DOI:
10.1039/C6RA08458C
(Paper)
RSC Adv., 2016,
6, 74802-74811
One-pot multicomponent reaction synthesis of spirooxindoles promoted by guanidine-functionalized magnetic Fe3O4 nanoparticles†
Received
1st April 2016
, Accepted 30th July 2016
First published on 1st August 2016
Abstract
Magnetic nanoparticles of Fe3O4-immobilized guanidine (MNPs-guanidine) have been characterized by powder X-ray diffraction (XRD), a vibrating sample magnetometer (VSM), scanning electronic microscopy (SEM), energy dispersive X-ray (EDS), X-ray photoelectron spectroscopy (XPS), DLS (dynamic light scattering), thermal gravimetric analysis (TGA) and FT-IR spectroscopy. A neat, atom-economical, environmentally benign one-pot multi-component synthetic route for the synthesis of spirooxindoles in good yields has been devised using guanidine-functionalized magnetic Fe3O4 nanoparticles. This method provides several advantages including mild reaction conditions, applicability to a wide range of substrates, reusability of the catalyst and little catalyst loading.
1. Introduction
Spirooxindoles are core building blocks for the structures of highly functionalized organic compounds, which are a subunit in alkaloids isolated from the roots of Gelsemium and Ophiorrhiza,1 leaves of Mitragyna parvifolia,2 and roots of Elueagnus commutate.3 Spirooxindoles are attractive targets in organic and medicinal chemistry due to their potency and wide spectrum of biological activities including antimicrobial,4–6 antitumor,7,8 antidiabetic,9 and can also serve as synthetic intermediates for many kinds of pharmaceuticals or drug precursors.10 Therefore, the development of simple, highly efficient methodologies for the preparation of spirooxindoles is still highly desirable. The synthesis of bioactive heterocycles from readily available starting materials using one-pot multicomponent reactions (MCRs) has gained considerable interest both from synthetic and medicinal chemists.11,12 The possibility of accomplishing multicomponent reactions under moderate conditions with a heterogeneous catalyst offers several advantages, including the benefits of green procedures, excellent synthetic efficiency, inherent atom economy, procedural simplicity and creation molecular complexity starting from simple substrates.13–16 Magnetic materials have emerged as a suitable group of heterogeneous catalysts owing to their numerous applications in organic syntheses. To overcome the separation problems of the routine methods, magnetic materials have emerged as recoverable catalysts. Separation of magnetic nanoparticles (MNs) is simple, convenient, economical and environmentally benign.17,18 The surface of MNPs can be functionalized easily through appropriate surface modifications to enable the loading of a variety of desirable functionalities. Recently, MNPs have been successfully used to immobilize enzymes, bioactive polymers, transition metal catalysts and organocatalysts.19–21 Surface functionalization of magnetic nanoparticles is a neat way to bridge the gap between heterogeneous and homogeneous catalysis. Therefore, the development of surface modification of magnetic nanoparticles as important candidates in the search for supporting of catalysts is currently an issue of increasing interest in chemical reactions.22–25
Guanidines are important classes of compounds that have been utilized as organic bases. Indeed, guanidines are stronger bases than other nitrogen compounds such as pyridines, amines, diamines and amidines. Hence, guanidines have been widely explored in numerous base-catalyzed organic reactions and some guanidines have proven to be advantageous when compared with other types of organic bases.26–28 Herein we wish to report the use of magnetic nanoparticle Fe3O4-immobilized guanidine as an efficient heterogeneous catalyst for the preparation of spirooxindoles (Scheme 1). Heterogeneous catalysts offer many advantages like easy separation of the catalyst from the reaction products and recycling.29,30 With an external magnetic field, the catalyst can be easily recycled, and long-life and high reusability are demonstrated without noticeable decrease in the catalytic performance after use for more than 7 times. The stabilization of organic layer on the magnetic support surface can explain the high catalytic activity and high reusability of our catalyst without losing considerable activity. Although different templates have their specific uses in regard to stabilization and precise control of the architecture of magnetic nanoparticles, functionalization by guanidines provides greater programmable capability due to their characteristic structural features and molecular recognition properties.
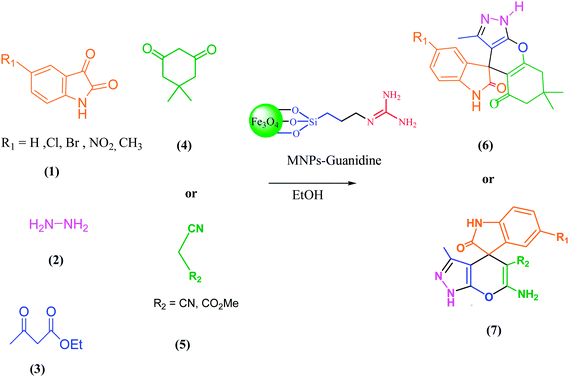 |
| Scheme 1 Synthesis of spirooxindoles using MNPs-guanidine. | |
The synthesis of spirooxindoles has been reported in the presence of diverse catalysts such as p-TSA,31,32 piperidine,33 alum (KAl(SO4)2·12H2O),34 amino-functionalized SBA-15 (SBA-Pr-NH2),35 nanocrystalline MgO,36 and L-Proline.37 However, some of the reported methods tolerate disadvantages including long reaction times, harsh reaction conditions and use of toxic and non-reusable catalyst. Therefore, to avoid these limitations, the exploration of an efficient, easily available catalyst with high catalytic activity and short reaction times for the preparation of spirooxindoles is still favored.
2. Results and discussion
The process of the preparation of guanidine-functionalized magnetic Fe3O4 nanoparticles is shown in Scheme 2.
 |
| Scheme 2 Schematic illustration of the preparation guanidine-functionalized magnetic Fe3O4 nanoparticles. | |
The particle size and morphology of guanidine-functionalized magnetic Fe3O4 nanoparticles (MNPs-guanidine) was determined by Scanning Electronic Microscopy (SEM). The SEM images show particles with diameters in the nanometer range (Fig. 1).
