DOI:
10.1039/C6RA08267J
(Paper)
RSC Adv., 2016,
6, 58394-58400
Fluorescence turn-on detection of Fe3+ in pure water based on a cationic poly(perylene diimide) derivative†
Received
31st March 2016
, Accepted 1st June 2016
First published on 2nd June 2016
Abstract
Polycondensation of perylene diimide (PDI) diamines with a 2,2′:6′,2′′-terpyridine (tpy)-containing dibromide gives a new fluorescent polymeric chemosensor (L) with good water solubility. L exhibits good selectivity for Fe3+ ions over a wide range of tested metal ions Ag+, K+, Na+, Fe2+, Cu2+, Al3+, Cr3+, Mn2+, Mg2+, Ni2+, Ca2+, Hg2+, Pd2+, Ba2+, Cd2+, Co2+ and Zn2+ in aqueous solution. The binding of Fe3+ to chemosensor L leads to significant emission bands at 548 nm and 591 nm, respectively, because the complexation of Fe3+ with the tpy moiety of L blocks the photoinduced electron transfer (PET) from tpy units to PDI units. A 1
:
2 stoichiometry was found for the complex formed by L and Fe3+ by Job's plot. The association constant (Ka) of Fe3+ binding in chemosensor L is 1.32 × 108 M−2. L can be used to detect Fe3+ over a wide pH range of 5.0–9.0. Moreover, by taking advantage of its instant fluorescence turn-on specific to Fe3+, L is used as a fluorescent probe to monitor the Fe3+/Fe2+ transition in the presence of reducing agents such as Na2S2O3 or ascorbic acid (Vc) in water. L can also work well in biological samples, which gives access to the detection of Fe3+ in an organism.
Introduction
Iron is the most abundant transition metal in the human body and plays a crucial role in many biological processes such as oxygen transportation,1 electron transfer2 and enzymatic catalysis.3 However, both excess with respect to and deficiency from the normal permissible limit can induce serous disorders and diseases.4 The considerable importance of iron in biological and environmental systems has led to increasing interest in recent years in the development of selective techniques for the determination of iron. A variety of analytical techniques such as atomic absorption spectroscopy,5 electrochemisty,6 spectrophotometry7 and inductively coupled plasma mass spectrometry8 have been adopted for sensitive iron determination, but these methods have limitations such as sophisticated instrumentation, and complicated pretreatment procedures due to other metal ion interference. Recently, fluorescence-based techniques have gained extensive attention for the detection of iron because of their ability to provide a simple, sensitive, selective, relatively low-cost method for online monitoring without any pretreatment of the sample.9,10 However, because of the paramagnetic nature of Fe3+ ions, to date, most reported fluorescent Fe3+ sensors are based on a fluorescence quenching mechanism.10 In addition, most of the reported Fe3+ sensors are insoluble or only partly soluble in water.10 Thus in those sensing system the detection of Fe3+ ions usually must be performed in organic solvents and water mixture. As well known, in contrast with fluorescence “turn-off” sensors, those operate in “turn-on” mode can reduces the likelihood of false positive signals and increase the sensitivity as demonstrated by numerous studies. Moreover, water solubility of sensors is essential for interfacing with biological substrates such as proteins and DNA etc. Therefore for practical applications especially in biological system, it is still necessary to develop Fe3+ sensors that are easily synthesized, and possess good water-solubility as well as selective and sensitive fluorescence turn-on signal output.
Photoinduced electron transfer (PET) has been widely used to design turn-on chemosensors for various ions detection.11 In such strategy, the free electron pair of a functional group attached to the fluorophore may quench its fluorescence intramolecularly due to a PET process. Metal coordination to the group renders it a less efficient electron donor, thereby interrupting the PET process. Thus, the native fluorescence of the fluorophore is restored. As an attractive class of chromophores, perylene-3,4,9,10-tetracarboxylic acid diimides (perylene diimides, PDIs) have been widely used as photonic materials, because of their high extinction coefficients, high fluorescence quantum yields, a broad color range, as well as outstanding chemical, thermal, and photochemical stability in organic solvents.12 PDIs are also good electron acceptors with low reduction potential.13 Therefore PDIs should be promising candidates for application as fluorophores in development of fluorescent turn-on chemosensors based on PET strategy. On the other hand, 2,2′:6′,2′′-terpyridine (tpy) groups not only can selectively coordinate with different metal ions,14 but also can act as good electron donor when PDIs are excited and thus quench the fluorescence of the PDIs.15 The binding of metal ions in the tpy unit will block the PET between tpy and PDI and thus restore the fluorescence of PDI. So, it is reasonable for one to design a new fluorescence turn-on chemosensors for Fe3+ based on PET process by combining PDIs with tpy groups.
