DOI:
10.1039/C6RA07051E
(Paper)
RSC Adv., 2016,
6, 67997-68004
Investigation of free volume characteristics of the interfacial layer in poly(methyl methacrylate)–alumina nanocomposite and its role in thermal behaviour†
Received
17th March 2016
, Accepted 7th July 2016
First published on 7th July 2016
Abstract
The free volume characteristics (free volume size, density and size distribution) of the interfacial layer (IL) in PMMA–alumina (0.3 wt%) nanocomposites were investigated using the well-established positron annihilation lifetime spectroscopy (PALS) technique. Differential scanning calorimetry (DSC) measurements were carried out to investigate the glass transition process of the PMMA matrix in these nanocomposites. PALS results show significant variations in the free volume structure of the nanocomposite, contrary to the assumptions made in earlier reports, i.e., free volume in PMMA remains unaffected on alumina loading. The study shows that as a result of strong interfacial interaction between PMMA and alumina, a denser region is created in the vicinity of alumina particles (interfacial layer), having smaller-sized nanoholes with narrower size distribution compared to bulk PMMA. This observation is consistent with the studies wherein an interfacial layer with constrained segmental motion was inferred from spectroscopic and thermal analysis techniques. The glass transition temperature, Tg, determined from DSC is observed to vary with alumina loading qualitatively similar to the variation in the fractional free volume of PMMA. The study indicates that the critical free volume for PMMA to undergo glass transition process depends on the interfacial layer fraction and hence varies as a function of alumina loading.
1. Introduction
Polymer nanocomposites are considered potential materials due to their enhanced thermo-mechanical properties as compared to the pure polymer matrix. It is assumed that due to the large nanofiller surface area available for interaction with the polymer matrix, the molecular packing of a significantly large volume of polymer matrix in the vicinity of nanofillers is altered.1,2 This region is termed the interfacial layer (IL), and its extension mainly depends on interfacial interaction as well as the dispersion of nanofillers in the polymer matrix.1–5 In the case of very fine dispersions of nanofillers, the interparticle distance is reduced, leading to a crossover (percolation) within the IL boundary associated with different nanofiller particles, finally resulting in complete alteration of molecular packing throughout the polymer matrix.6–9 The role of IL structure in thermo-mechanical properties is an active area of research, as understanding it will help in tuning the bulk physical properties of the nanocomposites. In this regard, polymethyl methacrylate (PMMA)-based nanocomposites with nanosized alumina (Al2O3) fillers have shown dramatic change in their thermo-mechanical properties at low alumina loading (<1.0 wt%).10,11 Ash et al. have shown that the glass transition temperature (Tg) of PMMA–alumina nanocomposites is reduced by 20 °C on addition of 1 wt% alumina, and this reduction in Tg is also accompanied by brittle-to-ductile transition of the nanocomposites.10,11 The mechanical properties have been explained considering the de-wetting of the PMMA–alumina interface leading to shear yielding when subjected to external load.10 In order to explain the reduction in Tg in this nanocomposite, Narayanan et al. have carried out X-ray photon correlation spectroscopy measurements and showed that the polymer matrix faces an internal stress in the presence of the nanoparticles. This internal stress, which arises due to entropic penalty, is relieved due to a relatively faster relaxation aided by de-wetting of the PMMA–alumina interface.12 In this study, it had been assumed that the addition of such low concentrations of alumina does not alter the free volume characteristics of the PMMA matrix, which otherwise would have significantly influenced the segmental relaxation of polymer chains as well as the thermo-mechanical properties of the nanocomposites.
Positron annihilation lifetime spectroscopy (PALS) is an established technique to determine the free volume characteristics of polymers.13 In the last few years, we have shown that the triplet state (ortho-positronium, o-Ps) of the bound form of a positron with an electron is an efficient probe to investigate the changes in free volume characteristics of the polymer matrix in its nanocomposites.6–9 Whenever a positron from o-Ps annihilates with an opposite-spin electron (other than its partner) from the surrounding medium, which is true for an o-Ps trapped in the nanoholes in a polymer matrix, its lifetime is reduced to few ns (pick-off lifetime) as compared to its intrinsic lifetime (142 ns). The reduction in o-Ps lifetime depends on the size of free volume holes, which means smaller the nanohole size, the shorter will be the pick-off lifetime. The formation probability of o-Ps in a matrix depends on the density of nanoholes. The o-Ps pick-off lifetime (τpo) in a medium can be correlated to the nanohole radius (R) using the well-established Tao–Eldrup equation:14,15
|
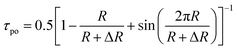 | (1) |
In this equation, ΔR = 0.166 nm is an empirical parameter that has been determined using materials with well-known free volume size.16 The o-Ps intensity is correlated with the nanohole's density or fractional free volume (FFV) in chemically invariable polymer matrices. PALS measurements also provide the free volume nanohole size distribution in a polymer matrix considering that the size of nanoholes follow a distribution, e.g. Gaussian, around its mean value. Due to the high sensitivity of o-Ps annihilation parameters (lifetime, intensity and distribution) to free volume characteristics, any change in free volume nanohole size, density and size distribution as a result of inclusion of nanofillers in a polymer matrix can be determined using PALS.
