DOI:
10.1039/C6RA07144A
(Paper)
RSC Adv., 2016,
6, 48515-48522
A robust process for lipase-mediated biodiesel production from microalgae lipid
Received
18th March 2016
, Accepted 8th May 2016
First published on 10th May 2016
Abstract
Microalgae lipid has been considered a good feedstock for biodiesel production because of its well-recognized advantages. To promote microalgae as a feedstock for biodiesel production, developing an appropriate method to convert the lipid into biodiesel is significant. A novel process with the combination of free lipase and immobilized lipase used for the conversion of microalgae lipid for biodiesel production is proposed in this paper. The combination of the two lipases was demonstrated to be effective in converting free fatty acids into biodiesel (FAME). The effect of different factors influencing immobilized lipase-catalyzed methanolysis was investigated systematically. It was demonstrated that the strategy of adding methanol, molecular sieve dosage, lipase dosage and temperature had significant influence on the FAME yield. Under the optimized conditions (10% 3 Å molecular sieve dosage (w/w), molar ratio of methanol to lipid 5
:
1, lipase dosage 5% (w/w), temperature 45 °C and agitation rate 300 rpm), a biodiesel yield of 97% could be obtained. Further, a 5-level-4-factor central composite design was employed to optimize immobilized lipase-mediated alcoholysis and the relationships between the variables and their co-influence on biodiesel yield were further analyzed systematically.
1. Introduction
As a renewable energy source, biodiesel has been regarded as a good substitute for petroleum. In the US and European Union countries, refined oils like soybean oil, cottonseed oil and rapeseed oil are major feedstocks for the production of biodiesel.1 However, such edible oils compete with the food supply and face serious challenges, especially for the sustainable development of the biodiesel industry. Recently, microalgae has been thought to be a potential choice to solve the dispute between the food and fuel industries. Some species of microalgae have been considered as a new generation of feedstock to produce biodiesel because of their high lipid content, easy cultivation and high growing rates.2–7 Recently, lipase has been widely investigated as a catalyst for biodiesel preparation for its environmental friendliness and efficacy in converting both triglycerides and free fatty acids (FFAs) into FAME.8–10
Immobilized lipase has been widely used to produce biodiesel because the product can be easily purified, the lipase can be easily reused and the good thermal and pH stability of the lipase can be maintained. However, previous research showed that the by-product glycerol had a poor solubility in renewable oils and subsequently generated a negative effect on lipase’s activity.11,12 Organic solvents are usually needed in the system to avoid the aforementioned inactivation.8 Recently, it has been demonstrated that free lipase can efficiently catalyze biodiesel production in an oil/water two-phase system and there is no need to add organic solvents. Compared to immobilized lipase, free lipase has several merits like a higher reaction rate, lower cost and lower requirements for the feedstock.1,9,10,13 However, in free lipase-mediated alcoholysis for biodiesel production in an oil/water two-phase system, free fatty acids (FFAs) are generated through the hydrolysis of triglycerides, leading to high content of FFAs in the product.14
To combine the advantages of free lipase and immobilized lipase together, a novel two-step process is proposed in this paper. In the first step, free lipase was adopted to catalyze microalgae lipid into FAME in a water/oil system. Around 80% conversion could be completed through this step and most of the by-product glycerol would be generated in this step and remain in the water phase, waiving the negative influence on the subsequent reaction catalyzed by immobilized lipase. In the second-step, immobilized lipase was used to realize the rest of the conversion in a solvent-free system and FFA could be converted effectively to FAME in this catalytic step. Since the negative effect caused by the by-product glycerol was removed, the immobilized lipase could retain rather good stability even in the solvent-free system. In the whole process, no organic solvent is adopted and the risk of deactivation of the lipase caused by methanol is also reduced significantly.
Further, a 5-level-4-factor central composite design was employed to optimize the immobilized lipase-mediated alcoholysis, including the levels of the molar ratio of methanol to lipid, lipase dosage, temperature and agitation rate. The relationships between the variables and their co-influence on the 6 h FAME yield were further analyzed systematically.
