DOI:
10.1039/C6RA07666A
(Communication)
RSC Adv., 2016,
6, 41947-41950
Light upconverting soft particles: triplet–triplet annihilation in the phospholipid bilayer of self-assembled vesicles†
Received
23rd March 2016
, Accepted 19th April 2016
First published on 20th April 2016
Abstract
Large unilamellar vesicles (100 nm) were functionalised to obtain supramolecular particles capable of light upconversion in pure aqueous media. Triplet–triplet annihilation of diphenylanthracene (DPA) leading to delayed fluorescence at 420 nm was used to up-convert the light absorbed by embedded metal complex sensitisers. The lipid type and the chromophore position in the membrane affect the TTA intensity, which remains constant over a large concentration range.
Introduction
Increasing the efficiency of light harvesting is an important task in natural photosynthesis as well in industrial solar systems. For some applications, high energies or short irradiation wavelength are required.1 Therefore, up-converting (UC) processes producing photons of higher energy by combination of low energy photons have been developed.2 Different systems showing upconversion are known, but most of them provide only low quantum yields or require specific conditions.3 In recent years, significant effort was put into the development of triplet–triplet annihilation (TTA) upconversion processes and their applications.
In this process low energy photons are absorbed by sensitizers, which after intersystem crossing (ISC) perform triplet–triplet energy transfer (TTET) with an annihilator dye. Two annihilator molecules then undergo TTA and singlet emission is observed (Fig. 1).4,5 TTA upconversion was used in sensing,6 in flexible LCDs7 and in bioimaging.8 The process has some advantages in comparison with other UC processes as it works with low excitation intensities and provides a relatively high quantum yield.9 Many pairs of sensitizers and annihilators were found to exhibit TTA in organic solvents,10 polymers,11,12 dendrimers13 and other supramolecular systems.14,15 However, most dyes used for TTA are lipophilic and only soluble in organic solvents. TTA systems operating in aqueous media use upconverting nano-capsules, micellar solutions or giant unilamellar vesicles.16–19 As the preparation of such water soluble TTA systems can be laborious we present here a supramolecular self-assembly approach based on large vesicles (100 nm) with embedded dyes exhibiting TTA (Fig. 2). In this system, platinum porphyrin PtOEP and an amphiphilic derivative of tris-(bipyridine)ruthenium(II) 1 are used as sensitizers. They are co-embedded in the membrane and appear inside (PtOEP) or on the outer part of the bilayer (1). Lipophilic diphenyl-anthracene DPA used as an annihilator is present inside the bilayer. Two other amphiphilic derivatives of DPA (DPA-COOH, DPA-PEG, Fig. 2) were examined in order to test the effect of the position of the annihilator in the membrane.
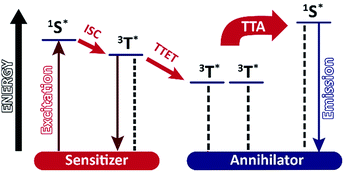 |
| Fig. 1 Schematic representation of the triplet–triplet annihilation process. | |
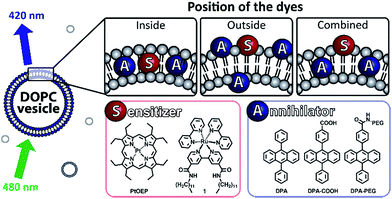 |
| Fig. 2 Used dyes and their assembly in the upconverting DOPC vesicles. | |
Results and discussion
The porphyrin complex PtOEP is a known TTA sensitizer.20 The amphiphilic sensitizer 1 was previously prepared in our group and used in vesicular systems.21 It co-embeds well in the membrane and creates stable vesicles with a variety of lipids. DPA was chosen as annihilator for its ability to perform triplet–triplet energy transfer with both sensitizers.11 Due to its molecular structure it neither forms photodimers, nor excimers upon excitation.11,22 Combinations of sensitizers and annihilators were introduced to DOPC vesicles to study the behaviour of the TTA. Vesicles were prepared according to known procedures and loading of the compounds co-embedded in the membrane did not exceed 11 mol%. All prepared vesicles were used fresh and were stable more than 24 h (ESI, Fig. S3†). We used a OPO pumped by a Nd:YAG laser to excite the sensitizers (PtOEP: 532 nm, 1: 480 nm) and recorded the observed delayed fluorescence of annihilator with a Streak Camera.23
TTA inside the bilayer
A recent report by Askes et al. shows that the confinement inside the DOPC bilayer does not affect TTA between palladium complex and perylene.16 Our selected pair of dyes operates at shorter wavelengths. Both PtOEP and DPA are lipophilic compounds and locate inside the phospholipid bilayer. A strong delayed fluorescence of DPA is observed when the sensitizer is excited (Fig. 3).