However, PDIs exhibit usually poor water solubility and the tendency to form aggregates in water due to intrinsic π–π stacking interactions between perylene backbones, resulting in the further decrease of solubility in water.16 Great efforts have been made to increase the water solubility of PDIs by introducing hydrophilic groups, such as cationic ammonium salts, anionic carboxylic acids, sulfonic acids etc., in the bay-region or at the imide- and ortho-positions.16 Inspired by those outstanding work, we designed and synthesized a new PDIs-based polymeric chemosensor (L) (Scheme 1), in which PDIs units in the backbone are used as fluorophores and 2,2′:6′,2′′-terpyridine (tpy) groups connected to the side chain are used as both the metal ion receptor and electron donor. The cationic ammonium salts located in the main chain ensure good water-solubility of chemosensor L. From Scheme 1, the working principle of L is described as following: in the absence of metal ions, free L displays weak fluorescence emission due to the efficient electron transfer between tpy unit and PDI unit. Upon coordination of L with Fe3+, the PET process is blocked, resulting in the fluorescence of L is restored. As expected, L exhibits good selectivity and high sensitivity for Fe3+ ions over relevant competing metal ions in aqueous solution. A fluorescence turn-on ratio over 40-fold was triggered under saturated conditions. Further, L is shown to be a promising potential fluorescent probe for facilely monitoring the Fe3+/Fe2+ transition in the presence of different reducing agents such as Na2S2O3 or ascorbic acid (Vc) in water. L can also be applied to detect Fe3+ in living cells.
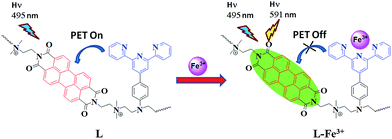 |
| Scheme 1 Illustration of fluorescence turn-on detection of Fe3+ based on a PDIs-based chemosensor. | |
Experimental section
Materials and instruments
The salts of iron used were FeCl3·6H2O and FeCl2·4H2O, respectively, and other cations in the form of nitrate salts were used for UV-vis and fluorescence spectroscopy. 3,4,9,10-Perylenetetracarboxylic dianhydride were purchased from Alfa Aesar Co. and used without further purification. All other chemicals used were local products of analytical grade. The water used throughout all experiments was purified by a Millipore filtration system. The polymer concentration was calculated based on the repeat unit.
General characterization
1H NMR and 13C NMR spectra were collected on a Bruker-400 NMR spectrometer (400 MHz) using tetramethylsilane (TMS) as an internal standard. The pH measurements were carried out on a Mettler-Toledo Delta 320 pH meter. The absorption spectrum was recorded on a Shimadzu UV-2550 spectrometer. Fluorescence measurements were carried out on a Hitachi F-4600 fluorescence spectrophotometer equipped with a xenon lamp excitation source. Cell image was performed on a Confocal Laser Scanning Microscope FV-1000 (Olympus, Japan).
Synthesis of 4′-p-[N,N-bis(2-hydroxyethyl)amino]phenyl-2,2′:6′,2′′-terpyridine (1)
Compound 1 was synthesized according to the procedure reported in literature.17 2-Acetylpyridine (1.16 g, 9.60 mmol) was added into a solution of 4-[N,N-bis(2-hydroxyethyl)-amino]benzaldehyde (1.00 g, 4.80 mmol) in EtOH (25 mL). KOH pellets (0.54 g, 9.60 mmol) and aq. NH3 (25 mL, 29.3%, 20 mmol) were then added to the solution. The solution was stirred at r.t. for 12 h. The mixture was refluxed for the next 4 h and was then evaporated. Recrystallization from Et2O–EtOH (20
:
1) afforded yellow solid 1 (0.79 g, 1.93 mmol, 39.6%). 1H NMR (400 MHz, d-DMSO, ppm) δ: 8.77 (d, J = 4 Hz, 2H), 8.64 (d, J = 8 Hz, 4H), 8.07–7.97 (m, J = 8 Hz, 2H), 7.77 (t, J = 4 Hz, 2H), 7.57–7.47 (dd, J = 6 Hz, 2H), 6.88 (d, J = 8 Hz, 2H), 4.86 (s, 2H), 3.63 (t, J = 6 Hz, 4H), 3.53 (t, J = 4 Hz, 4H). 13C NMR (101 MHz, d-DMSO, ppm) δ: 155.78, 149.65, 137.83, 128.08, 124.79, 123.60, 121.32, 116.63, 112.25, 58.54, 53.59, 40.39, 39.93, 39.45, 39.25. HRMS calcd for C25H25N4O2: [M + H+] 413.1978, found 413.1964. MP: 216–217 °C.