In the present study, we have investigated the free volume characteristics of PMMA–alumina nanocomposites with loading up to 3.0 wt% using PALS. Significant variation in free volume nanohole size, density and size distribution was observed even at very low loading (0.2 wt%). These observations contradict the assumptions made in literature for fixing the roles of different structural parameters towards the observed decrease in Tg for PMMA–alumina nanocomposites. The thermal behaviour of the present nanocomposites was investigated using Differential Scanning Calorimetry (DSC), and the observed variation in glass transition process is correlated with the free volume characteristics of the nanocomposites.
2. Experimental section
2.1. Preparation of PMMA–alumina nanocomposites
Poly methyl methacrylate, PMMA, (∼M.W. 350 kDa) and γ-alumina nanoparticles were procured from Otto Chemicals, Mumbai, India (average particle size = 20–30 nm). The PMMA–alumina nanocomposites were prepared through solvent casting. PMMA (2 g) was dissolved in 100 ml acetone at ∼40 °C under continuous stirring for 2 hours. The alumina powder was heated to ∼150 °C under vacuum (∼10−3 mbar) for 2 hours to remove any physisorbed moisture from the alumina particle surface. According to the wt% of alumina in the nanocomposites, the calculated amount of alumina was dispersed in 30 ml acetone. The alumina dispersion was subjected to ultrasonication (Elma S450H, ultrasonication power = 400 W) for 2 hours. The alumina dispersion was added to PMMA solution under stirring at 40 °C. After 3 hours of stirring, the (PMMA–alumina) solution was cast on a glass Petri dish. To control the evaporation of acetone, the Petri dish was covered with aluminium foil with a few small holes. After overnight drying, the nanocomposite films were taken off from the Petri dish. Following the above mentioned procedure, pure PMMA as well as PMMA–alumina nanocomposite films with 0.2, 0.5, 1.0, 2.0, 3.0 wt% of alumina were prepared. The as-prepared films were dried at 70 °C for 3 hours under vacuum (∼10−3 mbar) to remove any retained casting solvent from the films. These samples are further noted as PMxAl, where x represents the loading (wt%) of alumina in PMMA films. The film thickness was measured using a digital micrometer and was found to be 60 ± 15 μm.
2.2. Characterization of PMMA–alumina nanocomposites
X-ray diffraction patterns of alumina nanoparticles, pure PMMA and PMMA–alumina nanocomposites were recorded with a STOE diffractometer using monochromatized Cu Kα (λ = 0.154 nm) radiation. Differential scanning calorimetry (DSC; model number DSC 823e/700 of M/s. Mettler Toledo GmbH, Switzerland) measurements were carried out in N2 atmosphere at 10 K min−1 heating rate. The glass transition temperature of the nanocomposites was determined from the second heating run at 10 °C min−1. The Fourier transform-infrared (FTIR) spectrum of alumina powder was recorded by mixing it in KBr powder. The spectrum was recorded in diffused reflectance mode using a Vertex 80v (Bruker) spectrometer couple with a liquid nitrogen-cooled MCT detector from 4000 to 400 cm−1 at a resolution of 2 cm−1. The SEM micrographs of the film's surface were captured using a Sigma FE-SEM instrument from Carl Zeiss Microscopy Ltd., Cambridge, UK.
2.3. Free volume characteristics of PMMA–alumina nanocomposites
Free volume characteristics (free volume nanohole size, density and size distribution) of PMMA–alumina nanocomposites were determined through PALS measurements. The PALS spectrometer used for the measurements was a fast–fast coincidence set-up (time resolution = 260 ps) coupled with two plastic scintillation detectors. PALS spectra of alumina nanoparticles, pure PMMA and PMMA–alumina nanocomposites were recorded at room temperature in lab atmosphere. For these measurements, a positron source (22Na, ∼0.37 MBq) deposited between two kapton films (thickness ∼7 micrometer) was sandwiched between two stacks of polymer films (thickness of stack >1.5 mm). The whole assembly (source and sample) was kept at centre between two plastic scintillation detectors facing each other. The time dispersion for acquiring the spectra was 0.0127 ns per channel. To correct for the fraction of positrons annihilating in the source or kapton films (source correction components), PALS spectrum of a reference (Si single crystal) was also acquired. Two source correction components (0.412 and 2.16 ns with corresponding intensities 12.8% and 2.1%) were subtracted for the analysis of PALS spectra of the nanocomposites. For PALS measurement in nanosized alumina, the positron source was embedded in a sufficient amount of alumina powder, ensuring all positrons annihilate within the powder.