2. Experimental
2.1. Materials
Chlorella protothecoides was provided by ENN (China). Free lipase NS81006 (from Aspergillus niger, activity 3300 U mL−1) and Novozym 435 (immobilized lipase from Candida antarctica, type B, activity 10
000 PLU per g) were provided from Novo Industries (Denmark). One unit of lipase activity (LU) is defined as the amount of lipase that produces 1 μmol of free fatty acid per minute. Heptadecanoic acid methyl ester as GC standard and 14% BF3 (w/v) in methanol were purchased from Sigma-Aldrich (St. Louis, MO). Other reagents of analytical grade were obtained commercially.
2.2. Lipid extraction method
The pretreatment of the microalgae was performed in a 2 L single-neck round bottom flask equipped with a serpentine condenser tube, and a mixture of 100 g microalgae, 311.3 mL 88% (w/w) formic acid solution, 27.3 mL 37% (w/w) HCl solution and 261.5 mL water was put into the flask and heated under a boiling state for 30 min.
The microalgae lipid was extracted by using a modified version of Bligh and Dyer’s method.15,16 After the aforementioned pretreatment, the mixture was filtrated with a funnel, and the solids were transferred into a 2 L round bottom flask and then the solids were subjected to a re-extraction with 1 L chloroform, 100 mL methanol and 400 mL water for 30 s at 1500 rpm. The solvent layers were combined together and then evaporated to remove the existence of the organic solvents. Finally, the obtained lipid was washed with water until neutral pH was reached.
2.3. Enzyme catalysis procedure
2.3.1. Free lipase-catalyzed methanolysis for biodiesel production. The free lipase catalyzed process was carried out in a 500 mL three-neck round bottom flask equipped with a mechanical stirrer at 1300 rpm and immersed in a thermostat water bath of 45 °C for 6 h. The reaction mixture comprised 100 g algae lipid, 10 g Tris–HCl buffer (pH = 7.8), 20 mL methanol and 3 mL free lipase NS81006. The stepwise methanol addition strategy is described as follows: 35%, 20%, 15%, 15%, 10% and 5% of the total methanol volume was added into the reaction mixture at 1 h intervals from 0 h to 5 h. Samples were taken from the reaction mixture at specified times and centrifuged to get the lipid layer for GC analysis.
2.3.2. Immobilized lipase-catalyzed methanolysis for biodiesel production. The immobilized lipase catalyzed process was carried out in a 50 mL conical flask and placed in a thermostatic shaker at 40–50 °C for 6 h. The reaction mixture comprised lipid phase (5 g), molecular sieves of type 3 Å and Novozym 435. Samples were taken from the reaction mixture at specified times for further analysis. For HPLC analysis, the sample was dissolved in acetone, and after being shaken thoroughly, 20 μL sample was taken for diacylglyceride and monoglyceride analysis.
2.4. FAME analysis
The effective lipid can be defined as “fatty acids and derivatives”, which contains triacylglycerols, phospholipids, glycolipids and free fatty acids.6,7 It is noted that not all types of lipid extracted from microalgae can be transformed into FAME, such as pigments.15 Therefore, it is necessary to measure the effective lipid content which can be converted to FAME. The effective lipid FAME content can be measured using the standard procedure AOAC 991.39 (Association of Analytical Communities). The specific procedure is described as follows: 25 mg crude lipid, 2 mg heptadecanoic acid methyl ester (internal standard) and 1.5 mL NaOH (0.5 mol L−1) in methanol were put into a glass tube and well-shaken, the mixture was then heated to 100 °C for 15 min. After cooling down, 2 mL 14% BF3 (w/v) in methanol was added to the glass tube and the mixture was further heated to 100 °C for 30 min. After being cooled down to room temperature, 5 mL saturated NaCl solution and 1 mL hexane were added into the mixture which was agitated thoroughly followed by standing to allow it to become layered. The upper hexane layer of 1 μL was injected for further GC analysis.
The GC analysis was carried out by an Agilent 7890A GC system (Agilent Technologies, Santa Clara, USA) equipped with a CP-FFAP CB capillary column (25 m × 0.32 mm × 0.30 μm, Agilent Technologies, USA). The initial column temperature was set to 180 °C and maintained for 0.5 min, then heated to 250 °C at the rate of 10 °C min−1 and held for 6 min. The detector and injector temperatures were set to 250 °C and 245 °C, respectively.