Synthesis of 4′-p-[N,N-bis(2-bromineethyl)amino]phenyl-2,2′:6′,2′′-terpyridine (2)
Compound 2 was synthesized according to the reported method.18 The bis-hydroxy starting material 1 (100 mg, 0.24 mmol) from the previous step was dissolved in 30 mL of CH2Cl2, and the solution was cooled to 0 °C in an ice bath and brought under N2 atmosphere. To this stirred solution was added PBr3 (100 μL, 1.05 mmol) dropwise via syringe. During the next 2 h, the reaction temperature was maintained at 0 °C, and a sticky yellow precipitate was observed. The reaction was allowed to come to room temperature over 4 h. Next, the reaction was quenched by addition of 10 mL of water at 0 °C, and then neutralized to pH 7.0 with a saturated solution of Na2CO3. The organic layer was separated from the aqueous phase, dried over Na2SO4, and the solvent removed under reduced pressure. The product was purified via silica gel chromatography and eluted with CH2Cl2/hexanes (v/v 1
:
1) to afford a yellow solid. Yield: 120.2 mg (92%). 1H NMR (400 MHz, d-DMSO, ppm) δ: 8.78 (d, 2H), 8.66 (d, 4H), 8.04 (m, 2H), 7.80 (t, 2H), 7.53 (dd, 2H), 6.91 (d, 2H), 3.62 (s, 4H), 3.53 (s, 4H). 13C NMR (101 MHz, d-DMSO, ppm) δ: 155.83, 155.59, 149.69, 147.72, 137.95, 128.53, 125.72, 124.88, 121.41, 117.08, 112.81, 52.23, 40.65, 40.44, 40.23, 40.02, 39.81, 39.60, 39.40, 30.25. HRMS calcd for C25H23N4Br2: [M + H+] 539.0270, found 539.0283. MP: 163–164 °C.
Synthesis of N,N-bis[diethylamine]-3,4,9,10-perylenetetra-carboxylic diimide (3)
Compound 3 was synthesized according to the reported method.19 A suspension of 3,4,9,10-perylenetetracarboxylic dianhydride (200 mg, 0.51 mmol) and 2-dimethylaminoethyl-amine (0.34 mL, 5.10 mmol) in DMF (10 mL) was heated under stirring for 5 h to 130 °C. After cooling to room temperature, 50 mL THF were added and the resulting precipitate was collected by suction filtration and washed with 3 × 10 mL of THF. Drying in the vacuum oven at 40 °C overnight yielded the title compound as a dark purple solid 3 (247.0 mg, 0.46 mmol, 91%). 1H NMR (400 MHz, d-TFA, ppm) δ: 8.50 (d, J = 8.3 Hz, 4H), 8.45 (d, J = 8.0 Hz, 4H), 4.48 (t, J = 5 Hz, 4H), 3.55–3.38 (t, J = 5 Hz, 4H), 2.98–2.81 (s, 12H). HRMS calcd for C32H29N4O4: [M + H+] 533.2189, found 533.2191. MP: >300 °C.
Synthesis of polymer (L)
A suspension of monomer 3 (99.0 mg, 0.19 mmol) and other monomer 2 (100.0 mg, 0.19 mmol) in 10 mL of DMF was heated under stirring to 100 °C for 12 h. After cooling to room temperature, 100 mL of THF were added and the resulting precipitate was collected by suction filtration and washed with 3 × 100 mL of THF. After drying in the vacuum oven at 40 °C overnight, the crude product was dissolved in H2O. Then the solution was dialyzed through a membrane with a molecular weight cutoff of 3500 for three days and freeze-dried to yield a dark red solid (60.4 mg, 0.056 mmol, 30.4%). 1H NMR (400 MHz, d-TFA, ppm) δ: 8.70 (t, J = 19.8 Hz, 14H), 8.50 (dd, J = 19.4, 13.9 Hz, 8H), 4.47 (d, J = 11.8 Hz, 4H), 3.49 (t, J = 32.5 Hz, 4H), 3.21–2.65 (d, 12H). 13C NMR (101 MHz, d-TFA, ppm) δ: 160.02, 155.97, 155.54, 155.11, 154.67, 130.18, 126.96, 123.17, 118.24, 115.36, 112.47, 109.66, 106.84, 104.02, 52.09, 37.79, 30.08. FT-IR (KBr, cm−1): 2957 (C–H stretching vibration), 1693 (C–N stretching vibration), 1654 (C
O stretching vibration), 1592 (stretching vibration of C
N and C
C in pyridine ring), 1347 (C–N stretching vibration), 809 (out-of-plane bending vibration of C–H in benzene ring).
