DOI:
10.1039/C6RA06898G
(Paper)
RSC Adv., 2016,
6, 41542-41550
Roll coated large area ITO- and vacuum-free all organic solar cells from diketopyrrolopyrrole based non-fullerene acceptors with molecular geometry effects†
Received
15th March 2016
, Accepted 20th April 2016
First published on 21st April 2016
Abstract
In this paper, we investigate three diketopyrrolopyrrole (DPP) based small molecular non-fullerene acceptors, namely Ph(DPP)3, Ph(DPP)2, and PhDMe(DPP)2, focusing on molecular geometry effects on the frontier orbital level, light absorption, molecular configuration, electron mobility, thin film morphology, and photovoltaic performance of both spin-coated ITO based and roll coated large area, ITO- and vacuum-free organic solar cells (OSCs). For spin-coated devices based on P3HT as the donor polymer the solar cells gave power conversion efficiencies (PCEs) in the following order for (P3HT:PhDMe(DPP)2, 0.65%) > (P3HT:Ph(DPP)2, 0.48%) > (P3HT:Ph(DPP)3, 0.31%). All devices present an open circuit voltage (Voc) higher than 1.0 V. For the roll-coated devices, the PCEs were found to fall in another order and with lower values (P3HT:Ph(DPP)3, 0.54%) > (P3HT:Ph(DPP)2, 0.43%) > (P3HT:PhDMe(DPP)2, 0.04%) and the highest Voc was 0.82 V. Our preliminary results highlight the influence of geometry, structure and processing on the performance of non-fullerene acceptors.
Introduction
Electron accepting materials play a crucial role in the optimization of organic solar cells (OSCs). The majority of the focus has been directed towards optimization of fullerene based materials such as PC61BM, PC71BM, and indene-C60 bisadduct (ICBA), due to their high electron affinity, high electron mobility, isotropic charge transport, and possibly controlled morphology on a nanometer scale.1–7 Nevertheless, drawbacks of fullerene based acceptors such as their relatively low air stability and weak absorption in the energy rich region of the incident solar irradiation limit their ability for photocurrent generation. This combined with the limited tuning possibilities of the energy levels of fullerenes (especially the lowest unoccupied molecular orbital (LUMO) to match that of a given donor) makes the possibility for optimizing the open circuit voltage of the solar cell device limited. This limitation stems from the inability to efficiently split excitons located on the fullerene as described by Bloking et al.8 which give way to a large current drop in polymer/fullerene devices when the Voc exceeds 1.0 V. Accordingly, researchers have largely focused on non-fullerene acceptors, especially small molecule (SM) based, with attention to strong absorption in the visible region, appropriately positioned and tunable highest occupied molecular orbital (HOMO)/LUMO levels, structural uniformity and no batch-to-batch variations compared to their polymer counterparts, with significant progress being made during the past two years.9–17 Among these, most of the work involving vinazene, floranthene-fused imide, naphthalene diimide have been successfully pronounced for fabricating OPVs with PCEs higher than 1.5% and a few even higher than 2.5%.10,18–24 Some perylene diimide derivatives led to OPVs with PCEs higher than 5.0%.15,25–27 However, given their tendency towards crystallization and microscale aggregation with the polymer donor matrices, which strongly impede the formation of proper bulk heterojunction (BHJ) morphology for efficient charge separation and transport, alternatives to PDI based acceptors are also desirable. Diketopyrrolopyrrole (DPP) and its derivatives have become attractive unit for SM acceptors, due to their strong absorptions in the visible region, easily tunable HOMO/LUMO level by introducing electron donating or accepting moieties, and some of them have exhibited high electron mobility (>1 cm2 V−1 s−1).28,29 Meanwhile, the molecular design with defined geometry of SM non-fullerene acceptors is relevant, as it directly affects both the band gap tuning of the acceptors and their optical absorption. Generally, SM non-fullerene acceptors tend to have a planar structure;30,31 facilitating strong π–π interactions when co-facially orientated, which in turn gives the largest electronically interaction resulting in higher transport ability. Giving rise to the formation of structured domains (crystallinity) within films of the SM acceptors and donors, which is directly opposite to the behavior of the fullerene acceptors in BHJ devices, Lim et al.32 showed that using a planar, co-facially oriented, and highly ordered pentacene-based acceptor performed significantly worse than those exhibiting less planar and less co-facially orientation. The frontier orbitals of the two acceptors were similar while with various morphologies. Therefore to understand the properties of the SM based acceptors, considerations on the molecular geometry is essential.