The FAME yield is defined as follows:
FAME yield (%) = reaction mixture FAME content/effective lipid FAME content × 100%. |
2.5. Diacylglyceride and monoglyceride analysis
The analysis for diacylglyceride and monoglyceride was performed on a Shimadzu 20A HPLC, ELSD-LT II equipped with a C18 reversed-phase column. The temperature of the column and detector was set at 45 °C and 70 °C, respectively, with the hydrogen pressure kept at 320 kPa. The mobile phase was acetonitrile–acetic acid and dichloromethane with the elution program listed in Table 1. The diacylglyceride and monoglyceride content was calculated by the standard curve obtained by an external standard.
where Amono and Adia are the peak areas of monoglyceride and diacylglyceride respectively. msample is the weight of the oil phase (mg).
Table 1 HPLC elution program
Time (min) |
Flow rate (1 mL min−1) |
Dichloromethane (v/v, %) |
Acetonitrile–acetic (99.85 : 0.15) (v/v, %) |
0 |
1.50 |
0 |
100 |
4 |
1.50 |
0 |
100 |
12 |
1.50 |
10 |
90 |
25 |
1.50 |
10 |
90 |
30 |
1.50 |
30 |
70 |
35 |
1.50 |
30 |
70 |
45 |
1.50 |
80 |
20 |
55 |
1.50 |
80 |
20 |
60 |
1.50 |
0 |
100 |
65 |
1.50 |
0 |
100 |
2.6. Determination of the acid value in the lipid
An acid value is used to determine the content of free fatty acid in a lipid phase, which is defined as the mass of KOH in mg that is required to neutralize 1 g of a sample. The acid value was measured using the following procedure: 1–2 g sample was dissolved in 25 mL ethanol, after stirring thoroughly, 3 drops of phenolphthalein was added as indicator. The sample was then subject to titration with NaOH standard solution until the solution turns red. The acid value was calculated with the following formula:
where V is the volume of NaOH solution consumed (mL), n is the NaOH solution concentration (mol L−1) and m is the sample weight (g).
2.7. Response surface design and evaluation for immobilized lipase-catalyzed methanolysis
The influence of the molar ratio of methanol to lipid, lipase dosage, temperature and bed shaking speed was investigated by response surface methodology and the 5-level-4-factor central composite design was adopted. Table 1 shows the factors and Table 2 shows the experimental design as well as the related values. The FAME yield at 6 h was chosen as the response variable for a statistical study in the quadratic model to describe relationships between the response variable and the reaction conditions. The quadratic model was regressed as follows: |
Yyield = λ0 + ∑λiXi + ∑λiiXi2 + ∑λijXiXj
| (1) |
where Yyield is the FAME yield at 6 h. λ0, λi, λii and λij are the regression constants of the quadratic model and Xi and Xj (i = 1, 2, 3, 4; j = 1, 2, 3, 4; i ≠ j) represent the independent variables in code form. The regression of the quadratic polynomial model was conducted by the automatic regression algorithm “Backward” provided by Design-Expert (Stat-Ease, Inc, 8.0.6.1).
Table 2 Variables and experimental design levels for the response surface
|
|
Levels |
−2 |
−1 |
0 |
1 |
2 |
X1 |
Molar ratio of methanol to lipid |
3 |
4 |
5 |
6 |
7 |
X2 |
Lipase dosage (w/w, %) |
1 |
2 |
3 |
4 |
5 |
X3 |
Temperature (°C) |
40 |
42.5 |
45 |
47.5 |
50 |
X4 |
Agitation rate |
200 |
225 |
250 |
275 |
300 |
3. Results and discussion
3.1. Free lipase catalyzed methanolysis
It has been found that free lipase can efficiently catalyze soybean oil for biodiesel production in an oil/water two-phase system.1 Free lipase-mediated methanolysis is characterized by a high reaction rate, low cost and low requirements for the feedstock. Herein, free lipase-mediated biodiesel production from microalgae lipid was investigated. It can be seen from Fig. 1 that a FAME yield of 78% could be obtained at 10 h, while the diacylglyceride and monoglyceride content were very low at 21 h (data not shown). Fig. 1 also shows the change profile of the acid value during the free lipase-mediated biodiesel production process.