Results and discussion
Polymer synthesis and characterization
Chemosensor L was obtained following the synthetic methodology shown in Scheme 2. The Menshutkin reaction of ditertiary amines (3) with dihalides (2) gives a new linear cationic polymer (L), which shows good solubility in water (about 11.2 mg mL−1) due to the cationic ammonium salts in the polymeric backbone. The structure of chemosensor L was confirmed using NMR and FT-IR. Moreover, the chemical structure analysis results for the monomers 2 and 3 coincide well with theoretical calculations, reflecting the high purity of the polymer. The detailed characterization results were presented in ESI (Fig. S1–S10†). As expected, L itself exhibits very weak fluorescence with the fluorescence quantum yield (Φ) of 0.07, which was determined in water in reference to rhodamine (Φf = 0.69 in methanol).20
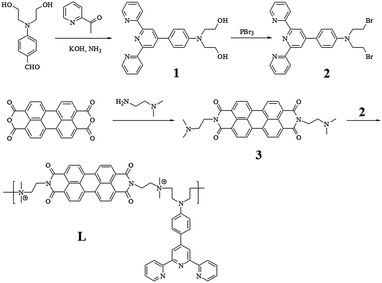 |
| Scheme 2 Synthetic route and chemical structures of L. | |
Absorption spectra response to Fe3+
UV-vis spectra titration of L was performed by means of stepwise addition of Fe3+ and recording a spectrum after each step (Fig. 1). As shown in Fig. 1, L (10 μM) in water exhibited a very weak absorption band at 499 nm and 531 nm, respectively (absorption coefficient: ε = 590 M−1 cm−1), which are typical spectroscopic features of PDI units.21 Upon addition of Fe3+, the corresponding absorbance peaks at 499 and 535 nm increased smoothly, which indicated the formation of a new complex between L and Fe3+. A dependence of the absorbance at 499 nm as a function of [Fe3+]/[L] was presented in inset of Fig. 1, and after a linear increase of the absorption with the concentration, the equivalence point was reached at a about 1
:
2 ratio of [Fe3+]/[L], indicating that the pendant tpy groups were coordinated with Fe3+ ions completely. However, the characteristic metal-to-ligand charge-transfer (MLCT) band located at 575 nm for tpy–iron(III) complex,22 cannot be observed, which should be attributed to its relatively weak absorbance and be overlapped. In order to confirm the rationality of the explanation, the absorption spectra of monomer 2 (20 μM) with tpy groups in absence and presence of Fe3+ ions in water were recorded and presented in Fig. S11.† As shown in Fig. S11,† MLCT band at 575 nm with ε = 1850 M−1 cm−1 is indeed very weak even under saturated conditions, i.e., the addition of 4 equiv. of Fe3+ ions.
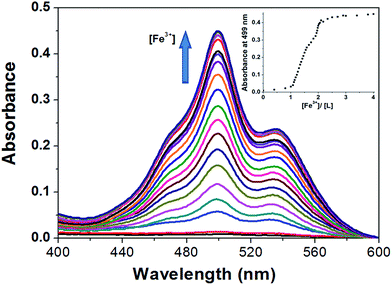 |
| Fig. 1 UV-vis spectra of L (10 μM) in water with increasing amounts of Fe3+ (0–4 equiv.). Inset: absorbance of L at 499 nm as a function of [Fe3+]/[L]. | |
To evaluate the selectivity of chemosensor L, a variety of other relevant cations were also added into its aqueous solution. As shown in Fig. S12,† only a very weak increase of absorbance at 499 nm and 535 nm was observed with 5 equiv. of Hg2+, Fe2+ and Pd2+, and no obvious response could be observed upon the addition of other ions. But, addition of the same amount of Fe3+ into the aqueous solution of L, immediately induced a significant absorbance enhancement (about 56-fold) at 499 nm and 531 nm. This showed the good selectivity of L toward Fe3+ over other completing metal ions.
Fluorescent spectra response to Fe3+
Fig. 2 shows the fluorescence spectra of L and those in the presence of different concentrations of Fe3+. The free chemosensor L (4 μM) exhibited a very weak fluorescence at 548 and 591 nm in water, respectively. This indicates PET effect from electron donors of tpy units to fluorophore of PDI units is retained in chemosensor L. The titration of Fe3+ with L led to a rapid increase of the emission intensity at 548 and 591 nm. Over 40-fold fluorescence enhancement was observed under saturated condition (ca. 3.8 equiv. of Fe3+). The increase of the fluorescence intensity at 548 nm followed the sigmoidal curves and the fluorescence turn-on constant (Kturn-on) was calculated according to the method reported in literature23 as 8.98 ± 0.23 μM with correlation coefficient R = 0.994 (Fig. S13†). From the changes in Fe3+-dependent fluorescence intensity at 548 nm (inset, Fig. 2), the detection limit was estimated to be 9.2 × 10−7 M based on 3 × S0/S, where S0 is the standard deviation of background and S is the sensitivity.24
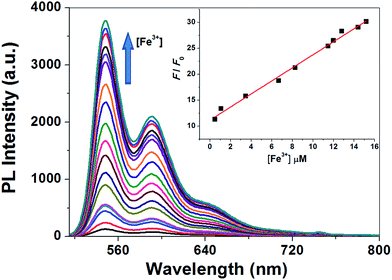 |
| Fig. 2 Fluorescent titration spectra of L (4 μM) in water in the presence of different concentrations of Fe3+ (0–3.8 equiv.). Inset: the fluorescence intensity ratio (F/F0) at 548 nm as a function of the Fe3+ concentration. λex = 495 nm. | |
To determine the binding stoichiometry of the L–Fe3+ complex, the emission intensity of chemosensor L at 548 nm was plotted as a function of Fe3+ molar fraction under a constant total concentration. The resulting Job's plot was shown in Fig. S14.† The maximum emission intensity was reached at a molar ratio of about 0.7. This indicates a 1
:
2 ratio for the L–Fe3+ complex, which is consistent with the results of UV-vis spectra titration of L with Fe3+. The association constant Ka of the L–Fe3+ complex was evaluated graphically by plotting 1/(F − F0) against 1/[Fe3+]2.15 The data were linearly fitted to the Benesi–Hildebrand equation and the Ka value was obtained from the slope and intercept of the line. The association constant (Ka) for Fe3+ binding in chemosensor L was determined to be 1.32 × 108 M−2.