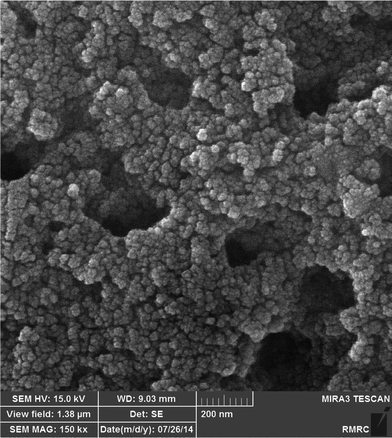 |
| Fig. 1 SEM image of MNPs-guanidine. | |
Fig. 2 shows the powder X-ray diffraction (XRD) spectrum. The pattern agrees well with the reported pattern for Fe3O4 (JCPDS no. 75-1609). All the strong peaks appeared at 2θ = 30.089°, 35.406°, 43.150°, 53.598°, 57.206°, 62.865°, and 74.029° can be easily indexed to nano-Fe3O4. The crystallite size diameter (D) of the MNPs-guanidine has been calculated by the Debye–Scherer equation (D = Kλ/β
cos
θ), where θ is the position site of the maximum of the diffraction peak and β is the full-width at half-maximum (FWHM), and λ is the X-ray wavelength (1.54 Å for Cu Kα), K is called shape factor, which usually takes a value of about 0.9. The crystallite size of MNPs-guanidine calculated by the Debye–Scherer equation is about 35–40 nm, in good agreement with the result obtained by SEM.
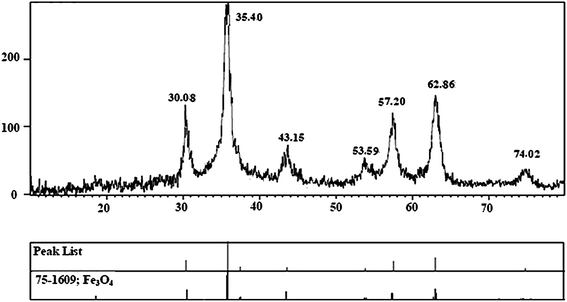 |
| Fig. 2 The XRD pattern of MNPs-guanidine. | |
Fig. 3 shows FT-IR spectra of MNPs coated by (3-chloropropyl)-trimethoxysilane (MNPs-CPTMS) and guanidine-functionalized magnetic Fe3O4 nanoparticles (MNPs-guanidine). The FT-IR spectra of MNPs-CPTMS and MNPs-guanidine show the vibrations of Fe–O at 570–588 cm−1. The introduction of CPTMS to the surface of MNPs is confirmed by the bands at 1005 and 1128 cm−1 assigned to the Fe–O–Si and C–Cl stretching vibrations respectively. Reaction of MNPs-CPTMS with guanidine produces MNPs-guanidine in which the presence of guanidine moiety is asserted with 1443 and 3394 cm−1 bands corresponding to the C–N and N–H stretches respectively.
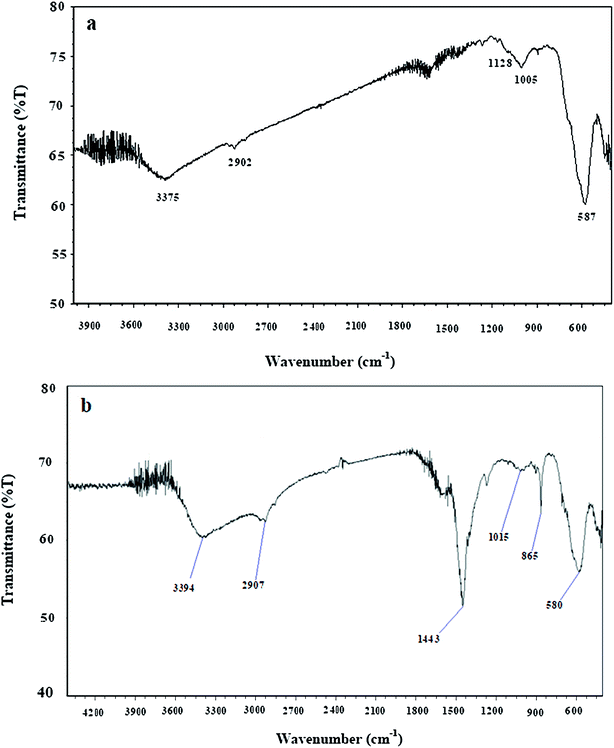 |
| Fig. 3 FT-IR spectra of MNPs coated by (3-chloropropyl)-trimethoxysilane (MNPs-CPTMS) (a) and guanidine-functionalized magnetic Fe3O4 nanoparticles (MNPs-guanidine) (b). | |
The magnetic properties of MNPs-CPTMS and MNPs-guanidine were revealed with the help of a vibrating sample magnetometer (VSM) (Fig. 4). The amount of saturation-magnetization for MNPs-CPTMS and MNPs-guanidine is 47.2 emu g−1 and 24.9 emu g−1. These results demonstrate that the magnetization property decreases by coating and functionalization.
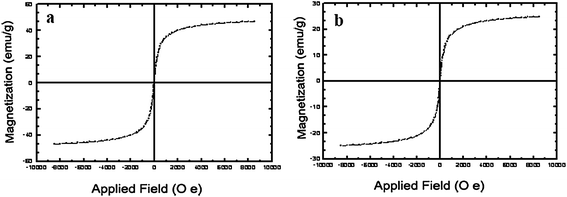 |
| Fig. 4 The M–H plots of: MNPs-CPTMS (a) and MNPs-guanidine (b). | |
Thermogravimetric analysis (TGA) evaluates the thermal stability of the MNPs-CPTMS and MNPs-guanidine. These nanoparticles show suitable thermal stability without significantly decreasing in weight (Fig. S1) (see ESI†). The weight loss at temperatures below 200 °C is due to the removal of physically adsorbed solvent and surface hydroxyl groups. The curve shows a weight loss about 10% from 250 to 600 °C, resulting from the decomposition of organic spacer grafting to the MNPs surface.