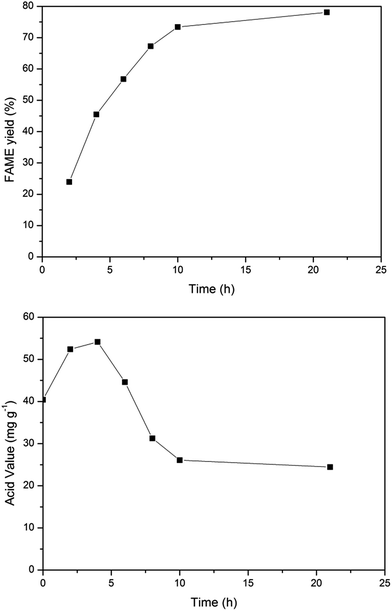 |
| Fig. 1 Free lipase-catalyzed methanolysis FAME yield from Chlorella protothecoides. Reaction conditions: 100 g lipid, pH = 7.8 Tris–HCl buffer 10 g, temperature 45 °C, lipase dosage 3% (w/w) and agitation rate 1300 rpm. | |
It can be seen that the acid value increased first and then decreased. The original acid value of the microalgae lipid was measured as 41.2 mg KOH per g oil and while the reaction proceeded the acid value increased which was followed by a rapid decrease and finally, the acid value reached around 25 mg KOH per g oil. The change in acid value is due to the following fact: the lipase-mediated hydrolysis of microalgae lipid occurred in an oil/water two-phase system resulting in the generation of FFAs, which lead to the initial increase in the acid value. Lipase also can catalyze the esterification of FFAs with methanol, which is why the acid value decreased as the reaction proceeded.
It can be seen that in this free lipase-catalyzed process around 80% conversion is completed and most of the glycerol by-product would be generated in this step and remain in the water phase. To further realize the thorough conversion of the microalgae lipid, immobilized lipase was adopted for the second-step catalysis in a solvent-free system and the FFAs can be converted to FAME accordingly.
3.2. Immobilized lipase catalyzed methanolysis
3.2.1. Effect of the addition strategy of methanol. Considering that the addition of methanol may influence the lipase’s activity, the effect of the methanol addition strategy was investigated. Fig. 2 shows that the methanol addition strategy had a significant influence on the methanolysis process and the FAME yield. It could be noticed that the inactivation of the lipase occurred with the strategy of adding methanol once. The strategy with methanol added at 0 h, 2 h and 4 h gave the highest biodiesel yield.
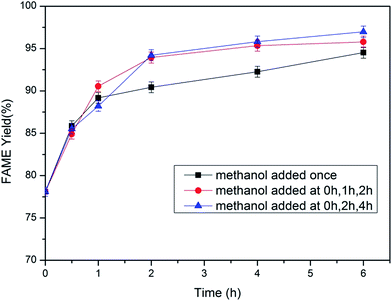 |
| Fig. 2 Effect of the addition strategy of methanol on FAME yield from Chlorella protothecoides. Reaction conditions: 10% (w/w) molecular sieve, molar ratio of methanol to lipid 5 : 1, temperature 45 °C, lipase dosage 3% (w/w) and agitation rate 250 rpm. | |
3.2.2. Effect of the molecular sieve dosage. It has been found that the water content in the Novozym-435-mediated methanolysis needs to be controlled at a low level to get a high yield of biodiesel.17,18 Herein, a molecular sieve is added to the system to remove the water produced in the reaction. A molecular sieve of type 3 Å is used as the water adsorbent. The initial water content is measured as 1.01% (w/w). It was found that when 10% (based on lipid weight) of the molecular sieve was added in the system, the highest biodiesel yield could be achieved (Fig. 3).
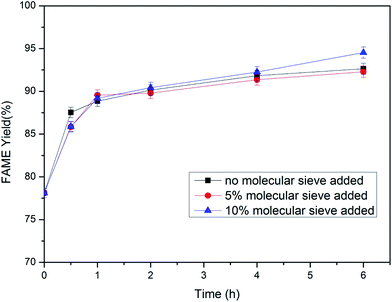 |
| Fig. 3 Effect of a molecular sieve on the immobilized lipase-mediated methanolysis. Reaction conditions: methanol added once, molar ratio of methanol to lipid 5 : 1, temperature 45 °C, lipase dosage 3% (w/w) and agitation rate 250 rpm. | |
3.2.3. Effect of the molar ratio of methanol to lipid. As methanol has a better solubility in FAME than in oil, the risk of lipase deactivation caused by methanol present in the system can be reduced to a certain extent. It can be observed from Fig. 4 that the molar ratio of methanol to lipid had little effect on the FAME yield. There was only about a 2% difference in the FAME yield when the molar ratio was 7
:
1 and 5
:
1 at 6 h. However, when too much methanol is added, it may influence the activity of the lipase. To maintain a high activity of the lipase, the molar ratio of methanol to lipid of 5
:
1 is the best for the second-step conversion.