Selectivity
Various metal ions were used to evaluate the binding properties and the selectivity of L by means of fluorescence spectra in water (Fig. 3). Fig. 3 shows the corresponding fluorescent spectra of L in water (2 μM) after addition of different metal ions (5 equiv.). The emission peaks appear at about 548 nm and 591 nm, which are the typical emission of PDI without any significant peak shifts. The result suggests that no other interaction between the tpy unit and PDI unit exists in the excited state besides the efficient electron transfer between both units in the absence of metal coordination.15 As shown in Fig. 3, the most distinctive fluorescence intensity enhancement resulted when Fe3+ was added. Addition of Al3+ and Cr3+ enhanced the fluorescence intensity of L too, but by an obviously much smaller magnitude. The presence of Mn2+, Hg2+, Cu2+ and Fe2+ only resulted in slight fluorescence intensity changes under identical experimental conditions.
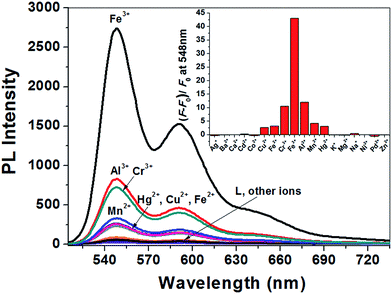 |
| Fig. 3 Fluorescence spectra of L (2 μM) in water in the presence of several relevant metal ions (5 equiv. for each metal ion). Inset: histogram representing the fluorescence enhancement or quenching of L in the presence of metal ions. λex = 495 nm. | |
The fluorescence intensity enhancements are usually described by the enhancement factor (EF), which is calculated from the fluorescence intensities of the chemosensor L with (F) or without metal ions (F0) (inset in Fig. 3). In the absence of metal ions, the fluorescence of L was strongly quenched by the PET process. But in the presence of Fe3+, the fluorescence intensity of L increased by over 43-fold. The EFs of Al3+ and Cr3+ calculated following the same method were 12 and 10, respectively. L thus show “turn-on” fluorescence, and exhibits a quite remarkably high selectivity for Fe3+ among the various ions under study in water. Due to the tpy unit is capable of forming stable chelate complexes with a variety of transition metal ions,14 most of reported tpy-based chemosensors to date display good selectivity for target ion in organic solvent or organic/water mixed solvent, but with a certain degree of interference from the other related ions, as observed in our system. The selectivity of chemosensor L for Fe3+ could be further improved by increasing the length of the spacer between tpy unit and PDI unit, resulting in more effective PET process. This work is just under way and will be reported in the near future.
To explore the possibility of using L as a practical ion-selective fluorophore for Fe3+, competition experiments were carried out, in which L (2 μM) was firstly mixed with 5 equiv. of various metal ions including Ag+, Al3+, Ba2+, Ca2+, Cd2+, Co2+, Cr3+, Cu2+, Fe2+, Hg2+, K+, Mg2+, Na+, Ni2+, Pb2+ and Zn2+ followed by addition of 5 equiv. of Fe3+ (Fig. 4). Emission spectroscopy was used to monitor the competition events. As shown in Fig. 4, in the presence of the above-mentioned ions, chemosensor L still has a good turn-on ratio for the detection of Fe3+. Similar fluorescence enhancement was observed for L after the addition of Fe3+ salts with NO3− as counter anion. The fluorescence responses of L and L + Fe3+ in acidic and basic regions of the pH range were examined, which showed that L can be used within a wide pH span of 5.0–9.0 (Fig. S15†).