3. Results and discussion
3.1. XRD of nanosized alumina and PMMA–alumina nanocomposites
Fig. 1 shows the XRD pattern of alumina nanoparticles, which have been used as filler for the preparation of nanocomposites. The diffraction peaks correspond to γ-alumina and are rather broad, indicating that the crystallite size is in nanometer range. The crystallite size ca. 4.32 nm of γ-alumina has been calculated using Debye–Scherrer equation (ESI†). In order to investigate the PMMA matrix morphology, XRD diffraction patterns of pure PMMA and its nanocomposite with alumina are recorded and shown in Fig. 2. Broad humps at 2θ ∼ 15, 30 and 41 degrees are observed for pure PMMA samples, indicating its amorphous nature.17,18 On addition of alumina nanoparticles, no sharp peaks corresponding to crystalline phases are observed, indicating the amorphous nature of the nanocomposites. The Bragg diffraction peaks corresponding to alumina are also not observed from the nanocomposite's XRD traces, probably due to low loading (max. 3 wt%) as well as fine dispersion of alumina in the PMMA matrix. The XRD patterns of the nanocomposites are qualitatively different, e.g., PM(0.2–1.0)Al samples show a rather narrower hump peaking at smaller 2θ value, while samples with higher loading show a broader hump. However, it is difficult to extract quantitative information about the packing of PMMA chains from the XRD patterns.
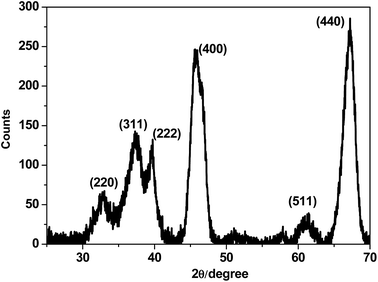 |
| Fig. 1 XRD pattern of alumina nanoparticles. The Bragg diffraction peaks indicate that alumina is in γ form. | |
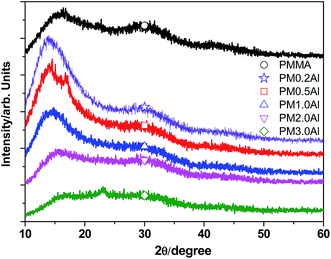 |
| Fig. 2 XRD patterns of pure PMMA and PMMA–alumina nanocomposites. | |
3.2. Free volume structure at interfacial layer (IL) from PALS
As mentioned before, PALS is an established technique to determine the free volume structure of a polymer matrix that has recently been employed successfully for the investigation of free volume structure of IL in polymer nanocomposites.7–9 These studies have shown that o-Ps is efficiently trapped in the free volume nanoholes at IL, and its lifetime, intensity and lifetime distribution can be well correlated with the size, density and size distribution of nanoholes in polymer nanocomposites. PALS spectra of alumina, pure PMMA and PMMA nanocomposites were analyzed using the computer program PALSfit into discrete positron lifetime components, keeping all input parameters free for analysis.19 Three positron lifetime components are observed in alumina (ESI, Table S1†), indicating a small fraction of positronium formation (2.43%) with its lifetime at 8.44 ± 2.43 ns in the nanosized alumina. PALS spectra of PMMA and its nanocomposites with alumina could be resolved into three as well as four positron lifetime components with acceptable reduced variance of fit (0.98–1.10). We have considered the four-component fit, as the reduced variance of fit was better for this analysis, which is also supported by continuous distribution analysis of PALS spectra, as discussed later. For comparison with earlier PALS results on PMMA films wherein three lifetime components have been reported, the three-component results are also shown in Fig. S1 (ESI†). The present o-Ps lifetime value (Fig. S1,† τ3 = 2.27 ± 0.02 ns) for pure PMMA is larger than the reported o-Ps lifetime (∼1.90 ns) for bulk PMMA materials.20 A study on solution-cast PMMA films has also shown that casting solution affects the free volume size in PMMA.21 Recently, a similar value of o-Ps lifetime (2.24 ± 0.04 ns) for PMMA films by Pejcic et al. has been reported for the solvent-cast PMMA films.22
Fig. 3 and 4 show the variation in o-Ps lifetime and its corresponding intensity as a function of alumina loading in PMMA matrix. It is observed from both figures that the o-Ps lifetimes (τ3 and τ4) first decrease on 0.2 wt% loading of alumina, followed by a continuous increase on further loading. The variation observed in o-Ps lifetime and intensity cannot be explained considering the admixture rule for positron annihilation from PMMA and alumina (Fig. S2, ESI†), and, hence, this variation is related to the modification in free volume structure of the PMMA matrix. This type of variation in o-Ps lifetime has been observed in various nanocomposites and has been explained considering poor dispersion (aggregation) of nanofillers at higher concentrations.7–9,23 At the lowest studied concentration, alumina nanoparticles are finely dispersed in PMMA matrix and provide a large surface area for interaction with PMMA molecules. This interaction probably occurs between (–OH) functionalities from the alumina surface (Fig. S3, ESI†) and the methoxy group of the PMMA chain. Such type of PMMA–nanofiller interaction has also been shown in different studies through FTIR measurements of the PMMA-based nanocomposites.24,25 This interfacial interaction between PMMA chains and alumina nanoparticles appears to restrict the chain motion, leading to a reduction in size of nanoholes in the vicinity of the nanoparticles, i.e., at IL. Such type of reduced chain motion due to interfacial interaction between PMMA and cellulose nanocrystals has been inferred by Dong et al. through the thermal properties of the nanocomposites.26 The effect of constrained chain motions on the free volume nanoholes at IL and bulk polymer layer in the nanocomposite is shown in Scheme 1. In such a case, the determined values of o-Ps lifetime (τ3 or τ4) can be ascribed through eqn (1) to the weighted average (Rav) of nanohole radii from the IL (Rint) and polymer bulk (RB), where Rav = (Rintnint + RBnB)/(Rint + RB). The parameters nint and nbulk are the values of nanohole density at the IL and bulk region, respectively (nint < nbulk).
