kambe, Cloning and sequencing of a poly(DL-lactic acid) depolymerase gene from Paenibacillus amylolyticus strain TB-13 and its functional expression in Escherichia coli, Appl. Environ. Microbiol., 2003, 69, 2498–2504 CrossRef.
- B. Eker, P. Asuri, S. Murugesan, R. J. Linhardt and J. S. Dordick, Enzyme–carbon nanotube conjugates in room-temperature ionic liquids, Appl. Biochem.
Biotechnol., 2007, 143, 153–163 CrossRef CAS PubMed.
- A. Röttig and A. Steinbüchel, Acyltransferases in bacteria, Microbiol. Mol. Biol. Rev., 2013, 77, 277–321 CrossRef PubMed.
- A. Sugihara, M. Ueshima, Y. Shimada, S. Tsunasawa and Y. Tominaga, Purification and characterization of a novel thermostable lipase from Pseudomonas cepacia, J. Biochem., 1992, 112, 598–603 CAS.
- N. K. Singh, B. D. Purkayastha, J. K. Roy, R. M. Banik, M. Yashpal and G. Singh, et al., Nanoparticle-induced controlled biodegradation and its mechanism in poly(ε-caprolactone), ACS Appl. Mater. Interfaces, 2009, 2, 69–81 Search PubMed.
- S. Kumar and P. Maiti, Understanding the controlled biodegradation of polymers using nanoclays, Polymer, 2015, 76, 25–33 CrossRef CAS.
- P. J. Baker, C. Poultney, Z. Liu, R. Gross and J. K. Montclare, Identification and comparison of cutinases for synthetic polyester degradation, Appl. Microbiol. Biotechnol., 2012, 93, 229–240 CrossRef PubMed.
- K. S. Shashidhara and S. M. Gaikwad, Conformational and functional transcription in class II α-mannoside from Aspergillus fischeri, J. Fluoresc., 2010, 20, 827–836 CrossRef CAS PubMed.
- C. T. Li, M. Zhang, J. X. Qin, Y. Zhang and J. H. Qiu, Study on molecular modeling and the difference of PC lipase-catalyzed degradation of poly(butylene succinate) copolymers modified by linear monomers, Polym. Degrad. Stab., 2015, 116, 75–80 CrossRef CAS.
- M. Ding, M. Zhang, J. Yang and J. H. Qiu, Study on the enzymatic degradation of aliphatic polyester–PBS and its copolymers, J. Appl. Polym. Sci., 2012, 124, 2902–2907 CrossRef CAS.
- K. Park and M. Xanthos, A study on the degradation of polylactic acid in the presence of phosphonium ionic liquids, Polym. Degrad. Stab., 2009, 94, 834–844 CrossRef CAS.
- M. A. Paul, C. Delcourt, M. Alexandre, P. Degée, F. Monteverde and P. Dubois, Polylactide/montmorillonite nanocomposites: study of the hydrolytic degradation, Polym. Degrad. Stab., 2005, 87, 535–542 CrossRef CAS.
- Y. Tokiwa, B. P. Calabia, C. U. Ugwu and S. Aiba, Biodegradability of plastics, Int. J. Mol. Sci., 2009, 10, 3722–3742 CrossRef CAS PubMed.
- S. K. Lee, D. G. Seong and J. R. Youn, Degradation and rheological properties of biodegradable nanocomposites prepared by melt intercalation method, Fibers Polym., 2005, 6, 289–296 CrossRef CAS.
- N. Grassie and G. Scott, Polymer degradation and stabilisation, CUP Archive, 1988 Search PubMed.
- R. B. Seymour and C. E. Carraher, Polymer chemistry, Marcel Dekker, 2003 Search PubMed.
- J. P. Santerre, R. S. Labow and G. A. Adams, Enzyme–biomaterial interactions: effect of biosystems on degradation of polyurethanes, J. Biomed. Mater. Res., 1993, 27(1), 97–109 CrossRef CAS PubMed.
- K. Stockhofe, J. M. Postema, H. Schieferstein and T. L. Ross, Radiolabeling of nanoparticles and polymers for PET imaging, Pharmaceuticals, 2014, 7(4), 392–418 CrossRef CAS PubMed.
- V. Massardier-Nageotte, C. Pestre, T. Cruard-Pradet and R. Bayard, Aerobic and anaerobic biodegradability of polymer films and physico-chemical characterization, Polym. Degrad. Stab., 2006, 91(3), 620–627 CrossRef CAS.
- K. I. Kasuya, K. I. Takagi, S. I. Ishiwatari, Y. Yoshida and Y. Doi, Biodegradabilities of various aliphatic polyesters in natural waters, Polym. Degrad. Stab., 1998, 59(1), 327–332 CrossRef CAS.