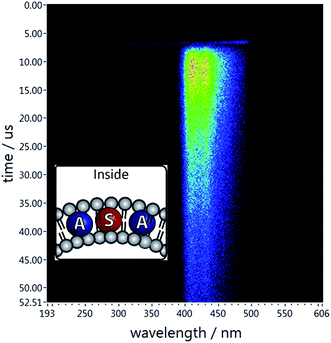 |
| Fig. 3 Delayed emission of DPA when both dyes are co-embedded inside the bilayer. The system contains DPA (0.06 mM), PtOEP (0.02 mM) in aq. DOPC vesicles (0.92 mM). | |
TTA with the sensitizer on the surface of the membrane
Sensitizer 1 is amphiphilic and expected to assemble with its polar head group at the surface of the bilayer. We examined TTA using DPA and 1 in water, in methanol and in aqueous solution containing DOPC vesicles (Fig. 4). When the same amounts of dyes were used in the vesicular solution, the absorbance of DPA was drastically reduced by 50% in comparison to the same concentrations in methanol (Fig. 4, left). This indicates that high local DPA concentration inside the bilayer result in the formation of larger aggregates, which are still present in vesicular solution, but do not absorb light. We studied the absorption and emission spectra of DPA in the vesicular systems (ESI, Fig. S2†) and observed the highest absorption intensity for 4 mol% loading of DPA, which corresponded also to the highest fluorescence emission intensity (Fig. 4, left).‡ With membrane loadings exceeding 4 mol%, the shape and intensity of the emission spectra change, which might be explained by the formation of aggregates in the membrane. This was confirmed by experiments where 3 volume equivalents of methanol were added to the vesicular solutions to burst and dissolve the vesicles, which restored the original fluorescence intensity of the chromophores (ESI, Fig. S4†).
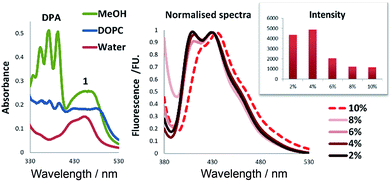 |
| Fig. 4 Left: UV-Vis spectra of different systems containing DPA (0.06 mM) and 1 (0.02 mM) in aq. DOPC vesicles (0.92 mM) (blue), plain Milli-Q water (red) or methanol (green). Right: Fluorescence (λex = 374 nm) of DOPC (0.89–0.97 mM) vesicles with different molar loading of DPA in presence of 1 mol% of 1 (0.01 mM). Intensity integrated from 390 nm to 580 nm. | |
For sensitizer 1, the differences of the photophysical properties between homogeneous solution and embedded in the membrane were much less pronounced (Fig. 4). We used these optimised concentrations of sensitizer and annihilator and recorded TTA in vesicles and solutions (Fig. 5).
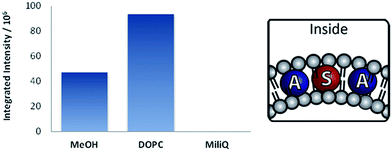 |
| Fig. 5 TTA intensity§ in vesicles containing DPA (0.06 mM) and 1 (0.02 mM) in aq. DOPC vesicles (0.92 mM), Milli-Q water or methanol. | |
DPA is negligibly soluble in water (ESI, Fig. S1†). Due to this fact, no delayed fluorescence is observed in aqueous solution (Fig. 5). The mixture was prepared in the same manner as vesicular solutions except the absence of the lipid. If lipids are present, a delayed fluorescence due to TTA is observed with twofold intensity compared with the methanol solution of the same concentration (Fig. 5). This can be attributed to higher local concentration of the dyes involved in TTA.
In order to increase the intensity of TTA, often a large excess of the annihilator is used to improve the efficiency of the triplet–triplet energy transfer to annihilator molecules. Therefore, we prepared vesicular solutions varying the ratio of DPA from 2 to 10 equivalents, respective to the sensitizer 1, which concentration was kept constant. Only a small increase in intensity is achieved by changing from 2 to 4 eq. of DPA (2 to 4%). Larger excess of DPA leads to significantly reduced emission intensities (Fig. 6). This corresponds well with effect of aggregation observed on fluorescence intensity.
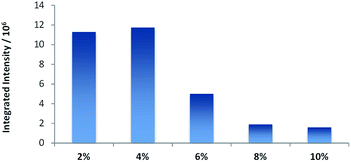 |
| Fig. 6 Intensity§ of TTA in DOPC (0.89–0.97 mM) vesicles with different molar loading of DPA in presence of 1 mol% of 1 (0.01 mM). | |
TTA on the surface of the membrane
During TTA upconversion two intermolecular processes (TTET, TTA) take place. The close proximity of the dyes plays a key role in its efficiency. In order to assemble all dyes at the water lipid interface, we prepared two derivatives of DPA bearing a polar head group (Scheme 1).