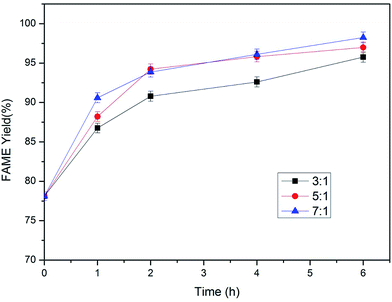 |
| Fig. 4 Effect of the molar ratio of methanol to lipid on the FAME yield from Chlorella protothecoides. Reaction conditions: methanol added at 0 h, 2 h, 4 h, 10% (w/w) molecular sieve added, temperature 45 °C, lipase dosage 3% (w/w) and agitation rate 250 rpm. | |
3.2.4. Effect of lipase dosage. The lipase dosage has a direct impact on the reaction rate. Fig. 5 indicates that the FAME yield increased significantly as the lipase dosage increased. The lipase dosage showed a greater influence on the FAME yield in the first 2 hours, while the reaction was nearly in equilibrium as it proceeded to 4 hours. It can be also seen from the figure that the equilibrium FAME yield at 5% (w/w) lipase dosage is higher than that at 3% (w/w) and 1% (w/w). So 5% (w/w) lipase dosage is needed for the process as it can reach equilibrium faster and with a higher FAME yield.
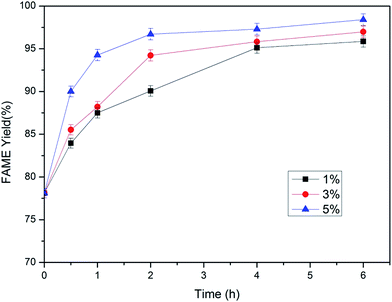 |
| Fig. 5 Effect of lipase dosage on FAME yield from Chlorella protothecoides. Reaction conditions: methanol added at 0 h, 2 h, 4 h, 10% (w/w) molecular sieve added, temperature 45 °C, molar ratio of methanol to lipid 5 : 1 and agitation rate 250 rpm. | |
3.2.5. Effect of temperature. As the immobilized lipase needs more activation energy to change conformation, it is more high-temperature resistant than the lipase without immobilization.17 Herewith, experiments were carried out to investigate the effect of temperature ranging from 40 °C to 50 °C on the FAME yield (Fig. 6). It can be seen that between 40 °C and 50 °C the catalytic efficiency showed a trend of rising first then falling. When the reaction temperature was at 45 °C, the FAME yield was 5–6% higher than when the reaction temperature was at 40 °C or 50 °C.
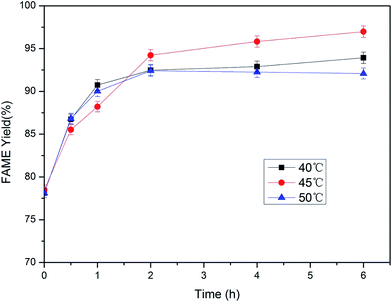 |
| Fig. 6 Effect of temperature on the FAME yield from Chlorella protothecoides. Reaction conditions: methanol added at 0 h, 2 h, 4 h, 10% (w/w) molecular sieve added, lipase dosage 3% (w/w), molar ratio of methanol to lipid 5 : 1 and agitation rate 250 rpm. | |
3.3. Response surface results for immobilized lipase catalyzed methanolysis
Several factors affecting the immobilized lipase-catalyzed methanolysis have been investigated in the following study. To optimize the immobilized lipase-catalyzed methanolysis, a 5-level-4-factor central composite design was employed and the levels of molar ratio of methanol to lipid, lipase dosage, temperature and agitation rate were investigated.
The analysis of variance for the quadratic polynomial model is shown in Tables 3 and 4. The F value (F(14,15) = Sm2/Ss2 = 7.41 > Ft(14,15) = 3.56) suggests that the model is highly significant. Besides, the low probability value [(probability > F) < 0.0500] also indicates the high significance of the model. The high coefficient of determination (R2 = 0.8737) indicates that the model is reasonable.