- M. R. Calil, F. Gaboardi, C. G. Guedes and D. S. Rosa, Comparison of the biodegradation of poly(ε-caprolactone), cellulose acetate and their blends by the Sturm test and selected cultured fungi, Polym. Test., 2006, 25(5), 597–604 CrossRef CAS.
- A. Calmon, L. Dusserre-Bresson, V. Bellon-Maurel, P. Feuilloley and F. Silvestre, An automated test for measuring polymer biodegradation, Chemosphere, 2000, 41(5), 645–651 CrossRef CAS PubMed.
- B. S. Zolnik and D. J. Burgess, Effect of acidic pH on PLGA microsphere degradation and release, J. Controlled Release, 2007, 122(3), 338–344 CrossRef CAS PubMed.
- I. K. Dimzon, X. Trier, T. Frömel, R. Helmus, T. P. Knepper and P. de Voogt, High Resolution Mass Spectrometry of Polyfluorinated Polyether-Based Formulation, J. Am. Soc. Mass Spectrom., 2016, 27(2), 309–318 CrossRef CAS PubMed.
- P. Giunchedi, B. Conti, S. Scalia and U. Conte, In vitro degradation study of polyester microspheres by a new HPLC method for monomer release determination, J. Controlled Release, 1998, 56(1), 53–62 CrossRef CAS PubMed.
- G. Schliecker, C. Schmidt, S. Fuchs, R. Wombacher and T. Kissel, Hydrolytic degradation of poly(lactide-co-glycolide) films: effect of oligomers on degradation rate and crystallinity, Int. J. Pharm., 2003, 266(1), 39–49 CrossRef CAS PubMed.
- S. Li and S. McCarthy, Further investigations on the hydrolytic degradation of poly(DL-lactide), Biomaterials, 1999, 20(1), 35–44 CrossRef CAS PubMed.
- C. A. Santos, B. D. Freedman, K. J. Leach, D. L. Press, M. Scarpulla and E. Mathiowitz, Poly(fumaric-co-sebacic anhydride): a degradation study as evaluated by FTIR, DSC, GPC and X-ray diffraction, J. Controlled Release, 1999, 60(1), 11–22 CrossRef CAS PubMed.
- S. Wang, C. Song, G. Chen, T. Guo, J. Liu, B. Zhang and S. Takeuchi, Characteristics and biodegradation properties of poly(3-hydroxybutyrate-co-3-hydroxyvalerate)/organophilic montmorillonite (PHBV/OMMT) nanocomposite, Polym. Degrad. Stab., 2005, 87(1), 69–76 CrossRef CAS.
- J. Fabia, J. Janicki, T. Graczyk, P. Dobrzyński and J. Kasperczyk, DSC, WAXD and SEM studies of biodegradation of poly(L-lactide–glycolide–trimethylenecarbonate) shape memory terpolymer, J. Therm. Anal. Calorim., 2013, 113(1), 413–417 CrossRef CAS.
- H. S. Azevedo, F. M. Gama and R. L. Reis, In vitro assessment of the enzymatic degradation of several starch based biomaterials, Biomacromolecules, 2003, 4(6), 1703–1712 CrossRef CAS PubMed.
- V. Tserki, P. Matzinos, E. Pavlidou, D. Vachliotis and C. Panayiotou, Biodegradable aliphatic polyesters. Part I. Properties and biodegradation of poly(butylene succinate-co-butylene adipate), Polym. Degrad. Stab., 2006, 91(2), 367–376 CrossRef CAS.
- J. Panyam, M. M. Dali, S. K. Sahoo, W. Ma, S. S. Chakravarthi, G. L. Amidon, R. J. Levy and V. Labhasetwar, Polymer degradation and in vitro release of a model protein from poly(D,L-lactide-co-glycolide) nano- and microparticles, J. Controlled Release, 2003, 92(1), 173–187 CrossRef CAS PubMed.
- M. Kowalczuk and G. Adamus, Mass spectrometry for the elucidation of the subtle molecular structure of biodegradable polymers and their degradation products, Mass Spectrom. Rev., 2016, 35(1), 188–198 CrossRef CAS PubMed.
- K. Mäder, Y. Crémmilleux, A. J. Domb, J. R. Dunn and H. M. Swartz, In vitro/in vivo comparison of drug release and polymer erosion from biodegradable P(FAD–SA) polyanhydrides—a noninvasive approach by the combined use of electron paramagnetic resonance spectroscopy and nuclear magnetic resonance imaging, Pharm. Res., 1997, 14(6), 820–826 CrossRef.
- G. L. Woo, M. W. Mittelman and J. P. Santerre, Synthesis and characterization of a novel biodegradable antimicrobial polymer, Biomaterials, 2000, 21(12), 1235–1246 CrossRef CAS PubMed.