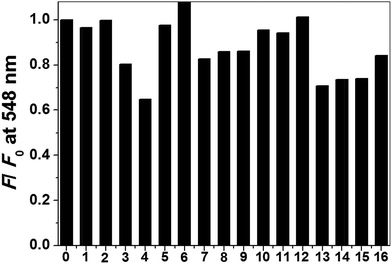 |
| Fig. 4 Change ratio (F/F0) of fluorescence intensity of L (2 μM) in water at 548 nm in various mixtures of metal ions FeCl3 (10 μM) and one other metal ion (10 μM). 0: Fe3+; 1: Fe3+ + Ag+; 2: Fe3+ + Al3+; 3: Fe3+ + Ba2+; 4: Fe3+ + Ca2+; 5: Fe3+ + Cd2+; 6: Fe3+ + Co2+; 7: Fe3+ + Cr3+; 8: Fe3+ + Cu2+; 9: Fe3+ + Fe2+; 10: Fe3+ + Hg2+; 11: Fe3+ + K+; 12: Fe3+ + Mg2+; 13: Fe3+ + Na+; 14: Fe3+ + Ni2+; 15: Fe3+ + Pb2+; 16: Fe3+ + Zn2+. λex = 495 nm. | |
Conversion of iron from Fe3+ to Fe2+
Since the specific Fe3+-amplified fluorescence of L is slightly affected by the presence of Fe2+, the Fe3+/Fe2+ transition monitoring was realized by determining the fluorescence of L. As shown in Fig. 5A, free L (2.5 μM) showed weak fluorescence emission in water, however, upon the addition of 10 μM of Fe3+, the fluorescent intensity increased immediately by 42-fold. When the aliquots of sodium thiosulfate (Na2S2O3) aqueous solution (stock solution concentration of 0.5 M) was added the above mixture, the emission decreased almost instantly upon each addition (Fig. 5B), suggesting the reduction of Fe3+ to Fe2+ by Na2S2O3. The emission spectra almost turned back to that of L–Fe2+ complex after 5 μL of Na2S2O3 solution was added. Control experiment of L without Fe3+ demonstrated the addition of Na2S2O3 did not cause any evident change in the emission. So, the results indicated that L possesses potential as probes to monitor the conversion between valence states of iron.
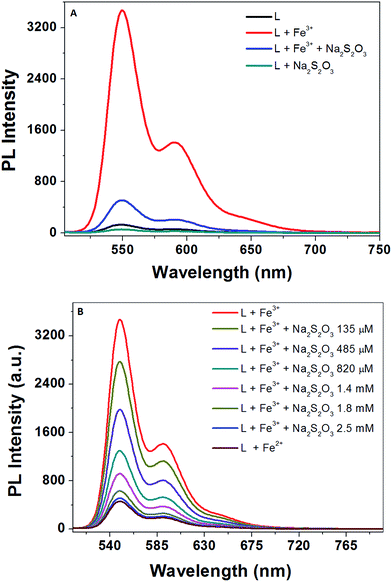 |
| Fig. 5 Redox conversion of iron from Fe3+ to Fe2+ dependence of the fluorescence intensity of L (2.5 μM) in water. (A) from top to bottom: free L; L in the presence of 4 equiv. of Fe3+; the mixed solution of L and 4 equiv. of Fe3+ was treated further with 12.5 μL of Na2S2O3 aqueous solution (0.5 M); L was only added 12.5 μL of Na2S2O3 aqueous solution (0.5 M). (B) from top to bottom: L in the presence of 4 equiv. of Fe3+; the mixed solution of L and 4 equiv. of Fe3+ was titrated by Na2S2O3 aqueous solution (the corresponding concentrations of Na2S2O3 were 135 μM, 485 μM, 820 μM, 1.4 mM, 1.8 mM and 2.5 mM, respectively); L in the presence of 4 equiv. of Fe2+. λex = 495 nm. | |
Furthermore, the kinetic assay of redox conversion of iron from Fe3+ to Fe2+ in the presence of ascorbic acid (Vc) was performed in water (Fig. S16†). The fluorescence of the complex of L (2.5 μM) with Fe3+ (10 μM) was remarkably quenched upon the addition of ascorbic acid (0.83 mM) in about 2 minute. It demonstrated the probe could instantly and quickly respond to Fe3+ concentration fluctuations which was reduced by ascorbic acid. Additional work is also being done to use L as fluorescent probe for the detection of Fe3+ and Fe3+/Fe2+ transition as well as imaging in living cells, which will be reported in the upcoming work.
Cell imaging
Practical application ability is also an important factor to judge a probe. Since the positively charged structure of L is favorable for cell membrane permeability, the living cell fluorescence imaging experiment was conducted to preliminarily investigated whether L could penetrated the cell membrane to detect Fe3+ in living cells. As shown in Fig. 6, when pancreas islet Ins-1 cells were incubated with L (2 μM) for 45 min at 37 °C, the cells showed a weak green fluorescence (Fig. 6a and c). After being treated with Fe3+ (10 μM) for 15 min at 37 °C, the cells showed strong fluorescence (Fig. 6d and f). The changes in the images of pancreas islet Ins-1 cells revealed that L could probe Fe3+ in the biological samples. The preliminary results of cytotoxicity assays of L show that L is not toxic to cells within the range of 0–20 μM. The results of these experiments indicate that as a probe for Fe3+, L can work in a relatively complex environment and has the potential to detection Fe3+ in biological samples.