Table 3 Analysis of variance for the fitted quadratic polynomial model
Source |
Sum of squares |
df |
Mean square |
F value |
Probability (P) > F |
Model |
59.50 |
14 |
4.25 |
7.41 |
0.0002 |
Residual |
8.60 |
15 |
0.57 |
— |
— |
Lack of fit |
7.51 |
10 |
0.75 |
3.42 |
0.0934 |
Pure error |
1.10 |
5 |
0.081 |
— |
— |
Cor total |
68.10 |
29 |
— |
— |
— |
R2 = 0.8737 |
Adj. R2 = 0.7557 |
|
|
Table 4 Regression analysis of the second-order polynomial model
Source |
Sum of squares |
df |
Mean square |
F value |
Probability (P) > F |
Significant at 1% level. |
Model |
59.50 |
14 |
59.50 |
7.41 |
0.0002a |
X1 |
0.20 |
1 |
0.20 |
0.35 |
0.5656 |
X2 |
6.98 |
1 |
6.98 |
12.16 |
0.0033a |
X3 |
1.50 |
1 |
1.50 |
2.62 |
0.1267 |
X4 |
15.20 |
1 |
15.20 |
26.50 |
0.0001a |
X1X2 |
0.12 |
1 |
0.12 |
0.20 |
0.6599 |
X1X3 |
0.28 |
1 |
0.28 |
0.49 |
0.4948 |
X1X4 |
1.22 |
1 |
1.22 |
2.13 |
0.1652 |
X2X3 |
0.32 |
1 |
0.32 |
0.56 |
0.4672 |
X2X4 |
0.31 |
1 |
0.31 |
0.55 |
0.4711 |
X3X4 |
1.08 |
1 |
1.08 |
1.89 |
0.1899 |
X12 |
0.48 |
1 |
0.48 |
0.84 |
0.3740 |
X22 |
0.065 |
1 |
0.065 |
0.11 |
0.7407 |
X32 |
28.48 |
1 |
28.48 |
46.16 |
<0.0001a |
X42 |
2.31 |
1 |
2.31 |
4.02 |
0.0633 |
It can be seen that the coefficients of X2, X4 and X32 are significant at a 1% confidence level. The polynomial quadratic model for the 6 h FAME yield regressed by considering only the significant terms is shown as below:
|
Yyield = 96.54 + 0.54X2 + 0.80X4 − 0.98X32
| (2) |
The positive coefficients of X2 and X4 indicated a linear effect to increase Yyield. However, the quadratic term X32 decreases Yyield.
Fig. 7(A)–(F) are 2D contour plots of the interaction of four different factors, which suggest the effects of two factors at a time on the 6 h FAME yield and the interaction between these factors, while the other two factors are held constant.
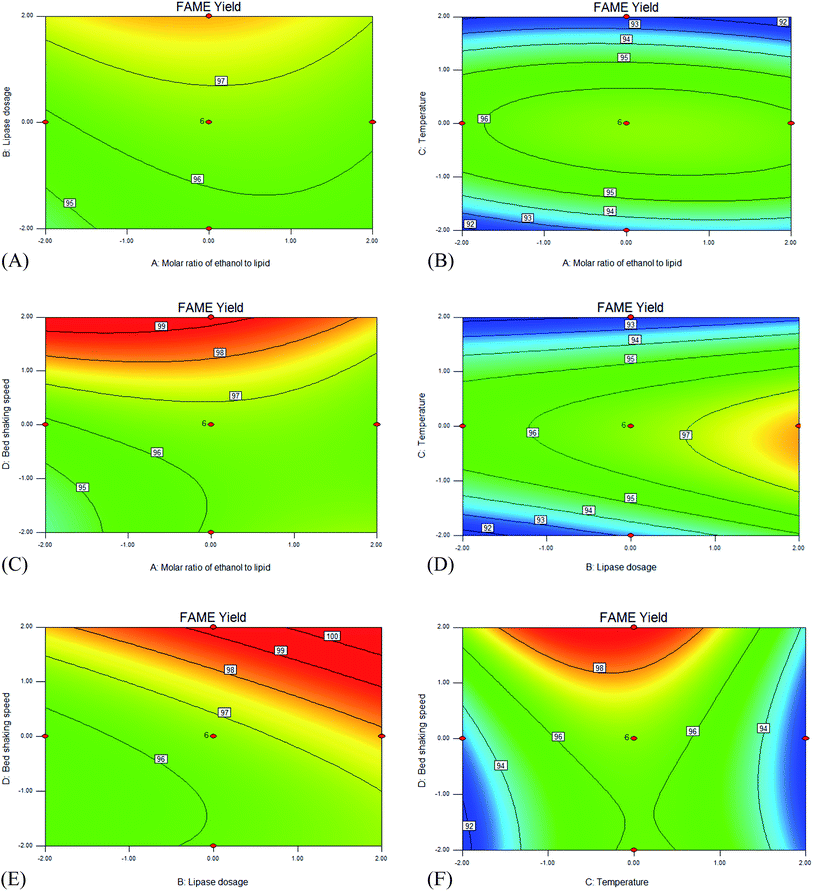 |
| Fig. 7 Contour plots of the combined effect of the molar ratio of methanol to lipid, lipase dosage, temperature and agitation rate at 6 h FAME yield from Chlorella protothecoides: (A) agitation rate 250 rpm, temperature 45 °C; (B) lipase dosage 3% (w/w), agitation rate 250 rpm; (C) lipase dosage 3% (w/w), temperature 45 °C; (D) molar ratio of methanol to lipid 5 : 1, agitation rate 250 rpm; (E) molar ratio of methanol to lipid 5 : 1, temperature 45 °C; (F) molar ratio of methanol to lipid 5 : 1, lipase dosage 3% (w/w). | |