- A. E. Somers, T. J. Bastow, M. I. Burgar, M. Forsyth and A. J. Hill, Quantifying rubber degradation using NMR, Polym. Degrad. Stab., 2000, 70(1), 31–37 CrossRef CAS.
- A. J. Domb, M. Rock, J. Schwartz, C. Perkin, G. Yipchuk, B. Broxup and J. G. Villemure, Metabolic disposition and elimination studies of a radiolabelled biodegradable polymeric implant in the rat brain, Biomaterials, 1994, 15(9), 681–688 CrossRef CAS PubMed.
- J. R. Wolf, Review: radiolabeled polymers containing covalently bound 3H and 14C, J. Labelled Compd. Radiopharm., 2016, 59, 38–47 CrossRef CAS PubMed.
- A. Sodergard and M. Stold, Properties of lactic acid based polymers and their correlation with composition, Prog. Mater. Sci., 2002, 27, 1123–1163 CAS.
- G. Kale, R. Auras, S. P. Singh and R. Narayan, Biodegradability of polylactide bottles in real and simulated composting conditions, Polym. Test., 2007, 26, 1049–1061 CrossRef CAS.
- J. Meng, H. Li, Y. Gao, H. Xu, H. Gu and J. Chang, Application of hydrophobic coatings in biodegradable devices, Bio-Med. Mater. Eng., 2015, 25, 77–88 CAS.
- H. Tsuji, Y. Kidokoro and M. Mochizuki, Enzymatic Degradation of Biodegradable Polyester Composites of Poly(L-lactic acid) and Poly(ε-caprolactone), Macromol. Mater. Eng., 2006, 291, 1245–1254 CrossRef CAS.
- R. M. Felfel, K. M. Z. Hossain, A. J. Parsons, C. D. Rudd and I. Ahmed, Accelerated in vitro degradation properties of polylactic acid/phosphate glass fibre composites, J. Mater. Sci., 2015, 50, 3942–3955 CrossRef CAS.
- M. Panigrahi, N. K. Singh, R. K. Gautam, R. M. Banik and P. Maiti, Improved biodegradation and thermal properties of poly(lactic acid)/layered silicate nanocomposites, Compos. Interfaces, 2010, 17, 143–158 CrossRef CAS.
- J. M. Steinbach, Y. E. Seo and W. M. Saltzman, Cell penetrating peptide-modified poly(lactic-co-glycolic acid) nanoparticles with enhanced cell internalization, Acta Biomater., 2016, 30, 49–61 CrossRef CAS PubMed.
- J. T. Garcia, Comparative degradation study of biodegradable microspheres of poly(DL-lactide-co-glycolide) with poly(ethyleneglycol) derivates, J. Microencapsulation, 1999, 16, 83–94 CrossRef CAS PubMed.
- N. Kamaly, B. Yameen, J. Wu and O. C. Farokhzad, Degradable Controlled-Release Polymers and Polymeric Nanoparticles: Mechanisms of Controlling Drug Release, Chem. Rev., 2016, 116, 2602–2663 CrossRef CAS PubMed.
- B. Jeong, Y. H. Bae and S. W. Kim, In situ gelation of PEG–PLGA–PEG triblock copolymer aqueous solutions and degradation thereof, J. Biomed. Mater. Res., 2000, 50, 171–177 CrossRef CAS PubMed.
- C. W. Chang, D. Choi, W. J. Kim, J. W. Yockman, L. V. Christensen, Y. H. Kim and S. W. Kim, Non-ionic amphiphilic biodegradable PEG–PLGA–PEG copolymer enhances gene delivery efficiency in rat skeletal muscle, J. Controlled Release, 2007, 118, 245–253 CrossRef CAS PubMed.
- F. Li, D. Yu, X. Lin, D. Liu, H. Xia and S. Chen, Biodegradation of poly(ε-caprolactone) (PCL) by a new Penicillium oxalicum strain DSYD05-1, World J. Microbiol. Biotechnol., 2012, 28, 2929–2935 CrossRef CAS PubMed.
- E. Murray, B. C. Thompson, S. Sayyar and G. G. Wallace, Enzymatic degradation of graphene/polycaprolactone materials for tissue engineering, Polym. Degrad. Stab., 2015, 111, 71–77 CrossRef CAS.
- S. Allı, R. S. T. Aydın, A. Allı and B. Hazer, Biodegradable Poly(ε-Caprolactone)-Based Graft Copolymers Via Poly(Linoleic Acid): In Vitro Enzymatic Evaluation, J. Am. Oil Chem. Soc., 2015, 92, 449–458 CrossRef.