Moreover, most of the state-of-art research results on single junction OPVs are with small active areas, for instance, no larger than 0.1 cm2, on rigid ITO glass substrates with a metal back electrode evaporated under high vacuum. The high energy consumption in ITO production and limited supply of indium have dramatically limited the development and commercialization of OPVs.33 Therefore it is essential to find alternatives to indium with preferably a solution-based ambient processable system, and the use of poly(3,4-ethylenedioxythiophene):polystyrene sulfonate (PEDOT:PSS) with a metal grid on flexible plastic (e.g. PET) substrates turns out to be the most readily scalable, facile substituent to ITO coated glass.33 Up to now, large area ITO- and vacuum-free OPVs fabrication processes such as roll-to-roll (R2R) slot-die coating, have been identified and explored as a large area processing technique by only few groups but with great successes.
Although OPVs containing small molecule acceptors have reached 8.4% for a bilayer design34 by slow sublimation techniques and over 7% for BHJ devices35 using ITO and calcium based electrodes, there is no guarantee on maintaining this high PV performance when upscaled to a roll-to-roll (R2R) production process from the laboratory-scale spin-coating and vacuum deposition techniques. Due to the inherent differences in processing, many of the achievements for small area OPVs are likely to prove difficult to reproduce using the R2R methods. In order to minimize/close this gap of knowledge, more efforts must be dedicated towards the testing of not only new organic donor but also novel promising SM acceptor materials in R2R fabricated OSCs.
To date, despite the increasing efficiency and success of the non-fullerene acceptors, there has been little work on transferring SMs from small area devices to medium or even large area devices. Chen et al.36 was the first to report the roll coated BHJ that showed the modest efficiency of 0.067%. Liu et al.37 reported the transfer of a SM acceptor together with P3HT, from spin coating with an efficiency of 3.17% (area 0.054 cm2) to an ITO-free flexible solar cell with a large area (∼1 cm2) performing at 0.65%. This is the best performing roll coated ITO-free flexible SM acceptor reported. In our previously described work38 we reported two simple non-fullerene acceptors Ph(DPP)2 and PhDMe(DPP)2 both exhibiting high Voc's exceeding 1.1 V from photovoltaic (PV) devices conventionally spin-coated and vacuum-deposited, revealing that slight modification in the molecular geometry showed large impact in both processing parameters (solubility) and efficiency.
In this report, we present design and synthesis of a novel three dimensional (3D) SM non-fullerene acceptor consisting of the same basic building blocks, having a center phenyl moiety flanked by three DPP moieties. This has been chosen in an effort to further reduce the crystallinity while not affecting the electron affinity. Furthermore, we investigate the transfer of the three SM acceptors (previously produced Ph(DPP)2 and PhDMe(DPP)2 and newly synthesized Ph(DPP)3) from a small area spin-coated device (10 mm2) to medium area roll-coated device (1 cm2), this reveal the large impact of the molecular geometry of the SM acceptors on the solar cell performance.
Experimental
Materials
All chemicals used were commercially available and used as received if not otherwise specified. 3-(5-Bromothiophen-2-yl)-2,5-bis(2-ethylhexyl)-6-(thiophen-2-yl)pyrrolo[3,4-c]pyrrole-1,4(2H,5H)-dione obtained from Suna Tech Inc. China and used without any additional purification. The materials Ph(DPP)2 and PhDMe(DPP)2 were synthesized following procedures reported in literature38 (as shown in Scheme 1).