EDS (energy dispersive X-ray) spectrum of MNPs-guanidine (Fig. 5) shows that the elemental compositions are carbon, oxygen, silicon, iron and nitrogen.
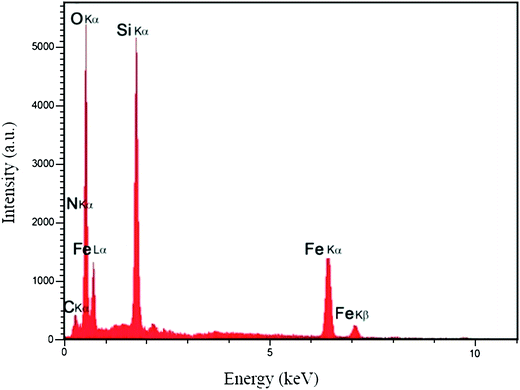 |
| Fig. 5 EDS spectrum of MNPs-guanidine. | |
X-ray photoelectron spectroscopy (XPS) analysis was used to investigate the chemical binding in the synthesized MNPs-guanidine. In the wide-scan spectrum of functionalized MNPs (Fig. S2) (see ESI†), the predominant components are Si 2p (103 eV), Si 2s (150 eV), C 1s (284 eV), N 1s (400 eV), O 1s (530 eV), and Fe 2p (710 eV).
In order to investigate the size distribution of nanoparticles, DLS (dynamic light scattering) graph of the nanoparticles were presented in Fig. S3 (see ESI†). This size distribution is centered at a value of 44 nm.
Initially, we had explored and optimized different reaction parameters for the synthesis of spirooxindoles by the condensation reaction of dimedone or malononitrile, hydrazine hydrate, ethyl acetoacetate and isatin as a model reaction. The model reactions were carried out in the presence of various catalysts such as CeO2 NPs, CaO NPs, SnO NPs, Fe3O4 NPs and MNPs-guanidine. Several reactions were scrutinized using various solvents such as EtOH, CH3CN, water, DMF. The best results were obtained in ethanol and found that the reaction gave satisfying results in the presence of MNPs-guanidine which gave excellent yields of products (Table 1). The catalyst showed best activity in ethanol compared to other organic solvents such as DMF, CH3CN and H2O.
Table 1 Optimization of reaction conditions using different catalystsa,b
Entry |
Solvent (reflux condition) |
Catalyst |
Time (min) |
Yielda%/Yieldb% |
Isolated yield: hydrazine hydrate (1.1 mmol), ethyl acetoacetate (1.1 mmol), isatin (1 mmol) and dimedone (1 mmol). Isolated yield: hydrazine hydrate (1.1 m mol), ethyl acetoacetate (1.1 mmol), isatin (1 m mol) and malononitrile (1.1 mmol). |
1 |
EtOH |
— |
200 |
8/10 |
2 |
EtOH |
CeO2 NPs (0.05 g) |
60 |
44/25 |
3 |
EtOH |
CaO NPs (0.07 g) |
60 |
33/52 |
4 |
EtOH |
SnO NPs (0.07 g) |
60 |
35/26 |
5 |
EtOH |
Fe3O4 NP (0.05 g) |
60 |
38/31 |
6 |
H2O |
MNPs-guanidine (0.02 g) |
25 |
60/45 |
7 |
DMF |
MNPs-guanidine (0.02 g) |
25 |
72/68 |
8 |
CH3CN |
MNPs-guanidine (0.02 g) |
25 |
82/75 |
9 |
EtOH |
MNPs-guanidine (0.01 g) |
25 |
85/89 |
10 |
EtOH |
MNPs-guanidine (0.02 g) |
25 |
87/91 |
11 |
EtOH |
MNPs-guanidine (0.03 g) |
25 |
87/93 |
12 |
EtOH |
MNPs-guanidine (0.04 g) |
25 |
87/93 |
To investigate the scope and limitation of this catalytic process, isatins, dimedone, malononitrile or methyl cyanoacetate were chosen as substrates. We first examined the reaction of dimedone, hydrazine hydrate, ethyl acetoacetate with a diverse array of isatins. Then, malononitrile or methyl cyanoacetate utilize for reaction with hydrazine hydrate, ethyl acetoacetate and isatins. The above results obviously show the present catalytic procedure is extendable to a wide variety of substrates to construct a diversity-oriented library of spirooxindoles. Investigations of the reaction scope revealed that various aromatic isatins (bearing electron-withdrawing and electron-donating groups) can be utilized in this protocol (Table 2).