- C. Marega and A. Marigo, Effect of electrospun fibers of polyhydroxybutyrate filled with different organoclays on morphology, biodegradation, and thermal stability of poly(ε-caprolactone), J. Appl. Polym. Sci., 2015 DOI:10.1002/app.42342.
- S. P. Pawar, S. Kumar, A. Misra, S. Deshmukh, K. Chatterjee and S. Bose, Enzymatically degradable EMI shielding materials derived from PCL based nanocomposites, RSC Adv., 2015, 5, 17716–17725 RSC.
- Y. Phua, N. Lau, K. Sudesh, W. Chow and Z. M. Ishak, Biodegradability studies of poly(butylene succinate)/organo-montmorillonite nanocomposites under controlled compost soil conditions: effects of clay loading and compatibiliser, Polym. Degrad. Stab., 2012, 97, 1345–1354 CrossRef CAS.
- B. P. Calabia, F. Ninomiya, H. Yagi, A. Oishi, K. Taguchi and M. Kunioka, et al., Biodegradable poly(butylene succinate) composites reinforced by cotton fiber with silane coupling agent, Polymers, 2013, 5, 128–141 CrossRef.
- S.-H. Lee and M.-N. Kim, Isolation of bacteria degrading poly(butylene succinate-co-butylene adipate) and their lip A gene, Int. Biodeterior. Biodegrad., 2010, 64, 184–190 CrossRef CAS.
- S. P. C. Gonçalves and S. M. Martins-Franchetti, Action of soil microorganisms on PCL and PHBV blend and films, J. Polym. Environ., 2010, 18, 714–719 CrossRef.
- D. M. Abou-Zeid, R. J. Müller and W. Deckwer, Degradation of natural and synthetic polyesters under anaerobic conditions, J. Biotechnol., 2001, 86(2), 113–126 CrossRef CAS PubMed.
- J. Pérez, J. Munoz-Dorado, T. D. L. R. de la Rubia and J. Martinez, Biodegradation and biological treatments of cellulose, hemicellulose and lignin: an overview, Int. Microbiol., 2002, 5, 53–63 CrossRef PubMed.
- R. K. Sukumaran, R. R. Singhania, G. M. Mathew and A. Pandey, Cellulase production using biomass feed stock and its application in lignocellulose saccharification for bio-ethanol production, Renewable Energy, 2009, 34, 421–424 CrossRef CAS.
- M. Miao, R. Li, C. Huang, B. Jiang and T. Zhang, Impact of β-amylase degradation on properties of sugary maize soluble starch particles, Food Chem., 2015, 177, 1–7 CrossRef CAS PubMed.
- M. Li, T. Witt, F. Xie, F. J. Warren, P. J. Halley and R. G. Gilbert, Biodegradation of starch films: the roles of molecular and crystalline structure, Carbohydr. Polym., 2015, 122, 115–122 CrossRef CAS PubMed.
- J. P. Maran, V. Sivakumar, K. Thirugnanasambandham and R. Sridhar, Degradation behavior of biocomposites based on cassava starch buried under indoor soil conditions, Carbohydr. Polym., 2014, 101, 20–28 CrossRef CAS PubMed.
- Z. N. Hanani, Y. Roos and J. Kerry, Use and application of gelatin as potential biodegradable packaging materials for food products, Int. J. Biol. Macromol., 2014, 71, 94–102 CrossRef PubMed.
- B. D. Kevadiya, S. Rajkumar, H. C. Bajaj, S. S. Chettiar, K. Gosai and H. Brahmbhatt, et al., Biodegradable gelatin–ciprofloxacin–montmorillonite composite hydrogels for controlled drug release and wound dressing application, Colloids Surf., B, 2014, 122, 175–183 CrossRef CAS PubMed.
- Y. Li, J. Rodrigues and H. Tomas, Injectable and biodegradable hydrogels: gelation, biodegradation and biomedical applications, Chem. Soc. Rev., 2012, 41, 2193–2221 RSC.
- C. Gao, M. Liu, J. Chen and X. Zhang, Preparation and controlled degradation of oxidized sodium alginate hydrogel, Polym. Degrad. Stab., 2009, 94, 1405–1410 CrossRef CAS.
- H. J. Kong, D. Kaigler, K. Kim and D. J. Mooney, Controlling rigidity and degradation of alginate hydrogels via molecular weight distribution, Biomacromolecules, 2004, 5, 1720–1727 CrossRef CAS PubMed.
- A. K. Mahanta, V. Mittal, N. Singh, D. Dash, S. Malik, M. Kumar and P. Maiti, Polyurethane-Grafted Chitosan as New Biomaterials for Controlled Drug Delivery, Macromolecules, 2015, 48, 2654–2666 CrossRef CAS.