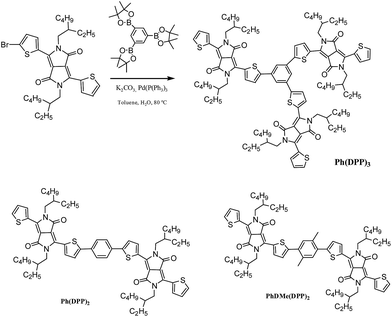 |
| Scheme 1 Synthesis of Ph(DPP)3 from mono-brominated DPP and 1,3,5-tribornic phenyl acid ester and structure of Ph(DPP)2 and PhDMe(DPP)2 which have been reported in our previous work.38 | |
Measurement
1H and 13C NMR spectra were measured using a Bruker Advance DMX 600 (600 MHz) nuclear magnetic resonance spectroscope. MALDI-TOF MS spectra were recorded on a Bruker Daltonics flex Analysis ultraflex TOF mass spectrometry. UV-visible absorption spectra were taken on a Shimadzu UV-2450 spectrophotometer. Differential scanning calorimetry (DSC) and thermal gravimetric analysis (TGA) was recorded on a TA Q20 V24.11 Build 124 differential scanning calorimeter nitrogen at a heating rate of 20 °C min−1. Cyclic voltammetry (CV) was done on a CHI 660C electrochemical workstation with Pt disk, Pt plate, and standard calomel electrode (SCE) as working electrode, counter electrode, and reference electrode, respectively, in a 0.1 M tetrabutylammonium hexafluorophosphate (Bu4NPF6) CHCl2 solution. The potential was calibrated against the ferrocene/ferrocenium redox couple (Fc/Fc+, 4.8 eV below the vacuum level). The highest occupied molecular orbital (HOMO) and the lowest unoccupied molecular orbital (LUMO) were estimated by the equations: HOMO = −(4.80 + Eonsetox) and LUMO = HOMO + EopticalBG. Atomic force microscopy (AFM) were acquired using NTegra Aura and Solver PRO AFMs (NT-MDT, Russia) operating in the intermittent contact mode with cantilevers Etalon HA-HR (17 N m−1) (ScanSens, Germany). The obtained topography images were processed using NT-MDT image analysis and WSxM software.39
Synthesis
Ph(DPP)3. To a three-necked round bottom flask were added 3-(5-bromothiophen-2-yl)-2,5-bis(2-ethylhexyl)-6-(thiophen-2-yl)pyrrolo[3,4-c]pyrrole-1,4(2H,5H)-dione (1.7 g, 2.82 mmol), 1,3,5-tris(4,4,5,5-tetramethyl-1,3,2-dioxaborolan-2-yl)benzene (0.28 g, 0.62 mmol), potassium carbonate (2.60 g, 18.8 mmol), deionized water (10 mL), ethanol (8 mL) and toluene (80 mL). The mixture was deoxygenated with nitrogen for 15 min. Pd(PPh3)4 (0.087 g, 0.075 mmol) was added under nitrogen. The mixture was heated at 80 °C for 24 h and then cooled down to room temperature. The mixture was extracted with dichloromethane (DCM) and washed with Brine. The organic phase was dried over anhydrous MgSO4 and filtered. After removing the solvent from the filtrate, the residue was purified by column chromatography on silica gel using DCM as eluent yielding a red solid (0.76 g, 74%).1H NMR (600 MHz, CDCl3, δ) 8.97 (d, J = 4.2 Hz, 3H), 8.96 (d, J = 3.6 Hz, 3H), 7.89 (s, 3H), 7.67 (d, J = 4.8 Hz, 3H), 7.60 (d, J = 3.6 Hz, 3H), 7.31–7.29 (m, 3H), 4.15–4.02 (m, 12H), 2.00–1.87 (m, 6H), 1.48–1.22 (m, 48H), 0.99–0.97 (t, J = 7.8, Hz, 9H), 0.93–0.85 (m, 17H); 13C NMR (600 MHz, CDCl3, d): 161.74, 161.69, 147.37, 140.70, 139.57, 136.43, 135.56, 135.22, 130.78, 129.94, 129.83, 128.51, 125.61, 123.76, 108.61, 108.05, 45.95, 39.28, 39.11, 30.34, 30.22, 28.54, 28.37, 23.78, 23.57, 23.09, 14.07, 14.04, 10.68, 10.53; MS (MALDI): m/z 1646.66.