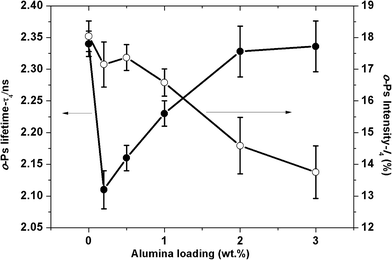 |
| Fig. 3 o-Ps lifetime (τ4/ns) and corresponding intensity ascribed to larger free volume nanoholes in PMMA matrix in the nanocomposites. | |
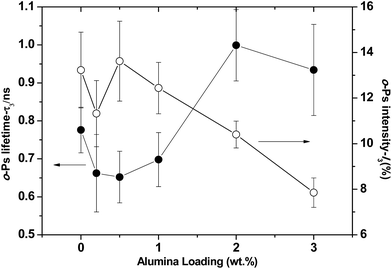 |
| Fig. 4 o-Ps lifetime (τ3/ns) and corresponding intensity ascribed to larger free volume nanoholes in PMMA matrix in the nanocomposites. | |
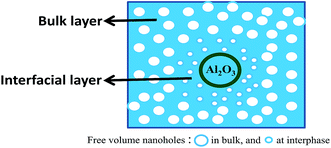 |
| Scheme 1 Free volume nanoholes present at bulk and IL in PMMA–alumina nanocomposites. | |
The creation of IL with smaller or larger nanohole sizes has been confirmed using PALS in different polymer nanocomposites.7–9 These results from PALS are supported by the observation of a rigid amorphous phase along with a mobile amorphous phase in polymer nanocomposites through thermal analysis.27 Depending on the interfacial interaction, in some cases two distinct o-Ps lifetime components corresponding to IL and bulk are also reported.6,8 In the cases wherein the nanohole sizes at IL and bulk layer of the polymer are not distinctly different, variation in nanohole size distribution (discussed later) is observed instead of two discrete o-Ps lifetime components.6,8 Polymer confinement between nanofiller sites even in the absence of any interfacial interaction is also shown to affect the thermal properties of the polymer matrix, which is ascribed to the change in molecular packing (free volume characteristics) of polymer chains due to their geometric confinement between nanofillers.28,29 Qin et al. have shown that the effect of confinement for variation in thermal properties (Tg) is opposite for weak (sometime repulsive) and strong (attractive) interfacial interaction.28 In this study, it has also been shown that the effect of confinement is maximum below interparticle distance (∼20 nm), and it diminishes (increases) with the increase (decrease) in interparticle distance. In the present case, the interparticle distance between two nanofiller sites assuming the finest dispersion of alumina for 0.2 wt% is ca. 114 nm (see ESI† for calculation of interparticle distance), which is large compared to values reported for the observation of confinement effect on PMMA chains. Hence, the observed variations in o-Ps lifetime and intensity can be exclusively correlated to the variation in molecular packing of PMMA chains at IL as a result of interfacial interaction. The effect of interfacial interaction is only extended to IL having its width smaller than the interparticle distance in the nanocomposite. Hence, at the lowest loading (0.2 wt%), an IL with smaller nanohole size along with reduced density is created due to interfacial interaction between PMMA and alumina nanoparticles (Scheme 1). The width of such an IL (distance from particle surface to the IL-bulk boundary) in this nanocomposite should be less than half of the interparticle distances, i.e. ∼57 nm.
On higher loading, dispersion of particles is hampered due to interparticle interaction resulting in larger size of alumina aggregates in the PMMA matrix. As mentioned before, the aggregation of nanofillers at higher concentration has been observed in various nanocomposites.7–9,23,27,28 The aggregation of alumina particles in larger aggregates is confirmed in the present case from the scanning electron micrographs (Fig. 5). As a result of aggregation, the surface area available for the interfacial interaction from the nanofiller to polymer chains is reduced. In this case, the IL volume fraction, considering it proportional to the surface area of nanofiller, is also reduced, and consequently o-Ps lifetime (weighted average of o-Ps lifetime from bulk and IL) starts increasing with the loading of alumina (Fig. 3 and 4). These aggregates, due to their large size, also contain polymer chains adhered to the interparticle surface, which are confined within the aggregate. The confinement of polymer chains between nanofiller particles has been confirmed in different nanocomposites through X-ray diffraction (intercalation in nanoclays) as well as in polymer–silica nanoparticle composites through thermal analysis.27,28 The interpenetrating polymer chains strongly interact with the particle's surface through a large number of contact points. This type of strong interaction leads to a drastic reduction in their segmental motion, finally resulting in loss of nanohole density associated with these chains. The loss of nanohole density associated with these confined chains is reflected in a continuous decrease of o-Ps intensity in these nanocomposites with an increase in loading of alumina (Fig. 3 and 4). Scheme 2 represents the free volume structure of PMMA–alumina nanocomposites in the (a) absence of aggregation and (b) presence of aggregation of alumina.