Table 2 Synthesis of spirooxindoles by MNPs-guanidine
Entry |
Isatin |
4 or 5 |
Product |
Time (min) |
Yielda % |
M. P. °C |
Isolated yield. |
1 |
 |
 |
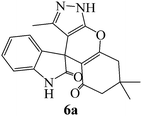 |
25 |
87 |
220–222 |
2 |
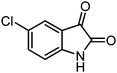 |
 |
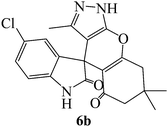 |
25 |
90 |
215–217 |
3 |
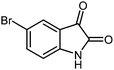 |
 |
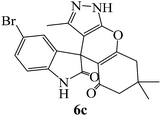 |
25 |
89 |
185–187 |
4 |
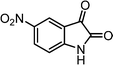 |
 |
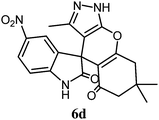 |
25 |
91 |
200–202 |
5 |
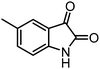 |
 |
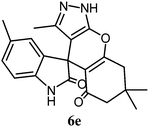 |
25 |
85 |
193–195 |
6 |
 |
 |
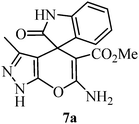 |
30 |
90 |
268–270 |
7 |
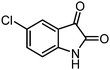 |
 |
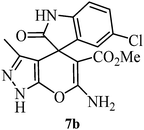 |
30 |
92 |
273–275 |
8 |
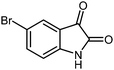 |
 |
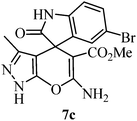 |
30 |
90 |
250–252 |
9 |
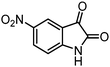 |
 |
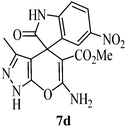 |
30 |
92 |
268–270 |
10 |
 |
 |
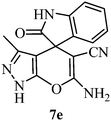 |
25 |
93 |
224–226 (ref. 33) |
11 |
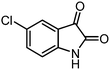 |
 |
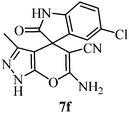 |
25 |
95 |
297–298 (ref. 38) |
12 |
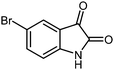 |
 |
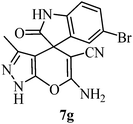 |
25 |
94 |
243–245 (ref. 33) |
13 |
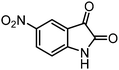 |
 |
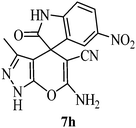 |
25 |
95 |
270–272 (ref. 33) |
The reusability is one of the significant properties of this catalyst. The reusability of MNPs-guanidine was studied for the reaction of isatin, hydrazine hydrate, ethyl acetoacetate and dimedone or malononitrile. After completion of the reaction, the nanocatalyst was easily separated using an external magnet. The recovered magnetite nanoparticles were washed several times with acetone and then dried at room temperature. Fig. 6 indicates that the catalyst could be reused for seven times with a minimal loss of activity.
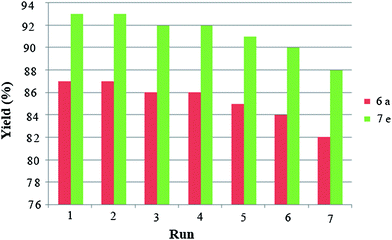 |
| Fig. 6 Reusability of MNPs-guanidine as catalyst for the synthesis of 6a and 7e. | |
The results indicate a suggestive mechanism for this reaction. Scheme 3 shows a proposed mechanism for this reaction in the presence of MNPs-guanidine as catalyst. Initially hydrazine hydrate is reacted with 1,3-dicarbonyl compound to form intermediate (I) via condensation reaction. Secondly, intermediate (I), in the presence of MNPs-guanidine, is condensed with isatin derivatives to form intermediate (II) via Knoevenagel condensation reaction. In the next step, malononitrile or methyl cyanoacetate reacts with intermediate (II) through Michael addition. Lastly, the final product is formed by intra-molecular cyclization reactions. This proposed mechanism has also been supported by literature.39–41
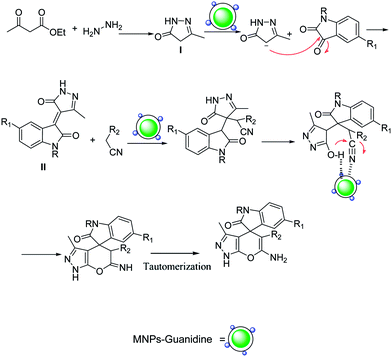 |
| Scheme 3 Proposed reaction pathway for the synthesis of spirooxindoles using MNPs-guanidine as catalyst. | |
Scheme 4 shows a proposed mechanism for this reaction in the presence of MNPs-guanidine as catalyst. Initially hydrazine hydrate reacted with 1,3-dicarbonyl compound to form intermediate (I) via condensation reaction. Secondary, intermediate (I) condensed with isatin derivatives to form intermediate (II) via Knoevenagel condensation reaction. In the next step, dimedone reacted with intermediate (II) through Michael addition in the presence of MNPs-guanidine. Lastly, the final product has been formed by intra-molecular cyclization reactions. This proposed mechanism also has been supported by literature.40–42
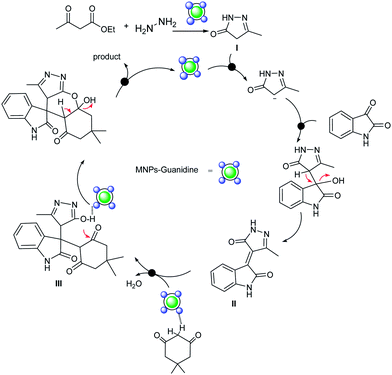 |
| Scheme 4 Proposed reaction pathway for the synthesis of spirooxindoles using MNPs-guanidine as catalyst. | |
3. Experimental
3.1. Chemicals and apparatus
The materials were purchased from Sigma-Aldrich and Merck in high purity. All of the materials were used without further purification. The elemental analyses (C, H, and N) were obtained by Carlo ERBA Model EA 1108 analyzer. The 1H NMR and 13C NMR spectra were recorded on Bruker Avance-400 MHz spectrometers in the presence of tetramethylsilane as internal standard and DMSO-d6 and D2O as solvents. All melting points were determined in capillary tubes on a Boetius melting point microscope. FT-IR spectra were obtained by WQF-510, spectrometer 550 Nicolet in KBr in the range of 400–4000 cm−1. The Energy-Dispersive X-ray Spectroscopy (EDS) measurement was carried out by SAMX analyser. X-ray photoelectron spectroscopy (XPS) spectra were measured on an ESCA-3000 electron spectrometer. DLS was performed using a Malvern Instruments. Magnetic properties were measured by a vibrating sample magnetometer (VSM, PPMS-9T) at 300 K in Iran (Kashan University). Powder XRD was performed on a Philips diffractometric of X'pert Company with Mono chromatized Cu Kα radiation (λ = 1.54056 Å). The SEM images were prepared by MIRA3-TESCAN. A TGAQ5 thermogravimetric analyser was used to study the thermal characteristic of the nanoparticles under heating at a rate of 10 °C min−1 and an inert N2 atmosphere at 20 mL min−1.