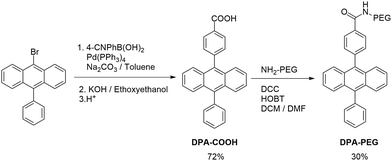 |
| Scheme 1 Synthesis of DPA amphiphiles. | |
The carboxylic acid in DPA-COOH serves as a small polar head group, which was further functionalized with a polyethylene glycol chain (Mr = 5000) giving DPA-PEG. Both compounds showed similar absorption and emission properties (ESI, Fig. S5†).
When used as previously, DPA derivatives with sensitizer 1 yielded stable 100 nm vesicles (ESI, Fig. S6†). The TTA intensity is clearly enhanced when amphiphilic DPA derivatives are used in DOPC vesicles (Fig. 7).
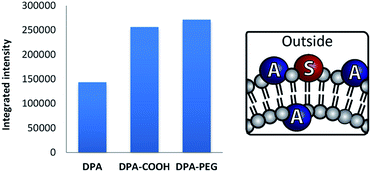 |
| Fig. 7 Intensity§ of delayed fluorescence in vesicles containing DPA or derivatives (0.06 mM) and 1 (0.02 mM) in DOPC vesicles (0.92 mM) in Milli-Q water. | |
Different lipids
The membrane mobility within vesicles depends on the chemical nature of the lipids and is characterized by the transition temperature between gel and liquid phase. Below the transition temperature the lateral movement of membrane embedded compounds is inhibited. Under the same conditions as for DOPC (Tm = −17 °C) we tested DSPC (Tm = 55 °C) and DMPC (Tm = 24 °C). Only low (DMPC) or no (DSPC) TTA was observed in comparison with DOPC (Fig. 8). DSPC-based membranes are rigid as a result of the high transition temperature of the lipid. This is known to induce phase separation and clustering of molecules on the membrane surface and similar effects might be expected inside the bilayer. DMPC on the other hand is a lipid, which should give at room temperature a similarly fluid membrane as DOPC, but the intensity of TTA is proportionally lower at the same DPA loadings. The shape of fluorescence spectra indicate that DPA behaves in DMPC membrane similar as in DOPC at higher loadings (ESI, Fig. S7†) and forms aggregates. It can be concluded that a fluid membrane is essential for effective TTA in vesicle membranes.
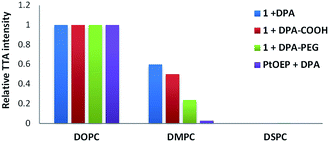 |
| Fig. 8 Relative intensity of TTA depending on the nature of the vesicle lipid (0.95 mM). The vesicles are functionalised with DPA derivatives (0.04 mM) and sensitizers (0.01 mM). For each TTA system the intensity§ in DOPC vesicles is used as 100% value. | |
Effect of dilution
In homogenous TTA systems, dilution leads to a nonlinear decrease of the intensity of delayed fluorescence of DPA due to the lower rate of TTET and TTA. Contrary, in the vesicular membrane the local concentration of the dyes does not change significantly upon dilution of the solution; only the overall concentration of the vesicles decreases. Therefore, the upconverting system is functionally independent on the concentration and emission intensity decreases linearly upon dilution (Fig. 9). Fitting of the time resolved emission measurements with a simple model described in the ESI† shows no significant change in the dynamics of the system. The rate constant for the decay of the sensitizer k1 was in the range of 4.99 to 5.20 × 104 s−1 and the rate constant for the decay of the annihilator showed values from 1.44 to 1.62 × 106 s−1.
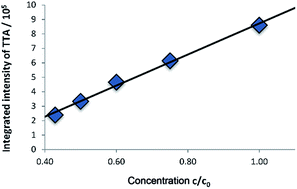 |
| Fig. 9 Integrated intensity§ of TTA for different dilutions of the solution shows linear dependency. Starting concentration c0 (concentration of all amphiphiles) of the solution: DOPC (0.93 mM) vesicles functionalised with 1 (0.01 mM) and DPA (0.06 mM) in Milli-Q water. | |
Conclusions
Functionalized DOPC vesicles exhibiting TTA were prepared by co-embedding dyes in different positions in the phospholipid bilayer of the vesicles. Three derivatives of the annihilator were used with two sensitizers. The vesicles are stable and exhibit twice as intense TTA compared to a methanolic solution of the dyes at identical concentrations. The ratios between sensitizer and annihilator dye and their respective concentrations in the membrane were optimized to yield the best TTA efficiency. Fluidity of the membrane is essential for effective TTA of the embedded dyes. Solutions of the functionalized vesicles can be diluted retaining their molar intensity of TTA, allowing their use at low concentrations for imaging applications. Additional functionalization of the outer membrane surface for specific applications can be readily envisaged.