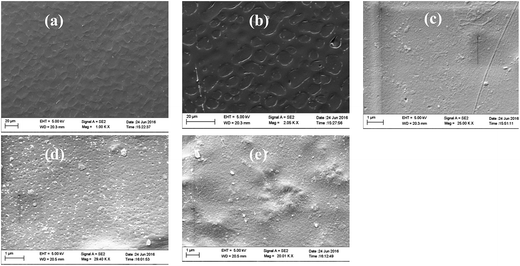 |
| Fig. 5 (a–e) FE-SEM micrographs of PMxAl composites with x = 0, 0.2, 0.5, 1.0 and 2.0 wt%, respectively. | |
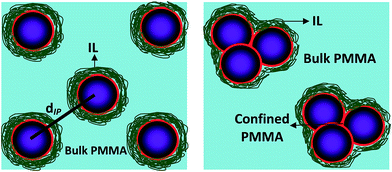 |
| Scheme 2 Illustration of structure (left panel) without aggregation (PM0.2Al) and (right panel) with aggregation (PMxAl, x > 0.2). IL indicates the interfacial layer. | |
As both parameters, o-Ps lifetime and intensity, vary as a function of alumina loading, in such a case, the fractional free volume (FFV) provides average information compared to individual parameters. Relative FFV calculated according to the following equation is plotted in Fig. 6:
|
FFV = 4π[I3R33 + I4R43]/3
| (2) |
where
R3 and
R4 are the nanohole's radii calculated from
o-Ps lifetime values (
τ3,
τ4) using
eqn (1), and
I3 and
I4 are the corresponding
o-Ps intensities in the fraction.
Fig. 6 shows that the FFV decreases with alumina loading at 0.2 wt% followed by an increase up to 2 wt%. At highest loading, again a decrease in FFV is observed, which can be attributed to the large fraction of polymer chains confined between the particles in the aggregates.
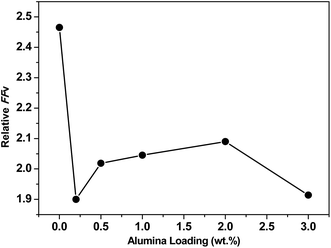 |
| Fig. 6 The relative total fractional free volume in PMMA–alumina nanocomposites as a function of alumina loading. | |
It is a well known fact that the polymer matrix has a free volume nanohole size distribution, and the discrete o-Ps annihilation lifetime corresponds to the statistical average of the radii of these nanoholes. In the case of polymer nanocomposites, along with the size and density of the nanoholes, their size distribution is also modified.6–9 Hence, information regarding the modification of nanohole distribution is crucial to understanding the role of the nanofiller in the molecular packing of the polymer chains. The continuous distribution of o-Ps lifetime is extracted from PALS spectrum using the program CONTIN, which is based on inverse Laplace transform. The details of this program can be obtained elsewhere.30 The extracted o-Ps lifetime distribution can also be transformed to nanohole radius or nanohole volume distribution using eqn (1) as described in detail in our earlier study on the polyvinyl alcohol–SiC nanocomposite.8 In recent years, we have applied this program successfully for different polymer nanocomposites, and the distribution of nanohole size has been correlated to the thermo-mechanical properties of these nanocomposites.6–9 Fig. 6 shows the o-Ps lifetime distribution in PMMA–alumina nanocomposites, wherein two o-Ps lifetime distributions are observed. The bimodal distribution (Fig. 7) of o-Ps lifetimes in PMMA and its nanocomposites with nanosized alumina support the discrete component analysis, wherein two o-Ps lifetime components were observed in pure PMMA as well as in the nanocomposites (Fig. 3 and 4). The average values of the distributions (peak positions) fairly match the discrete component results. In order to compare the size distributions, the o-Ps lifetime distribution traces have been fitted to the Gaussian function, and the full width at half maxima (FWHM) corresponding to longer o-Ps lifetime, τ4, (larger nanohole size) is plotted in Fig. 8. It is observed that the distribution of nanoholes in PMMA matrix is also severely modified with the loading of alumina, and it follows a trend similar to that of the o-Ps lifetime (Fig. 3). This indicates that the nanoholes present at IL have narrower size distribution compared to the bulk polymer. As the loading of nanofillers is increased, due to reduction in IL fraction the distribution becomes broader, and FHWM for 2 wt% loading becomes similar to that of pure PMMA (Fig. 7). The nanocomposite with 3 wt% loading shows a decreased FFV and FHWM as compared to 2 wt% loading, which we attribute to the confinement of polymer chains between the alumina particles in the aggregates.