3.2. General procedure for the preparation of MNPs-guanidine
MNPs-guanidine was prepared according to the procedure reported in the literature with some modification.22
3.2.1 General procedure for the synthesis of Fe3O4 nanoparticles. Fe3O4 nanoparticles were prepared according to the procedure reported in the literature with some modification.43,44 The Fe3O4 nanoparticles were synthesized by co-precipitation of FeCl3·6H2O (11.68 g) and FeCl2·4H2O (4.30 g) were dissolved in 200 mL of deionized water, then 15 mL of 25% NH3·H2O was added to the solution dropwise under nitrogen gas and with vigorous stirring at 70–75 °C. The magnetic nanoparticles were separated from solution by an external magnetic decantation and washed twice with deionized water.
3.2.2 Preparation of MNPs coated by (3-chloropropyl)-trimethoxysilane (MNPs-CPTMS). The obtained MNPs powder (1.5 g) was dispersed in 250 mL ethanol/water (volume ratio, 1
:
1) solution by sonication for 30 min, and then (3-chloropropyl)-trimethoxysilane (99%, 2.5 mL) was added to the mixture. After mechanical stirring under N2 atmosphere at 33–38 °C for 8 h, the suspended substance was separated with centrifugation. The settled product was re-dispersed in ethanol by sonication. The final sample was separated by an external magnet and washed five times with ethanol.
3.2.3 Preparation of guanidine-functionalized magnetic Fe3O4 nanoparticles (MNPs-guanidine). The MNPs-CPTMS (1 g) was dispersed in dry toluene (6–8 mL) by ultrasonication for 10 min. Subsequently, guanidine hydrochloride (0.382 g, 0.004 mmol) and sodium bicarbonate (0.672 g, 0.008 mmol) were added and the mixture was refluxed for 28 h. Then, the final product was separated by magnetic decantation and washed twice by dry CH2Cl2, EtOH to remove the unattached substrates.
3.3. General procedure for the preparation of spirooxindoles
A mixture of isatin (1 mmol), hydrazine hydrate (1.1 mmol), ethyl acetoacetate (1.1 mmol), methyl cyanoacetate (1.1 mmol) or malonitiril (1.1 mmol) or dimedone (1 mmol), and MNPs-guanidine (0.03 g) were heated in EtOH (10 mL) under reflux conditions. The completion of the mixture was monitored by TLC and the catalyst was separated from reaction before work up by an external magnet field. Then the mixture was poured in to cold water, the precipitate obtained was filtered and recrystallized twice by (EtOAc/n-hexane 3
:
1). Afterward the products were characterized by 1H NMR, FT-IR, 13C NMR spectroscopy.
3.4. Spectral data
3.4.1 3,7,7-Trimethyl-7,8-dihydro-1H-spiro[chromeno [2,3-c]pyrazole-4,3′-indoline]-2′,5(6H)-dione (6a). Yellow solid, mp: 220–222 °C, 1H NMR (DMSO-d6, 400 MHz): δ 0.97 (6H, s, 2CH3), 1.40 (3H, s, CH3), 1.90–2.00 (2H, m, CH2), 2.20–2.30 (2H, m, CH2), 6.85 (1H, m, CH2), 6.87 (1H, m, CH2), 6.93–6.94 (1H, m, CH), 7.14 (1H, m, CH), 11.07 (1H, s, NH), 11.72 (1H, bs, NH) ppm. 13C NMR (100 MHz, DMSO-d6): δ 11.10, 25.42, 30.50, 42.48, 48.10, 51.82, 97.80, 110.50, 120.28, 124.52, 128.00, 130.73, 133.93, 136.72, 142.55, 146.80, 164.93, 173.74, 198.80 ppm. FT-IR (KBr): (vmax/cm−1): 3374 (stretch NH), 3182 (stretch NH), 1691, 1680 (stretch C
O), 1470 (aromatic C
C), 930 and 758. Anal. calcd for C20H19N3O3: C, 68.75; H, 5.48; N, 12.03. Found: C, 68.71; H, 5.52; N 12.07.
3.4.2 3,7,7-Trimethyl-7,8-dihydro-5′-chloro-1H-spiro[chromeno [2,3-c]pyrazole-4,3′-indoline]-2′,5(6H)-dione (6b). Cream solid, mp: 215–217 °C, 1H NMR (DMSO-d6, 400 MHz): δ 1.01 (6H, s, 2CH3), 1.78 (3H, s, CH3), 1.97–2.00 (2H, m, CH2), 2.21–2.24 (2H, m, CH2), 6.76 (1H, d, J = 8.0 Hz, CH), 7.12 (1H, d, J = 8.0 Hz, CH), 7.20 (1H, s, CH) 10.56 (1H, s, NH), 11.22 (1H, bs, NH) ppm. 13C NMR (100 MHz, DMSO-d6): δ 11.14, 27.44, 30.55, 44.90, 48.12, 52.10, 96.04, 111.10, 120.13, 126.00, 128.63, 130.65, 135.90, 137.00, 141.33, 145.90, 164.34, 172.13, 197.00 ppm. FT-IR (KBr): (vmax/cm−1): 3207 (stretch NH), 3110 (stretch NH), 1706 (stretch C
O), 1658 (stretch C
O), 1473 (aromatic C
C), 806, 769. Anal. calcd for C20H18ClN3O3: C, 62.58; H, 4.73; N; 10.95. Found: C, 62.62; H, 4.76; N, 10.91.