Roll-coated solar cell and device characterization
All roll coated devices were printed on a substrate known as Flextrode comprising of PEDOT:PSS (Heraeus Clevios PH1000) and ZnO on barrier foil with a large scale roll-to-roll flexographic printed silver grid electrode, by using a mini-roll coater where all different layers were coated (with in situ heating) in one roll.40,41 The active layer SM (1, 2 and 3):P3HT (with the fixed D
:
A ratio 1
:
1.5), hole transport layer (HTL) PEDOT:PSS (Clevios P VP Al 4083) and the transporter Clevios F-010 were all slot-die coated as reported in detail by Andersen et al.42 and Angmo et al.43 The back silver electrode was applied by flexographic printing of a heat curing silver paste PV410 (Dupont) as previously reported.42 All cells possess an active area of 1 cm2.
PV device performance
Solar cells were measured with a Keithley 2400 sourcemeter under a KHS 575 solar simulator with an AM 1.5G 1000 W m−2 intensity. The external quantum efficiency (EQE) was performed using a QEX10 system (PV Measurements). The light beam-induced current (LBIC) was conducted on system described in the literature.44
Morphology characterization
Grazing Incidence Wide Angle X-ray Scattering (GIWAXS) measurements were performed with a Rigaku RU-200 rotating Cu anode as the source, operated at 50 kV, 200 mA. The X-ray beam point source was monochromatized and collimated (λ = 1.5418 Å) by a multilayer optic.45 Roll coated samples were fabricated by slot-die coating 30 g L−1 of P3HT
:
SM acceptors mixture (1
:
1.5) on a Flextrode substrate as described previously. Reference samples of SM acceptor were spin-coated on Si wafer, while the mixtures of P3HT
:
SM acceptor were spin-coated on ITO/glass substrate.
Results and discussion
Synthesis and characterization
The synthesized small molecule was successfully obtained via classical Pd(PPh3)4-catalyzed Suzuki coupling reaction between 3-(5-bromothiophen-2-yl)-2,5-bis(2-ethylhexyl)-6-(thiophen-2-yl)pyrrolo[3,4-c]pyrrole-1,4-(2H,5H)-dion and 1,3,5-tris(4,4,5,5-tetramethyl-1,3,2-dioxaborolan-2-yl)benzene under alkaline conditions and has been structural determined by 1H NMR, 13C NMR and MALDI-TOF MS, the results are noted under the synthetic procedure. The DSC/TGA curve of Ph(DPP)3 shows high purity of the material as there is only one melting peak at 227 °C and good thermal stability with its decomposition temperature at 394 °C, which is slightly higher than those reported for Ph(DPP)2 and PhDMe(DPP)2 due to its extended rigidity and larger molecule weight from the extra DPP moiety (see ESI Fig. 1†).38 Ph(DPP)3 showed a solubility of 10 mg mL−1 in dichloromethane and 20 mg mL−1 in chloroform at room temperature, being sufficient for solution processing, the Ph(DPP)3 solution appears light brick-red, similar to previously reported Ph(DPP)2 and PhDMe(DPP)2.