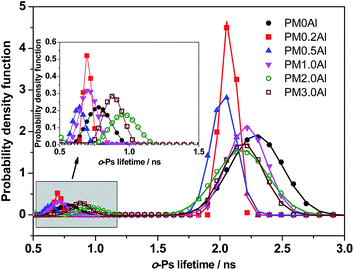 |
| Fig. 7 Free volume nanohole size distribution in PMMA–alumina nanocomposites. | |
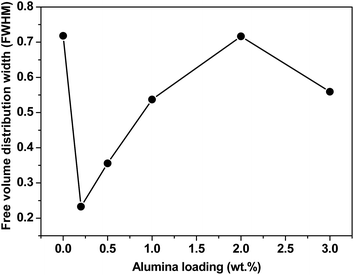 |
| Fig. 8 Free volume nanohole size distribution corresponding to larger nanohole size in PMMA–alumina polymer nanocomposites. | |
3.3. Role of free volume structure in thermal behaviour
The glass transition process of the polymer matrix in polymer nanocomposites is indexed to observe the effect of nanofiller loading on the thermal properties.26–28 The change in thermal properties as a function of loading is also used to infer the changes in the molecular packing of polymer chains.27 In this regard, any change in glass transition temperature, Tg, (start of the glass transition process) is determined from DSC or dynamic mechanical analysis (DMA) techniques, and the observed change as result of nanofiller loading is correlated to the rate of segmental motions of polymer chains. Both positive and negative deviation as compared to pure polymer matrix have been observed in polymer nanocomposites,10,26 which have been explained in terms of the changes in molecular packing as a result of interfacial interaction between polymer and nanofillers.10,26,28 As mentioned earlier, the observed decrease in Tg for PMMA nanocomposites with alumina nanoparticles has been explained to be due to faster segmental motion of PMMA chains as a result of internal stress.11,12 In this study, it is assumed that low loading of alumina (≤5 wt%) in PMMA matrix would not alter the free volume characteristics of the matrix. Also, the confinement of polymer chains between the nanofillers has not been considered. However, it has been shown from spectroscopic techniques that PMMA chains interact with (–OH) functionalities present at the alumina particle surface, and a region (IL) having constrained segmental motion is created.31,32 The present study shows that the free volume characteristics of the PMMA matrix are severely modified as a result of alumina loading (Fig. 3, 4 and 6–8). The observed variations in free volume characteristics (o-Ps annihilation parameters) also indicate the confinement of polymer chains with increased alumina loading. It is well known that the thermo-mechanical properties, e.g. Tg, are dependent on the free volume characteristics of polymers. Hence, the free volume characteristics of PMMA in its nanocomposites have to be considered in explaining the observed changes in its thermal behaviour.
Fig. 9 shows the DSC thermograms from the 2nd heating cycle of the nanocomposites. The glass transition temperature (Tg) values of pure PMMA and the nanocomposites have been determined as the onset of the glass transition process, which is the intersection point of the tangents from the low temperature base line and from the inflection point as shown in Fig. S4 (ESI†). Thus determined Tg values are shown in Table 1. The temperature values corresponding to the end of the glass transition process, which has been determined as the intersection of tangents from the inflection point and the high temperature baseline, are also shown in the table. The present study does not show a drastic decrease in Tg in PMMA–alumina nanocomposites as observed by Ash et al.11 However, the present results are consistent with the other studies on PMMA-based nanocomposites having attractive interfacial interactions.26–28,37 It is observed that the variation in Tg (Table 1 and Fig. 10) follows a trend qualitatively similar to relative FFV (Fig. 6) as well as the nanohole size distribution or o-Ps lifetime (Fig. 8), but the observed variations cannot be quantitatively correlated. In the case of bulk polymers having Tg > RT (room temperature), it has been reported that Tg and FFV (determined at RT) are inversely related, considering that a critical FFV or nanohole size 〈VC〉 is required for the onset of glass transition process for a particular polymer matrix.33 On loading of nanofillers, it has been shown from temperature-dependent PALS measurements that the 〈VC〉 (o-Ps lifetime) at Tg varies as a function of nanofiller loading.34,35 On the other hand, the presence of nanofillers modifies the molecular packing of the polymer matrix at RT, resulting in a higher or lower FFV depending on many factors such as interfacial interaction, dispersion of nanofillers and width of interphase, etc.8 Hence, a direct relation between FFV at RT and value of Tg for a particular nanocomposite cannot be drawn. This is primarily because the presence of nanofillers acts as a physical barrier for the segmental motion of the polymer chains at the interface, and an increase in Tg would be expected. These relaxations may be highly constrained in the presence of strong interfacial interaction between polymer and nanofillers, and two different Tg values can also be observed corresponding to IL and bulk polymer matrix, respectively.36 In the present case, it appears that the critical 〈VC〉 for PMMA to undergo glass transition process is modified in these nanocomposites in such a way that the variation in Tg follows a trend similar to FFV or FHWM of size distribution (Fig. 6 and 8). As discussed above, the variation in FFV and FWHM of size distribution are the results of variation in the volume fraction of IL due to aggregation of alumina at higher loading. This indicates that variation in 〈VC〉 (smaller or larger as compared to pure PMMA) is also a function of IL's volume fraction.