3.4.3 3,7,7-Trimethyl-7,8-dihydro-5′-bromo-1H-spiro[chromeno [2,3-c]pyrazole-4,3′-indoline]-2′,5(6H)-dione (6c). Yellow solid, mp: 185–187 °C, 1H NMR (DMSO-d6, 400 MHz): δ 1.02 (6H, s, 2 CH3), 1.80 (3H, s, CH3), 2.06–2.09 (2H, m, CH2), 2.26–2.30 (2H, s, CH2), 6.72 (1H, d, J = 7.2 Hz, CH), 7.25 (1H, d, J = 7.2 Hz, CH), 7.32 (1H, s, CH), 10.58 (1H, s, NH), 11.35 (1H, bs, NH) ppm. 13C NMR (100 MHz, DMSO-d6): δ 11.13, 25.33, 33.11, 44.80, 48.20, 52.68, 96.80, 111.51, 120.28, 124.51, 126.00, 130.74, 133.93, 136.74, 142.56, 146.70, 163.74, 174.33, 197.10 ppm. FT-IR (KBr): (vmax/cm−1): 3216 (stretch NH), 3110 (stretch NH), 1724 (stretch C
O), 1678 (stretch C
O), 1477 (aromatic C
C), 818, 660. Anal. calcd for C20H18BrN3O3: C, 56.09; H, 4.24; N, 9.81. Found: C, 56.05; H, 4.29; N, 9.92.
3.4.4 3,7,7-Trimethyl-7,8-dihydro-5′-nitro-1H-spiro[chromeno [2,3-c]pyrazole-4,3′-indoline]-2′,5(6H)-dione (6d). Yellow solid, mp: 200–202 °C, 1H NMR (DMSO-d6, 400 MHz): δ 1.05 (6H, s, 2CH3), 1.78 (3H, s, CH3), 2.00–2.01 (2H, m, CH2), 2.28–2.30 (2H, m, CH2), 7.04 (1H, d, J = 8.2 Hz, CH), 7.67 (1H, s, CH), 8.12 (1H, d, J = 8.2 Hz), 10.18 (1H, s, NH), 11.36 (1H, s, NH) ppm. 13C NMR (100 MHz, DMSO-d6): δ 11.11, 28.51, 32.16, 42.44, 46.83, 52.16, 96.90, 111.04, 119.41, 120.11, 126.71, 137.25, 138.51, 144.16, 148.89, 148.90, 164.02, 173.16, 198.90 ppm. FT-IR (KBr): (vmax/cm−1): 3222 (stretch NH), 3104 (stretch NH), 1735 (stretch C
O), 1684 (stretch C
O), 1517 and 1336 (stretch N–O), 1428 (aromatic C
C) and 694. Anal. calcd for C20H18N4O5: C, 60.91; H, 4.60; N, 14.21. Found: C, 60.87; H, 4.53; N, 14.32.
3.4.5 3,7,7-Trimethyl-7,8-dihydro-5′-methyl-1H-spiro[chromeno [2,3-c]pyrazole-4,3′-indoline]-2′,5(6H)-dione (6e). Yellow solid; mp: 193–195 °C; 1H NMR (DMSO-d6, 400 MHz) δ (ppm): 0.94 (6H, s, 2CH3), 1.72 (s, 3H, CH3), 1.83 (s, 3H, CH3), 1.98–2.13 (m, 2H, CH2), 2.17–2.28 (m, 2H, CH2), 6.90 (d, J = 6.9 Hz, 1H, CH), 7.13 (d, J = 6.9 Hz, 1H, CH), 7.48 (s, 1H, CH), 10.24 (s, 1H, NH), 10.78 (s, 1H, NH); 13C NMR (100 MHz, DMSO-d6) δ (ppm): 11.13, 25.33, 27.42, 33.11, 42.44, 46.83, 52.16, 98.32, 108.33, 111.54, 121.49, 127.63, 129.48, 135.65, 139.47, 143.32, 145.96, 164.34, 172.13, 197.00. FT-IR (KBr): (vmax/cm−1): 3303 (stretch NH), 3191 (stretch NH), 1690, 1682 (stretch C
O). Anal. calcd for C21H21N3O3: C, 69.41; H, 5.82; N, 11.56. Found: C, 69.47; H, 5.86; N 11.53.
3.4.6 Methyl 6′-amino-3′-methyl-2-oxo-2′H-spiro[indoline-3,4′-pyrano[2,3-c]pyrazole]-5′-carboxylate (7a). White solid; mp: 268–270 °C; 1H NMR (400 MHz, DMSO-d6): δ 1.65 (3H, s, CH3), 3.28 (3H, s, OCH3), 6.68–7.12 (4H, m, ArH), 7.87 (2H, br s, NH2), 9.97 (1H, s, NH), 11.87 (1H, s, NH) ppm; 13C NMR (100 MHz, DMSO-d6): δ 10.10, 48.82, 58.66, 78.51, 97.80, 111.50, 120.28, 124.52, 125.99, 130.73, 133.93, 136.72, 142.55, 156.80, 146.93, 173.74 ppm. FT-IR (KBr): (cm−1) (vmax/cm−1): 3492 and 3388 (stretch NH), 3253 (stretch NH), 1705 (stretch C
O), 1664, 1602, 1439, 1394, 1093, 930, 758; Anal. calcd for C16H14N4O4: C, 58.89; H, 4.32; N, 17.17; Found: C, 58.78; H, 4.39; N, 17.22.