Fig. 7(A) shows that when the temperature and agitation rate are held at a middle level, the effect of the molar ratio of methanol to lipid is not significant especially when the lipase dosage is high. It could be interpreted that when the lipase dosage is high, the lipase activity is the key factor and the effect of the reaction dynamics is not significant. On the other hand, the effect of lipase dosage is significant. It can be seen from Fig. 7B, D and F that when the temperature is about 45 °C, the response Yyield is highest. Fig. 7C indicates that when the molar ratio of methanol to lipid is below 6, the effect of the agitation rate at 6 h FAME yield is significant. However, when the molar ratio of methanol to lipid is above 6, the agitation rate had little influence. It is probably because when the molar ratio of methanol to lipid is high, the toxicity of the methanol to the lipase counteracts the decrease of the mass transfer resistance. Fig. 7E shows that when the molar ratio of methanol to lipid and the temperature are held at a middle level, the effects of the lipase dosage and agitation rate are both very significant. An increment of the FAME yield at 6 h is observed with an increase in lipase dosage or agitation rate.
The optimal transesterification conditions can be calculated with the quadratic polynomial model according to the limit criterion of methanol dosage minimization and FAME yield at 6 h maximization: molar ratio of methanol to lipid 3
:
1, lipase dosage 5% (w/w), temperature 45 °C and agitation rate 300 rpm.
3.4. FFAs in the immobilized lipase-catalyzed methanolysis procedure
It can be seen that the FAME yield had been elevated in the immobilized lipase-catalyzed procedure from the context above and Table 5. Table 5 also suggests that the FFA content had been reduced in the second-step catalysis. Also, it can be observed that almost all of the triacylglycerol had been transformed into FAME after 2 h. In conclusion, the two-step enzymatic catalysis was effective in converting the remaining FFA into FAME. Compared with the use of only novozym-435, it not only can be solvent free, but it is much faster and can reach a much higher FAME yield.
Table 5 Immobilized lipase-catalyzed second-step methanolysis reaction conditions: methanol added at 0 h, 2 h, 4 h, temperature 45 °C, 10% (w/w) molecular sieve added, lipase dosage 5% (w/w), molar ratio of methanol to lipid 3
:
1 and agitation rate 300 rpm
Time (h) |
FAME yield (%) |
Acid value (mg g−1) |
Monoglyceride content (%) |
Diacylglycerol content (%) |
0 |
78.5 ± 0.5 |
24.44 ± 0.32 |
0.60 ± 0.07 |
0.15 ± 0.03 |
1 |
88.2 ± 1.1 |
9.99 ± 0.23 |
Trace |
Trace |
2 |
94.2 ± 0.6 |
4.91 ± 0.57 |
Trace |
Trace |
4 |
95.8 ± 0.2 |
1.47 ± 0.47 |
Trace |
Trace |
6 |
97.0 ± 0.8 |
0.86 ± 0.23 |
Trace |
Trace |
The stability of the immobilized lipase was also investigated and it was found that there was no obvious loss in lipase activity after 5 batches (data not shown). The good operational stability of the lipase was mainly due to the removal of glycerol by-product in the first step catalysis.