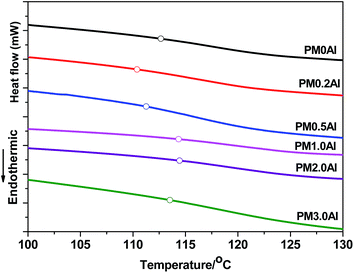 |
| Fig. 9 DSC thermograms of PMMA–alumina nanocomposites. In order to plot the traces without overlap, the traces have been shifted on the Y-axis. | |
Table 1 Onset (Tg) and the end point (Tend) of glass transition process determined from DSC
Sample |
Tg/°C |
Tend/°C |
PM0Al |
112.7 |
122.1 |
PM0.2Al |
110.3 |
120.5 |
PM0.5Al |
111.2 |
120.7 |
PM1.0Al |
114.3 |
124.8 |
PM2.0Al |
114.4 |
124.1 |
PM3.0Al |
113.4 |
123.0 |
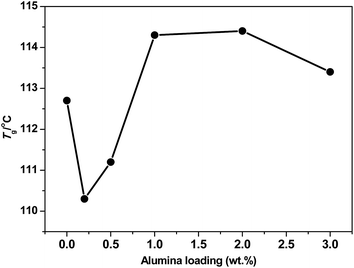 |
| Fig. 10 Variation in Tg as a function of alumina loading in PMMA. | |
4. Conclusion
Positron annihilation lifetime spectroscopy can be efficiently used to investigate molecular packing (free volume characteristics) of interfacial layer of a polymer matrix in its nanocomposites. The present study shows that the free volume characteristics, such as free volume nanohole size, density and size distribution in the PMMA matrix, are severely modified in its nanocomposites with alumina nanofillers. This phenomenon is also expected to be universally true for all other nanofillers. This suggests that the changes in free volume characteristics should be considered when setting up the structure–property correlations in the polymer nanocomposites. The study also indicates that the free volume characteristics of a polymer matrix in polymer nanocomposites determined at ambient temperature cannot be directly related to the glass transition temperature determined from differential scanning calorimetry. Variation in critical free volume for PMMA to undergo the glass transition process appears to be proportional to the interfacial layer's volume fraction in this nanocomposite, which would be confirmed through temperature-dependent positron annihilation lifetime measurements.
Acknowledgements
The authors thank Dr Jyoti Prakash and Mr Ravikumar, BARC, Mumbai for providing the FE-SEM images of the samples.
References
- D. Ciprari, K. Jacob and R. Tannenbaum, Macromolecules, 2006, 39, 6565–6573 CrossRef CAS.
- R. Tannenbaum, M. Zubris, K. David, D. Ciprari, K. Jacob, I. Jasiuk and N. Dan, J. Phys. Chem. B, 2006, 110, 2227–2232 CrossRef CAS PubMed.
- V. Kovacevic, S. Lucic and M. Leskovac, J. Adhes. Sci. Technol., 2002, 16, 1343–1365 CrossRef CAS.
- S. Sen, Y. Xie, S. K. Kumar, H. Yang, A. Bansal, D. L. Ho, L. Hall, J. B. Hooper and K. S. Schweizer, Phys. Rev. Lett., 2007, 98, 128302 CrossRef PubMed.
- S. Coiai, D. Prevosto, M. Bertoldo, L. Conzatti, V. Causin, C. Pinzino and E. Passaglia, Macromolecules, 2013, 46, 1563–1572 CrossRef CAS.
- S. K. Sharma, J. Bahadur, P. N. Patil, P. Maheshwari, S. Mukherjee, K. Sudarshan, S. Mazumder and P. K. Pujari, ChemPhysChem, 2013, 14, 1055–1062 CrossRef CAS PubMed.
- S. K. Sharma, J. Prakash, J. Bahadur, K. Sudarshan, P. Maheshwari, S. Mazumder and P. K. Pujari, Phys. Chem. Chem. Phys., 2014, 16, 1399–1408 RSC.
- S. K. Sharma, J. Prakash, K. Sudarshan, D. Sen, S. Mazumder and P. K. Pujari, Macromolecules, 2015, 48, 5706–5713 CrossRef CAS.
- S. K. Sharma, J. Prakash and P. K. Pujari, Phys. Chem. Chem. Phys., 2015, 17, 29201–29209 RSC.
- B. J. Ash, R. W. Siegel and L. S. Schadler, Macromolecules, 2004, 37, 1358–1369 CrossRef CAS.
- B. J. Ash, R. W. Siegel and L. S. Schadler, J. Polym. Sci., Part B: Polym. Phys., 2004, 42, 4371–4383 CrossRef CAS.
- R. A. Narayanan, P. Thiyagarajan, S. Lewis, A. Bansal, L. S. Schadler and L. B. Lurio, Phys. Rev. Lett., 2006, 97, 075505 CrossRef PubMed.