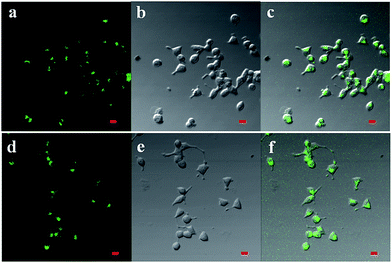 |
| Fig. 6 Fluorescence images of Ins-1 cells incubated with L (2 μM) for 45 min at 37 °C ((a) from the dark field; (b) from the bright field; (c) overlapped image of (a and b)) and further treated with Fe3+ (10 μM) for 15 min at 37 °C ((d) from the dark field; (e) from the bright field; (f) overlapped image of (d and e)). Excitation: 488 nm; collection: 536 to 608 nm. The red scale bar is 10 μm. | |
Conclusions
In summary, a new water-soluble PDIs-based polymeric fluorescent chemosensor (L) for Fe3+ was devised and synthesized via integration of the Fe3+-chelating tpy groups with PDI fluorophores. Fluorescence turn-on sensing platform for the detection of Fe3+ in pure water was successfully developed based on PET mechanism. L shows good sensitivity and selectivity in water toward Fe3+ over other interfering metal ions. Moreover, the Fe3+/Fe2+ transition at the μM level can be monitor using the sensor upon irradiation by taking advantage of the different fluorescent response to Fe3+ and Fe2+. More importantly, L can be applied to detect Fe3+ in living cells. Considering the multitude of the choices for the structure modification of PDI, we are confident that these results presented here will contribute to the rational design of more excellent probes for Fe3+ in the future.
Acknowledgements
This study was supported by the National Natural Science Foundation of China (No: 51373122, 21074093), the Program for New Century Excellent Talents in University (NCET-12-1066) and 131 talents program of Tianjin.
Notes and references
- P. Aisen, M. Wessling-Resnick and E. A. Leibold, Curr. Opin. Chem. Biol., 1999, 3, 200 CrossRef CAS PubMed; M. D. Connie and C. W. Hsia, N. Engl. J. Med., 1998, 338, 239 CrossRef PubMed.
- D. J. A. Netz, M. Stümpfig, C. Doré, U. Mühlenhoff, A. J. Pierik and R. Lill, Nat. Chem. Biol., 2010, 6, 758 CrossRef CAS PubMed; M. B. Murataliev, R. Feyereisen and F. A. Walker, Biochim. Biophys. Acta, 2004, 1698, 1 CrossRef PubMed; M. F. Lucas, D. L. Rousseau and V. Guallar, Biochim. Biophys. Acta, 2011, 1807, 1305 CrossRef PubMed.
- R. S. Eisenstein, Annu. Rev. Nutr., 2000, 20, 627 CrossRef CAS PubMed; T. A. Rouault, Nat. Chem. Biol., 2006, 2, 406 CrossRef PubMed.
- J. D. Haas and T. Brownlie, J. Nutr., 2001, 131, 676S CrossRef CAS PubMed.
- A. Ohashi, H. Ito, C. Kanai, H. Imura and K. Ohashi, Talanta, 2005, 65, 525 CrossRef CAS PubMed.
- V. A. Elrod, K. S. Johnson and K. H. Coale, Anal. Chem., 1991, 63, 893 CrossRef CAS.
- Z. O. Tesfaldet, J. F. van Staden and R. I. Stefan, Talanta, 2004, 64, 1189 CrossRef CAS PubMed.
- K. Akatsuka, J. W. McLaren, J. W. Lam and S. S. Berman, J. Anal. At. Spectrom., 1992, 7, 889 RSC.
- B. Valeur and I. Leray, Coord. Chem. Rev., 2000, 205, 3 CrossRef CAS.
- F. Petrat, U. Rauen and H. De-Groot, Hepatology, 1999, 29, 1171 CrossRef CAS PubMed; X. Qu, Q. Liu, X. Ji, H. Chen, Z. Zhou and Z. Shen, Chem. Commun., 2012, 48, 4600 RSC; X. Wu, B. Xu, H. Tong and L. Wang, Macromolecules, 2010, 43, 8917 CrossRef; G. Zhang, B. Lu, Y. Wen, L. Lu and J. Xua, Sens. Actuators, B, 2012, 171–172, 786 CrossRef; P. Li, C. Ji, H. Ma, M. Zhang and Y. Cheng, Chem.–Eur. J., 2014, 20, 5741 CrossRef PubMed.
- B. Daly, J. Ling and A. P. de Silva, Chem. Soc. Rev., 2015, 44, 4203 RSC.