3.4.7 Methyl 6′-Amino-3′-methyl-2-oxo-5-chloro-2′H-spiro [indoline-3,4′-pyrano[2,3-c]pyrazole]-5′-carboxylate (7b). White solid; mp: 273–275 °C; 1H NMR (400 MHz, DMSO-d6): δ 1.76 (3H, s, CH3), 3.33–3.35 (3H, s, OCH3), 6.94–7.28 (3H, s, ArH), 7.82 (2H, br s, NH2), 9.53 (1H, s, NH), 11.41 (1H, s, NH) ppm. 13C NMR (100 MHz, DMSO-d6): δ 10.14, 49.07, 56.07, 74.87, 96.04, 113.09, 120.13, 125.99, 128.63, 130.66, 135.90, 137.00, 141.33, 157.60, 164.39, 172.13 ppm. FT-IR (KBr): (vmax/cm−1): 3404 and 3283 (stretch NH), 3180 (stretch NH), 1698 (stretch C
O), 1670 (stretch C
O), 1601, 1550, 1469, 1207, 806, 769. Anal. calcd for C16H13ClN4O4: C, 53.27; H, 3.63; N, 15.53; Found: C, 53.21; H, 3.68; N, 15.45.
3.4.8 Methyl 6′-amino-3′-methyl-2-oxo-5-bromo-2′H-spiro[indoline-3,4′-pyrano[2,3-c]pyrazole]-5′-carboxylate (7c). Yellow solid; mp: 250–252 °C; 1H NMR (DMSO-d6, 400 MHz): δ 1.58 (3H, s, CH3), 3.25 (3H, s, OCH3), 6.79–7.27 (3H, m, ArH), 8.03 (2H, s, NH2), 10.54 (1H, s, NH), 12.22 (1H, s, NH) ppm. 13C NMR (100 MHz, DMSO-d6): 10.13, 48.80, 56.69, 77.70, 96.82, 111.51, 120.32, 124.51, 125.93, 130.74, 133.96, 136.70, 142.52, 157.99, 162.33, 174.33 ppm. FT-IR (KBr): (vmax/cm−1): 3377 and 3294 (stretch NH), 3143 (stretch NH), 1702 (stretch C
O), 1676 (stretch C
O), 1612, 1550, 1473, 1207, 818, 660. Anal. calcd for C16H13Br N4O4: C, 47.43; H, 3.23; N, 13.83; Found: C, 47.39; H, 3.14; N, 13.90.
3.4.9 Methyl 6′-amino-3′-methyl-2-oxo-5-nitro-2′H-spiro[indoline-3,4′-pyrano[2,3-c]pyrazole]-5′-carboxylate (7d). Yellow solid; mp: 268–270 °C; 1H NMR (DMSO-d6, 400 MHz): δ 1.58–1.63 (3H, s, CH3), 3.25–3.33 (3H, s, OCH3), 7.03–8.13 (3H, m, ArH), 8.13 (2H, s, NH2), 11.15 (1H, s, NH), 12.28 (1H, s, NH) ppm. 13C NMR (100 MHz, DMSO-d6): 10.11, 48.83, 52.16, 74.78, 96.90, 111.04, 119.41, 126.71, 137.25, 137.50, 138.51, 144.16, 148.89, 155.77, 164.02, 173.16 ppm. FT-IR (KBr): (vmax/cm−1): 3398 and 3300 (stretch NH), 3145 (stretch NH), 1712 (stretch C
O), 1670 (stretch C
O), 1622, 1498, 1338, 1292, 694. Anal. calcd for C16H13N5O6: C, 51.76; H, 3.53; N, 18.86; Found: C, 51.72; H, 3.59; N, 18.75.
4. Conclusions
In conclusion, we have developed a straightforward and efficient method for the synthesis of spirooxindoles using magnetic nanoparticles Fe3O4-immobilized guanidine (MNPs-guanidine). The method offers several advantages including cleaner reaction profiles, use of easily available, high yields, shorter reaction times, simple experimental, reusability of the catalyst and little catalyst loading. This green nanocatalyst could be used for other significant organic reactions and transformations. We hope that this article will serve to stimulate research in this fascinating and very useful area of organic synthesis.
Acknowledgements
The authors are grateful to department of chemistry, Science and Research branch, Islamic Azad University, Tehran, Iran for supporting this work.
References
- M. Kitajima, J. Nat. Med., 2007, 61, 14–23 CrossRef CAS.
- R. Pandey, S. C. Singh and M. M. Gupta, Phytochemicals, 2006, 67, 2164–2169 CrossRef CAS PubMed.
- C. Pellegrini, M. Weher and H.-J. Borschberg, Helv. Chim. Acta, 1996, 79, 151–168 CrossRef CAS.
- G. Bhaskar, Y. Arun, C. Balachandran, C. Saikumar and P. T. Perumal, Eur. J. Med. Chem., 2012, 51, 79–91 CrossRef CAS PubMed.
- A. H. Abdel-Rahman, E. M. Keshk, M. A. Hanna and Sh. M. El-Bady, Bioorg. Med. Chem., 2004, 12, 2483–2488 CrossRef CAS PubMed.
- G. Wu, L. Ouyang, J. Liu, S. Zeng, W. Huang, B. Han, F. Wu, G. He and M. Xiang, Mol. Diversity, 2013, 17, 271–283 CrossRef CAS PubMed.
- G. Chen, H. P. He, J. Ding and X. J. Hao, Heterocycl. Commun., 2009, 15, 355–360 CrossRef CAS.
- A. S. Girgis, Eur. J. Med. Chem., 2009, 44, 1257–1264 CrossRef CAS PubMed.
- R. Murugan, S. Anbazhagan and S. S. Narayanan, Eur. J. Med. Chem., 2009, 44, 3272–3279 CrossRef CAS PubMed.
- T. L. Pavlovska, R. G. Redkin, V. V. Lipson and D. V. Atamanuk, Mol. Diversity, 2016, 20, 299–344 CrossRef CAS PubMed.