4. Conclusions
The combination of free lipase and immobilized lipase in converting microalgae lipid for biodiesel production was developed in this study. It was demonstrated that the addition strategy of methanol, molecular sieve dosage, lipase dosage and temperature had a significant influence on immobilized lipase-mediated methanolysis. Under the optimized conditions, a biodiesel yield of 97% could be achieved with an acid value less than 1 mg KOH per g oil. This process showed great prospect in converting non-edible oils, especially microalgae lipid, for biodiesel production.
Acknowledgements
The authors express their gratitude for the support from Science & Technology Department of Guangdong Province (2015B020215001) and National Natural Science Foundation of China (21376139).
References
- X. Chen, W. Du, D. H. Liu and F. Ding, J. Chem. Technol. Biotechnol., 2008, 83, 71 CrossRef CAS.
- T. M. Mata, A. A. Martins and N. S. Caetano, Renewable Sustainable Energy Rev., 2010, 14, 217 CrossRef CAS.
- I. Rawat, R. R. Kumar, T. Mutanda and F. Bux, Appl. Energy, 2013, 103, 444 CrossRef CAS.
- A. Concas, A. Steriti, M. Pisu and G. Cao, Bioresour. Technol., 2014, 153, 340 CrossRef CAS PubMed.
- A. Steriti, R. Rossi, A. Concas and G. Cao, Bioresour. Technol., 2014, 164, 70 CrossRef CAS PubMed.
- M. Griffiths, R. Van Hille and S. Harrison, Lipids, 2010, 45, 1053 CrossRef CAS PubMed.
- L. M. Laurens, M. Quinn, S. Van Wychen, D. W. Templeton and E. J. Wolfrum, Anal. Bioanal. Chem., 2012, 403, 167 CrossRef CAS PubMed.
- W. Du, W. Li, T. Sun, X. Chen and D. Liu, Appl. Microbiol. Biotechnol., 2008, 79, 331 CrossRef CAS PubMed.
- Y. Li, W. Du and D. Liu, Biochem. Eng. J., 2015, 94, 45 CrossRef CAS.
- H. Ren, Y. Li, W. Du and D. Liu, J. Am. Oil Chem. Soc., 2013, 90, 73 CrossRef CAS.
- Y. Shimada, Y. Watanabe, T. Samukawa, A. Sugihara, H. Noda, H. Fukuda and Y. Tominaga, J. Am. Oil Chem. Soc., 1999, 76, 789 CrossRef CAS.
- Y. Shimada, Y. Watanabe, A. Sugihara and Y. Tominaga, J. Mol. Catal. B: Enzym., 2002, 17, 133 CrossRef CAS.
- S. Cesarini, P. Diaz and P. M. Nielsen, Process Biochem., 2013, 48, 484 CrossRef CAS.
- J. Marchetti, V. Miguel and A. Errazu, Fuel Process. Technol., 2008, 89, 740 CrossRef CAS.
- E. G. Bligh and W. J. Dyer, Can. J. Biochem. Physiol., 1959, 37, 911 CrossRef CAS PubMed; R. Halim, M. K. Danquah and P. A. Webley, Biotechnol. Adv., 2012, 30, 709 CrossRef PubMed.
- D. Royon, M. Daz, G. Ellenrieder and S. Locatelli, Bioresour. Technol., 2007, 98, 648 CrossRef CAS PubMed.
- J. Lu, Y. Chen, F. Wang and T. Tan, J. Mol. Catal. B: Enzym., 2009, 56, 122 CrossRef CAS.
- W. Du, Y. Xu, J. Zeng and D. H. Liu, Biotechnol. Appl. Biochem., 2004, 40, 187 CrossRef CAS PubMed.
|
This journal is © The Royal Society of Chemistry 2016 |