- M. R. Wasielewski, J. Org. Chem., 2006, 71, 5051 CrossRef CAS PubMed; J. A. A. W. Elemans, R. van Hameren, R. J. M. Nolte and A. E. Rowan, Adv. Mater., 2006, 18, 1251 CrossRef; H. Langhals, Helv. Chim. Acta, 2005, 88, 1309 CrossRef; F. Würthner, Chem. Commun., 2004, 1564 RSC.
- J. Feng, B. Liang, D. Wang, L. Xue and X. Li, Org. Lett., 2008, 10, 4437 CrossRef CAS PubMed; C. Zhao, Y. Zhang, R. Li, X. Li and J. Jiang, J. Org. Chem., 2007, 72, 2402 CrossRef PubMed; W. Xu, H. Chen, Y. Wang, C. Zhao, X. Li, S. Wang and Y. Weng, ChemPhysChem, 2008, 9, 1409 CrossRef PubMed; B. A. Jones, M. J. Ahrens, M.-H. Yoon, A. Facchetti, T. J. Marks and M. R. Wasielewski, Angew. Chem., Int. Ed., 2004, 43, 6363 CrossRef PubMed; K. Muthukumaran, R. S. Loewe, C. Kirmaier, E. Hindin, J. K. Schwartz, I. V. Sazanovich, J. R. Diers, D. F. Bocian, D. Holten and J. S. Lindsey, J. Phys. Chem. B, 2003, 107, 3431 CrossRef; C. Kirmaier, E. Hindin, J. K. Schwartz, I. V. Sazanovich, J. R. Diers, K. Muthukumaran, M. Taniguchi, D. F. Bocian, J. S. Lindsey and D. Holten, J. Phys. Chem. B, 2003, 107, 3443 CrossRef.
- S. Bhowmik, B. Nath Ghosh, V. Marjomäki and K. Rissanen, J. Am. Chem. Soc., 2014, 136, 5543 CrossRef CAS PubMed; P. Mahato, S. Saha and A. Das, J. Phys. Chem. C, 2012, 116, 17448 CrossRef; M. Chiper, M. A. R. Meier, D. Wouters, S. Hoeppener, C.-A. Fustin, J.-F. Gohy and U. S. Schubert, Macromolecules, 2008, 41, 2771 CrossRef; R. Dobrawa and F. Würthner, Chem. Commun., 2002, 1878 RSC; R. Dobrawa, M. Lysetska, P. Ballester, M. Grüne and F. Würthner, Macromolecules, 2005, 38, 1315 CrossRef; M. Zheng, H. Tan, Z. Xie, L. Zhang, X. Jing and Z. Sun, ACS Appl. Mater. Interfaces, 2013, 5, 1078 Search PubMed; J. Wang, X. Li, X. Lu, Y.-T. Chan, C. N. Moorefield, C. Wesdemiotis and G. R. Newkome, Chem.–Eur. J., 2011, 17, 4830 CrossRef PubMed.
- H. Wang, D. Wang, Q. Wang, X. Li and C. A. Schalley, Org. Biomol. Chem., 2010, 8, 1017 Search PubMed.
- D. Görl, X. Zhang and F. Würthner, Angew. Chem., Int. Ed., 2012, 51, 6328 CrossRef CAS PubMed; M. Sun, K. Müllen and M. Yin, Chem. Soc. Rev., 2016, 45, 1513 RSC.
- J. Wang and G. S. Hanan, Synlett, 2005, 1251 CAS.
- Q. Dong, M. J. Rose, W.-Y. Wong and H. B. Gray, Inorg. Chem., 2011, 50, 10213 CrossRef CAS PubMed.
- F. Biedermann, E. Elmalem, I. Ghosh, W. M. Nau and O. A. Scherman, Angew. Chem., Int. Ed., 2012, 51, 7739 CrossRef CAS PubMed.
- R. A. Velapoldi and H. H. Tonnesen, J. Fluoresc., 2004, 14, 465 CrossRef CAS PubMed.
- L. Zhong, F. Xing, Y. Bai, Y. Zhao and S. Zhu, Spectrochim. Acta, Part A, 2013, 115, 370 CrossRef CAS PubMed.
- H. Hofmeier and U. S. Schubert, Macromol. Chem. Phys., 2003, 204, 1391 CrossRef CAS.
- P. Du and S. J. Lippard, Inorg. Chem., 2010, 49, 10753 CrossRef CAS PubMed.
- B. Zhu, F. Yuan, R. Li, Y. Li, Q. Wei, Z. Ma, B. Du and X. Zhang, Chem. Commun., 2011, 47, 7098 RSC.
Footnote |
† Electronic supplementary information (ESI) available. See DOI: 10.1039/c6ra08267j |
|
This journal is © The Royal Society of Chemistry 2016 |