- W. Xia, P. Liu and J. Liu, Advance in chitosan hydrolysis by non-specific cellulases, Bioresour. Technol., 2008, 99, 6751–6762 CrossRef CAS PubMed.
- M. S. Brzezinska, U. Jankiewicz and M. Walczak, Biodegradation of chitinous substances and chitinase production by the soil actinomycete Streptomyces rimosus, Int. Biodeterior. Biodegrad., 2013, 84, 104–110 CrossRef CAS.
- D. Mawad, C. Warren, M. Barton, D. Mahns, J. Morley and B. T. Pham, et al., Lysozyme depolymerization of photo-activated chitosan adhesive films, Carbohydr. Polym., 2015, 121, 56–63 CrossRef CAS PubMed.
- D. Mudgil, S. Barak and B. Khatkar, Effect of enzymatic depolymerization on physicochemical and rheological properties of guar gum, Carbohydr. Polym., 2012, 90, 224–228 CrossRef CAS PubMed.
- W.-J. Chi, Y.-K. Chang and S.-K. Hong, Agar degradation by microorganisms and agar-degrading enzymes, Appl. Microbiol. Biotechnol., 2012, 94, 917–930 CrossRef CAS PubMed.
- Y. Freile-Pelegrín, T. Madera-Santana, D. Robledo, L. Veleva, P. Quintana and J. Azamar, Degradation of agar films in a humid tropical climate: thermal, mechanical, morphological and structural changes, Polym. Degrad. Stab., 2007, 92, 244–252 CrossRef.
- Y. Li, C. Han, J. Bian, L. Han, L. Dong and G. Gao, Rheology and biodegradation of polylactide/silica nanocomposites, Polym. Compos., 2012, 33, 1719–1727 CrossRef CAS.
- Y. B. Luo, X. L. Wang and Y. Z. Wang, Effect of TiO2 nanoparticles on the long-term hydrolytic degradation behavior of PLA, Polym. Degrad. Stab., 2012, 97, 721–728 CrossRef CAS.
- T. Niemelä, Effect of β-tricalcium phosphate addition on the in vitro degradation of self-reinforced poly-L,D-lactide, Polym. Degrad. Stab., 2005, 89, 492–500 CrossRef.
- M. J. Sobkowicz, J. L. Feaver and J. R. Dorgan, Clean and green bioplastic composites: comparison of calcium sulfate and carbon nanospheres in polylactide composites, Clean: Soil, Air, Water, 2008, 36, 706–713 CrossRef CAS.
- G. S. Deshmukh, S. U. Pathak, D. R. Peshwe and J. D. Ekhe, Effect of uncoated calcium carbonate and stearic acid coated calcium carbonate on mechanical, thermal and structuralproperties of poly(butylene terephthalate) (PBT)/calcium carbonate composites, Bull. Mater. Sci., 2010, 33, 277–284 CrossRef CAS.
- X. Shi, G. Zhang, C. Siligardi, G. Ori and A. Lazzeri, Comparison of Precipitated Calcium Carbonate/Polylactic Acid and Halloysite/Polylactic Acid Nanocomposites, J. Nanomater., 2015 DOI:10.1155/2015/905210.
- N. Teramoto, K. Urata, K. Ozawa and M. Shibata, Biodegradation of aliphatic polyester composites reinforced by abaca fiber, Polym. Degrad. Stab., 2004, 86, 401–409 CrossRef CAS.
- E. Fortunati, D. Puglia, M. Monti, C. Santulli, M. Maniruzzaman, M. L. Foresti, A. Vazquez and J. M. Kenny, Okra (Abelmoschus esculentus) fibre based PLA composites: mechanical behaviour and biodegradation, J. Polym. Environ., 2013, 21, 726–737 CrossRef CAS.
- A. A. Yussuf, I. Massoumi and A. Hassan, Comparison of polylactic acid/kenaf and polylactic acid/rise husk composites: the influence of the natural fibers on the mechanical, thermal and biodegradability properties, J. Polym. Environ., 2010, 18, 422–429 CrossRef CAS.
- P. J. Jandas, S. Mohanty and S. K. Nayak, Renewable resource-based biocomposites of various surface treated banana fiber and poly lactic acid: characterization and biodegradability, J. Polym. Environ., 2012, 20, 583–595 CrossRef CAS.
- A. S. Harmaen, A. Khalina, I. Azowa, M. A. Hassan, A. Tarmian and M. Jawaid, Thermal and biodegradation properties of poly(lactic acid)/fertilizer/oil palm fibers blends biocomposites, Polym. Compos., 2015, 36, 576–583 CrossRef CAS.