The simple preparation by self-assembly and easy variations of type and ratio of dyes makes visible light upconverting functionalized vesicles a valuable alternative to TTA in homogeneous solution.
Notes and references
- X. Huang, S. Han, W. Huang and X. Liu, Chem. Soc. Rev., 2013, 42, 173–201 RSC
.
- G. S. He, L.-S. Tan, Q. Zheng and P. N. Prasad, Chem. Rev., 2008, 108, 1245–1330 CrossRef CAS PubMed
.
- R. Scheps, Prog. Quantum Electron., 1996, 20, 271–358 CrossRef CAS
.
- T. N. Singh-Rachford and F. N. Castellano, Coord. Chem. Rev., 2010, 254, 2560–2573 CrossRef CAS
.
- J. A. Briggs, A. C. Atre and J. A. Dionne, J. Appl. Phys., 2013, 113, 124509 CrossRef
.
- S. M. Borisov, C. Larndorfer and I. Klimant, Adv. Funct. Mater., 2012, 22, 4360–4368 CrossRef CAS
.
- M. Tzenka, Y. Vladimir, N. Gabriele and B. Stanislav, New J. Phys., 2008, 10, 103002 CrossRef
.
- Q. Liu, T. Yang, W. Feng and F. Li, J. Am. Chem. Soc., 2012, 134, 5390–5397 CrossRef CAS PubMed
.
- S. Baluschev, V. Yakutkin, T. Miteva, G. Wegner, T. Roberts, G. Nelles, A. Yasuda, S. Chernov, S. Aleshchenkov and A. Cheprakov, New J. Phys., 2008, 10, 013007 CrossRef
.
- J. Zhao, S. Ji and H. Guo, RSC Adv., 2011, 1, 937–950 RSC
.
- P. C. Boutin, K. P. Ghiggino, T. L. Kelly and R. P. Steer, J. Phys. Chem. Lett., 2013, 4, 4113–4118 CrossRef CAS
.
- Y. C. Simon and C. Weder, J. Mater. Chem., 2012, 22, 20817–20830 RSC
.
- K. Tanaka, K. Inafuku and Y. Chujo, Chem. Commun., 2010, 46, 4378–4380 RSC
.
- K. Katta, D. Busko, Y. Avlasevich, R. Muñoz-Espí, S. Baluschev and K. Landfester, Macromol. Rapid Commun., 2015, 36, 1084–1088 CrossRef CAS PubMed
.
- P. Ceroni, Chem.–Eur. J., 2011, 17, 9560–9564 CrossRef CAS PubMed
.
- S. H. C. Askes, N. L. Mora, R. Harkes, R. I. Koning, B. Koster, T. Schmidt, A. Kros and S. Bonnet, Chem. Commun., 2015, 51, 9137–9140 RSC
.
- T. Andrey, B. Dmitry, B. Stanislav, M. Tzenka and L. Katharina, New J. Phys., 2011, 13, 083035 CrossRef
.
- J.-H. Kim and J.-H. Kim, J. Am. Chem. Soc., 2012, 134, 17478–17481 CrossRef CAS PubMed
.
- S. H. C. Askes, M. Kloz, G. Bruylants, J. T. M. Kennis and S. Bonnet, Phys. Chem. Chem. Phys., 2015, 17, 27380–27390 RSC
.
- P. B. Merkel and J. P. Dinnocenzo, J. Lumin., 2009, 129, 303–306 CrossRef CAS
.
- M. Hansen, F. Li, L. Sun and B. Konig, Chem. Sci., 2014, 5, 2683–2687 RSC
.
-
K. K. Rohatgi-Mukherjee, Fundamentals of Photochemistry, Wiley, 1978 Search PubMed
.
- R.-J. Kutta, T. Langenbacher, U. Kensy and B. Dick, Appl. Phys. B, 2013, 111, 203–216 CrossRef CAS
.
Footnotes |
† Electronic supplementary information (ESI) available. See DOI: 10.1039/c6ra07666a |
‡ The compared fluorescence emission intensities belong to systems with different absorption spectra. |
§ The intensity is the sum of all counting events from 2 μs after excitation to the end of the measurement from 365 to 480 nm. |
|
This journal is © The Royal Society of Chemistry 2016 |
Click here to see how this site uses Cookies. View our privacy policy here.