- S. Slobbe, E. Ruijter and R. V. A. Orru, Med. Chem. Commun., 2012, 3, 1189–1218 RSC.
- V. Estevez, M. Villacampa and J. C. Menendez, Chem. Soc. Rev., 2010, 39, 4402–4421 RSC.
- J. Safaei-Ghomi, A. Javidan, A. Ziarati and H. Shahbazi-Alavi, J. Nanopart. Res., 2015, 17, 338–350 CrossRef.
- D. Wang and D. Astruc, Chem. Rev., 2014, 114(14), 6949–6985 CrossRef CAS PubMed.
- A. Kiasat and J. Davarpanah, J. Mol. Catal. A: Chem., 2013, 373, 46–54 CrossRef CAS.
- J. Safaei-Ghomi, M. Navvab and H. Shahbazi-Alavi, Ultrason. Sonochem., 2016, 31, 102–106 CrossRef CAS PubMed.
- C. W. Lim and I. S. Lee, Nano Today, 2010, 5, 412–434 CrossRef CAS.
- M. Demirelli, E. Karaoglu, A. Baykal, H. Sozeri and E. Uysal, J. Alloys Compd., 2014, 582, 201–207 CrossRef CAS.
- M. B. Gawande, P. S. Branco and R. S. Varma, Chem. Soc. Rev., 2013, 42, 3371–3393 RSC.
- L. M. Rossi, N. J. S. Costa, F. P. Silva and R. Wojcieszak, Green Chem., 2014, 16, 2906–2933 RSC.
- A. H. Lu, E. L. Salabas and F. Schuth, Angew. Chem., Int. Ed., 2007, 46, 1222–1244 CrossRef CAS PubMed.
- A. Rostami, B. Atashkar and D. Moradi, Appl. Catal., A, 2013, 467, 7–16 CrossRef CAS.
- S. Y. Lee and M. T. Harris, J. Colloid Interface Sci., 2006, 293, 401–408 CrossRef CAS PubMed.
- A. Kumar and V. Kumar, Chem. Rev., 2014, 114, 7044–7078 CrossRef CAS PubMed.
- C. Chiu, L. Ruan and Y. Huang, Chem. Soc. Rev., 2013, 42, 2512–2527 RSC.
- F. Jérome, G. Kharchafi, I. Adam and J. Barrault, Green Chem., 2004, 6, 72–74 RSC.
- C. Alonso-Moreno, A. Antinolo, F. Carrillo-Hermosilla and A. Otero, Chem. Soc. Rev., 2014, 43, 3406–3425 RSC.
- E. A. Faria, H. F. Ramalho, J. S. Marques, P. A. Z. Suarez and A. G. S. Prado, Appl. Catal., A, 2008, 338, 72–78 CrossRef CAS.
- J. Safaei-Ghomi, F. Eshteghal and H. Shahbazi-Alavi, Ultrason. Sonochem., 2016, 33, 99–105 CrossRef CAS PubMed.
- R. Mrowczynski, A. Nan and J. Liebscher, RSC Adv., 2014, 4, 5927–5952 RSC.
- S. Ahadi, H. R. Khavasi and A. Bazgir, Chem. Pharm. Bull., 2008, 56, 1328–1330 CrossRef CAS PubMed.
- R. Ghahremanzadeh, F. Fereshtehnejad, Z. Yasaei, T. Amanpour and A. Bazgir, J. Heterocycl. Chem., 2010, 47, 967–972 CrossRef CAS.
- S. Ahadi, Z. Yasaei and A. Bazgir, J. Heterocycl. Chem., 2010, 47, 1090–1094 CrossRef CAS.
- M. MirhosseiniMoghaddam, A. Bazgir, M. M. Akhondi and R. Ghahremanzadeh, Chin. J. Chem., 2012, 30, 709–714 CrossRef CAS.
- G. Mohammadi Ziarani, A. Badiei, S. Mousavi, N. Lashgari and A. Shahbazi, Chin. J. Catal., 2012, 33, 1832–1839 CrossRef CAS.
- B. Karmakar, A. Nayak and J. Banerji, Tetrahedron Lett., 2012, 53, 5004–5007 CrossRef CAS.
- H. Li, H. Chen, C. Shi, D. Shi and S. Ji, J. Comb. Chem., 2010, 12, 231–237 CrossRef PubMed.
- J. Yu, Y. Zhou, T. Shen, W. Mao, K. Chen and Q. Song, J. Chem. Res., 2013, 37, 365–368 CrossRef CAS.
- J. Feng, K. Ablajan and A. Sali, Tetrahedron, 2014, 2, 484–489 CrossRef.
- R. Y. Guo, Z. M. An, L. P. Mo, S. T. Yang, S. X. Wang and Z. H. Zhang, Tetrahedron, 2013, 47, 9931–9938 CrossRef.
- Y. Zou, Y. Hu, H. Liu and D. Shi, ACS Comb. Sci., 2012, 14, 38–43 CrossRef CAS PubMed.
- A. Khalafi-Nezhad, E. S. Shahidzadeh, S. Sarikhani and F. Panahi, J. Mol. Catal. A: Chem., 2013, 379, 1–8 CrossRef CAS.
- F. Alemi-Tameh, J. Safaei-Ghom, M. Mahmoudi-Hashemi and R. Teymuri, Res. Chem. Intermed., 2016, 42, 6391–6406 CrossRef CAS.
- K. Petcharoen and A. Sirivat, Mater. Sci. Eng., B, 2012, 177, 421–427 CrossRef CAS.
Footnote |
† Electronic supplementary information (ESI) available. See DOI: 10.1039/c6ra08458c |
|
This journal is © The Royal Society of Chemistry 2016 |
Click here to see how this site uses Cookies. View our privacy policy here.