- L. Liu, J. Yu, L. Cheng and X. Yang, Biodegradability of poly(butylene succinate) (PBS) composite reinforced with jute fibre, Polym. Degrad. Stab., 2009, 94, 90–94 CrossRef CAS.
- F. Ma, X. Lu, Z. Wang, Z. Sun, F. Zhang and Y. Zheng, Nanocomposites of poly(L-lactide) and surface modified magnesia nanoparticles: fabrication, mechanical property and biodegradability, J. Phys. Chem. Solids, 2011, 72, 111–116 CrossRef CAS.
- D. N. Bikiaris, Nanocomposites of aliphatic polyesters: an overview of the effect of different nanofillers on enzymatic hydrolysis and biodegradation of polyesters, Polym. Degrad. Stab., 2013, 98, 1908–1928 CrossRef CAS.
- L. Han, C. Han, W. Cao, X. Wang, J. Bian and L. Dong, Preparation and characterization of biodegradable poly(3-hydroxybutyrate-co-4-hydroxybutyrate)/silica nanocomposites, Polym. Eng. Sci., 2012, 52, 250–258 CAS.
- X. Yu, S. Zhou, X. Zheng, Y. Xiao and T. Guo, Influence of in vitro degradation of a biodegradable nanocomposite on its shape memory effect, J. Phys. Chem. C, 2009, 113, 17630–17635 CAS.
- N. K. Singh, S. K. Singh, D. Dash, P. Gonugunta, M. Misra and P. Maiti, CNT induced β-phase in polylactide: unique crystallization, biodegradation, and biocompatibility, J. Phys. Chem. C, 2013, 117, 10163–10174 CAS.
- S. S. Ray, K. Yamada, A. Ogami, M. Okamoto and K. Ueda, New polylactide/layered silicate nanocomposite: nanoscale control over multiple properties, Macromol. Rapid Commun., 2002, 23, 943–947 CrossRef CAS.
- X. Wu, Y. Ma, G. Zhang, Y. Chu, J. Du and Y. Zhang, et al., Thermally Stable, Biocompatible, and Flexible Organic Field-Effect Transistors and Their Application in Temperature Sensing Arrays for Artificial Skin, Adv. Funct. Mater., 2015, 25, 2138–2146 CrossRef CAS.
- J. B. Jonnalagadda and I. V. Rivero, Effect of cryomilling times on the resultant properties of porous biodegradable poly(ε-caprolactone)/poly(glycolic acid) scaffolds for articular cartilage tissue engineering, J. Mech. Behav. Biomed. Mater., 2014, 40, 33–41 CrossRef CAS PubMed.
- A. P. Bonartsev, V. L. Myshkina, D. A. Nikolaeva, E. K. Furina, T. A. Makhina, V. A. Livshits, A. P. Boskhomdzhiev, E. A. Ivanov, A. L. Iordanskii and G. A. Bonartseva, Biosynthesis, biodegradation, and application of poly(3-hydroxybutyrate) and its copolymers-natural polyesters produced by diazotrophic bacteria, Communicating Current Research and Educational Topics and Trends in Applied Microbiology, 2007, vol. 1, pp. 295–307 Search PubMed.
- http://www.depuysynthes.com/hcp/biomaterials/products/qs/RAPIDSORB-Fixation-System.
- R. P. Meinig, Clinical use of resorbable polymeric membranes in the treatment of bone defects, Orthop. Clin. N. Am., 2010, 41, 39–47 CrossRef PubMed.
- N. Ensari, H. Tutar, O. Ekinci, M. B. Ugur, Y. A. Bayazıt and C. Gokdogan, et al., Effects of polylactic acid film on middle ear mucosa and cochlear function in guinea pigs, Arch. Oto-Rhino-Laryngol., 2015, 272, 1091–1097 CrossRef PubMed.
- G. Z. Teoh, C. Crowley, M. Birchall and A. Seifalian, Development of resorbable nanocomposite tracheal and bronchial scaffolds for paediatric applications, Br. J. Surg., 2015, 102, 140–150 CrossRef PubMed.
- E. Saito, D. Suarez-Gonzalez, W. L. Murphy and S. J. Hollister, Biomineral Coating Increases Bone Formation by Ex Vivo BMP-7 Gene Therapy in Rapid Prototyped Poly(L-lactic acid) (PLLA) and Poly(ε-caprolactone) (PCL) Porous Scaffolds, Adv. Healthcare Mater., 2015, 4, 621–632 CrossRef CAS PubMed.
- M. D. Schofer, P. P. Roessler, J. Schaefer, C. Theisen, S. Schlimme and J. T. Heverhagen, et al., Electrospun PLLA nanofiber scaffolds and their use in combination with BMP-2 for reconstruction of bone defects, PLoS One, 2011, 6, 25462 Search PubMed.