- P. E. Mallon, Application to Polymers in Principles and Applications of Positron and Positronium Chemistry, ed. Y. C. Jean, P. E. Mallon and D. M. Schrader, World Scientific, London, 2003 Search PubMed.
- S. J. Tao, J. Chem. Phys., 1972, 56, 5499–5510 CrossRef CAS.
- M. Eldrup, D. Lightbody and J. N. Sherwood, Chem. Phys., 1981, 63, 51–58 CrossRef CAS.
- N. Nakanishi, S. J. Wang and Y. C. Jean, in Positron Annihilation Studies of Fluids, ed. S. C. Sharma, World Scientific, Singapore, 1988, p. 292 Search PubMed.
- M. A. Reyes-Acostaa, A. M. Torres-Huertab, M. A. Domínguez-Crespo, A. I. Flores-Vela, H. J. Dorantes-Rosales and E. Ramírez-Menesese, J. Alloys Compd., 2015, 643, 140–148 CrossRef.
- W. Pan, H. Zhang and Y. Chen, J. Mater. Sci. Technol., 2009, 25, 247–250 CAS.
- J. V. Olsen, P. Kirkegaard, N. J. Pedersen and M. Eldrup, Phys. Status Solidi, 2007, 4, 4004–4006 CrossRef CAS.
- J. Zhang, M. Yang and F. H. J. Maurer, Macromolecules, 2011, 44, 5711–5721 CrossRef CAS.
- Y. J. Fu, C. C. Hu, K. R. Lee, H. A. Tsai, R. C. Ruaan and J. Y. Lai, Eur. Polym. J., 2007, 43, 959–967 CrossRef CAS.
- B. Pejcic, E. Crooke, L. Boyd, C. M. Doherty, A. J. Hill, M. Myers and W. Cameron, Anal. Chem., 2012, 84, 8564–8570 CrossRef CAS PubMed.
- G. Xue, J. Zhong, S. Gao and B. Wang, Carbon, 2016, 96, 871–878 CrossRef CAS.
- Z. Wang, S. Leng, Z. Wang, G. Li and H. Yu, Macromolecules, 2011, 44, 566–574 CrossRef CAS.
- G. Goncalves, P. A. A. P. Marques, A. Barros-Timmons, I. Bdkin, M. K. Singh, N. Emamic and J. Gracioa, J. Mater. Chem., 2010, 20, 9927–9934 RSC.
- H. Dong, Y. R. Sliozberg, J. F. Snyder, J. Steele, T. L. Chantawansri, J. A. Orlicki, S. D. Walck, R. S. Reiner and A. W. Rudie, ACS Appl. Mater. Interfaces, 2015, 7, 25464–25472 CAS.
- Q. Ma, B. Mao and P. Cebe, Polymer, 2011, 52, 3190–3200 CrossRef CAS.
- X. Qin, W. Xia, R. Sinko and S. Keten, Nano Lett., 2015, 15, 6738–6744 CrossRef CAS PubMed.
- C. W. Chiu, T. K. Huang, Y. C. Wang, B. G. Alamani and J. J. Lin, Prog. Polym. Sci., 2014, 39, 443–485 CrossRef CAS.
- S. W. Provencher, Comput. Phys. Commun., 1982, 27, 213–227 CrossRef.
- K. Konstadinidis, B. Thakkar, A. Chakraborty, L. W. Potts, R. Tannenbaum, M. Tirrell and J. F. Evans, Langmuir, 1992, 8, 1307 CrossRef CAS.
- Y. Grohens, M. Auger, R. Prudhomme and J. Schultz, J. Polym. Sci., Part B: Polym. Phys., 1999, 37, 2985–2995 CrossRef CAS.
- R. Srithawatpong, Z. L. Peng, B. G. Olson, A. M. Jamieson, R. Simha, J. D. McGervey, T. R. Mair, A. F. Halasa and H. Ishida, J. Polym. Sci., Part B: Polym. Phys., 1999, 37, 2754–2770 CrossRef CAS.
- S. E. Harton, S. K. Kumar, H. Yang, T. Koga, K. Hicks, H. K. Lee, J. Mijovic, M. Liu, R. S. Vallery and D. W. Gidley, Macromolecules, 2010, 43, 3415–3421 CrossRef CAS.
- S. Harms, K. Rätzke, F. Faupel, G. J. Schneider, L. Willner and D. Richter, Macromolecules, 2010, 43, 10505–10511 CrossRef CAS.
- L. Chen, K. Zheng, X. Tian, K. Hu, R. Wang, C. Liu, Y. Li and P. Cui, Macromolecules, 2010, 43, 1076–1082 CrossRef CAS.
- J. Moll and S. K. Kumar, Macromolecules, 2012, 45, 1131–1135 CrossRef CAS.
Footnote |
† Electronic supplementary information (ESI) available. See DOI: 10.1039/c6ra07051e |
|
This journal is © The Royal Society of Chemistry 2016 |