- X. Yang and Z. Li, Influence of hydroxyapatite and BMP-2 on bioactivity and bone tissue formation ability of electrospun PLLA nanofibers, J. Appl. Polym. Sci., 2015, 132 DOI:10.1002/app.42249.
- A. S. Mistry and A. G. Mikos, Tissue engineering strategies for bone regeneration, in Regenerative medicine II, Springer, Berlin Heidelberg, 2005 Jan 1, pp. 1–22 Search PubMed.
- G. Kapusetti, N. Misra, V. Singh, S. Srivastava, P. Roy and K. Dana, et al., Bone cement based nanohybrid as a super biomaterial for bone healing, J. Mater. Chem. B, 2014, 2, 3984–3997 RSC.
- P. Ceschi, A. Bohl, K. Sternberg, A. Neumeister, V. Senz and K. Schmitz, et al., Biodegradable polymeric coatings on cochlear implant surfaces and their influence on spiral ganglion cell survival, J. Biomed. Mater. Res., Part B, 2014, 102, 1255–1267 CrossRef CAS PubMed.
- V. H. Orozco, V. Kozlovskaya, E. Kharlampieva, B. L. López and V. V. Tsukruk, Biodegradable self-reporting nanocomposite films of poly(lactic acid) nanoparticles engineered by layer-by-layer assembly, Polymer, 2010, 51, 4127–4139 CrossRef CAS.
- A. Grumezescu, Nanobiomaterials in Hard Tissue Engineering: Applications of Nanobiomaterials, 2016 Search PubMed.
- K. Whang, C. H. Thomas, K. E. Healy and G. Nuber, A novel method to fabricate bioabsorbable scaffolds, Polymer, 1995, 36(4), 837–842 CrossRef CAS.
- H. Lo, M. S. Ponticiello and K. W. Leong, Fabrication of controlled release biodegradable foams by phase separation, Tissue Eng., 1995, 1(1), 15–28 CrossRef CAS PubMed.
- C. M. Agrawal and K. A. Athanasiou, Technique to control pH in vicinity of biodegrading PLA–PGA implants, J. Biomed. Mater. Res., 1997, 38(2), 105–114 CrossRef CAS PubMed.
- K. S. Stankevich, A. Gudima, V. D. Filimonov, H. Klüter, E. M. Mamontova and S. I. Tverdokhlebov, et al., Surface modification of biomaterials based on high-molecular polylactic acid and their effect on inflammatory reactions of primary human monocyte-derived macrophages: perspective for personalized therapy, Mater. Sci. Eng. C., 2015, 51, 117–126 CrossRef CAS PubMed.
- S. K. Pandey, D. K. Patel, R. Thakur, D. P. Mishra, P. Maiti and C. Haldar, Anti-cancer evaluation of quercetin embedded PLA nanoparticles synthesized by emulsified nanoprecipitation, Int. J. Biol. Macromol., 2015, 75, 521–529 CrossRef CAS PubMed.
- N. Uzun, T. D. Martins, G. M. Teixeira, N. L. Cunha, R. B. Oliveira and E. J. Nassar, et al., Poly(L-lactic acid) membranes: absence of genotoxic hazard and potentialfor drug delivery, Toxicol. Lett., 2015, 232, 513–518 CrossRef CAS PubMed.
- S. J. Parka, Y. J. Leea, D. N. Heo, I. K. Kwon, K. S. Yun, J. Y. Kang and S. H. Lee, Functional nerve cuff electrode with controllable anti-inflammatory drug loading and release by biodegradable nanofibers and hydrogel deposition, Sens. Actuators, B, 2015, 215, 133–141 CrossRef.
- S. Yang, F. Zhu, Q. Wang, F. Liang, X. Qu and Z. Gan, et al., Combinatorial targeting polymeric micelles for anti-tumor drug delivery, J. Mater. Chem. B, 2015, 3, 4043–4051 RSC.
- P. Carsten Christophersen, M. Fano, L. Saaby, M. Yang, H. Mørck Nielsen and H. Mu, Characterization of particulate drug delivery systems for oral delivery of peptide and protein drugs, Curr. Pharm. Des., 2015, 21, 2611–2628 CrossRef.
- C. Damgé, P. Maincent and N. Ubrich, Oral delivery of insulin associated to polymeric nanoparticles in diabetic rats, J. Controlled Release, 2007, 117, 163–170 CrossRef PubMed.
- Q. Guan, W. Chen and X. Hu, Development of lovastatin-loaded poly(lactic acid) microspheres for sustained oral delivery: in vitro and ex vivo evaluation, Drug Des., Dev. Ther., 2015, 9, 791 CrossRef PubMed.
- A. Kumari, S. K. Yadav and S. C. Yadav, Biodegradable polymeric nanoparticles based drug delivery systems, Colloids Surf., B, 2010, 75, 1–18 CrossRef CAS PubMed.
- H. K. Makadia and S. J. Siegel, Poly lactic-co-glycolic acid (PLGA) as biodegradable controlled drug delivery carrier, Polymers, 2011, 3, 1377–1397 CrossRef CAS PubMed.
- K. E. Uhrich, S. M. Cannizzaro, R. S. Langer and K. M. Shakesheff, Polymeric systems for controlled drug release, Chem. Rev., 1999, 99, 3181–3198 CrossRef CAS PubMed.
- I. Yamakawa, M. Ishida, T. Kato, H. Ando and N. Asakawa, Release behavior of poly(lactic acid-co-glycolic acid) implants containing phosphorothioate oligodeoxynucleotide, Biol. Pharm. Bull., 1997, 20, 455–459 CAS.
- K. Tojo, H. Aoyagi and T. Kurita, Surface Dissolution-Bulk Erosion Model of Drug Release from Biodegradable Polymer Rods, J. Chem. Eng. Jpn., 1998, 31, 648–651 CrossRef CAS.
- M. J. Kipper and B. Narasimhan, Molecular Description of Erosion Phenomena in Biodegradable Polymers, Macromolecules, 2005, 38, 1989–1999 CrossRef CAS.
- P. F. McDonald, J. G. Lyons, L. M. Geever and C. L. Higginbotham, In vitro degradation and drug release from polymer blends based on poly(DL-lactide), poly(L-lactide–glycolide) and poly(ε-caprolactone), J. Mater. Sci., 2010, 45, 1284–1292 CrossRef CAS.
- J. Ahmed and S. K. Varshney, Polylactides—chemistry, properties and green packaging technology: a review, Int. J. Food Prop., 2011, 14, 37–58 CrossRef CAS.
- http://www.greenerpackage.com/bioplastics/danone_first_switch_pla_yogurt_cup_germany#comment-post-region.
- Y. Qin, J. Yang and J. Xue, Characterization of antimicrobial poly(lactic acid)/poly(trimethylene carbonate) films with cinnamaldehyde, J. Mater. Sci., 2015, 50, 1150–1158 CrossRef CAS.
- R. Zhao, H. Wang, T. Ji, G. Anderson, G. Nie and Y. Zhao, Biodegradable cationic ε-poly-L-lysine-conjugated polymeric nanoparticles as a new effective antibacterial agent, Sci. Bull., 2015, 60, 216–226 CrossRef CAS.
- J. W. Rhim, H. M. Park and C. S. Ha, Bio-nanocomposites for food packaging applications, Prog. Polym. Sci., 2013, 38, 1629–1652 CrossRef CAS.
- F. Yahiaoui, F. Benhacine, H. Ferfera-Harrar, A. Habi, A. S. Hadj-Hamou and Y. Grohens, Development of antimicrobial PCL/nanoclay nanocomposite films with enhanced mechanical and water vapor barrier properties for packaging applications, Polym. Bull., 2015, 72, 235–254 CrossRef CAS.
- T. Jin and H. Zhang, Biodegradable polylactic acid polymer with nisin for use in antimicrobial food packaging, J. Food Sci., 2008, 73, M127–M134 CrossRef CAS PubMed.
- B. Ghanbarzadeh, S. A. Oleyaei and H. Almasi, Nanostructured Materials Utilized in Biopolymer-based Plastics for Food Packaging Applications, Food Sci. Nutr., 2015, 55, 1699–1723 CAS.
- F. Yahiaoui, F. Benhacine, H. Ferfera-Harrar, A. Habi, A. S. Hadj-Hamou and Y. Grohens, Development of antimicrobial PCL/nanoclay nanocomposite films with enhanced mechanical and water vapor barrier properties for packaging applications, Polym. Bull., 2015, 72, 235–254 CrossRef CAS.
- L. Xie, H. Xu, J.-B. Chen, Z.-J. Zhang, B. S. Hsiao and G.-J. Zhong, et al., From Nanofibrillar to Nanolaminar Poly(butylene succinate): Paving the Way to Robust Barrier and Mechanical Properties for Full-Biodegradable Poly(lactic acid) Films, ACS Appl. Mater. Interfaces, 2015, 7, 8023–8032 CAS.
- M. P. Arrieta, M. D. Samper, J. López and A. Jiménez, Combined effect of poly(hydroxybutyrate) and plasticizers on polylactic acid properties for film intended for food packaging, J. Polym. Environ., 2014, 22, 460–470 CrossRef CAS.
|
This journal is © The Royal Society of Chemistry 2016 |
Click here to see how this site uses Cookies. View our privacy policy here.