Molecular modeling
Theoretical computations were conducted to understand the geometrical structure by means of density functional theory (DFT) at the B3LYP level using the basis set 6-31G(d). To reduce the computational load, the side groups were reduced to methyl groups. As shown in Fig. 1a and b Ph(DPP)3 exhibited dihedral angles (between the core and the DPP units) of 24.6°, 26.6° and 27.2°, respectively, due to the mutual influences between the neighboring DPP units, with the consequence of forming a twisted, non-planar triangular structure.
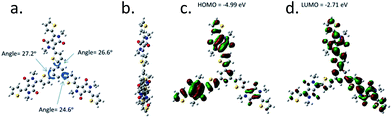 |
| Fig. 1 The geometrically optimized structure of Ph(DPP)3 (a) front view with the dihedral angles marked by arrows; (b) side view; (c) and (d) the HOMO and LUMO molecular frontier orbitals using DFT evaluation at the B3LYP/6-31G(d) level, respectively. | |
This reduced planarity is expected to result in a lower crystallinity compared to that of Ph(DPP)2 and PhDMe(DPP)2. Sherman et al.30 discussed that the nearly planar non-fullerene acceptors might be disadvantageous, mainly due to the larger phase separation. The spatial interactions among the three DPP moieties in Ph(DPP)3 are not taken into account as the alkyl modification of the DPP moiety was omitted. The molecular simulations of the HOMO and LUMO levels showed that the MOs are distributed along the conjugated system, similar to the findings of Arrechea et al.46 and Rutledge et al.47 which showed that symmetry affected the distribution of HOMO and LUMO, when a donor moiety is flanked by an acceptor moiety. This falls in line with the expectation that the HOMO is located on the electron rich moiety and LUMO on an electron poor moiety;48 but as Ph(DPP)3 only contains a weak donor in the form of a phenyl group,49 it is not expected to result in a large distribution difference. The energy levels of HOMO/LUMO were −4.99 eV/−2.71 eV, these MOs are shown in Fig. 1c and d. A slight mismatch is observed versus the values determined by CV, which is suspected to derive from the lack of spatial considerations in the simulation. Wei et al.50 found that, for a small molecule donor using the same method as this study, the LUMO exhibits the largest error, which is similar to these findings.
Photo physical and electronic properties
The solution and film absorbance of Ph(DPP)3 can be seen in Fig. 2. With the transition from solution to film, there is a red shift of the absorbance onset of 32 nm going from 605 nm in solution to 637 nm in film because of improved molecular aggregation in the condensed state. This absorbance onset of 637 nm corresponds to an optical band gap of 1.95 eV.
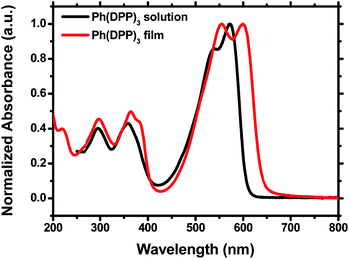 |
| Fig. 2 UV-vis absorbance spectrum of Ph(DPP)3 in solution (black) and Ph(DPP)3 in film (red). | |
The presence of peaks at 297 nm and 365 nm (in both solution and film) and the more intense peaks around 555 nm (in solution) and 598 nm (in film) are typical for the π–π* and intermolecular charge transfer (ICT) of the donor–acceptor linkage. This is especially interesting comparing the absorbance spectrum with the molecular modelling results. The peak of 598 nm could also be a vibronic vibrations peak, indicating an efficient π–π packing; although this is counter intuitive as these Ph(DPP)3 molecules are expected to show a low degree of packing as a function of geometry of the devices. This is further confirmed by the shoulder peak emerging at about 525 nm in the film due to stronger intermolecular π–π overlapping, suggesting higher degree of molecular planarity, compared to our previously reported works.38 Chen et al.51 showed that a perylene diimide based small molecule acceptor with a coordinated tetrahedral configuration resulted in a large spatial interactions and observed a small increase in absorbance going from solution to film, and also a relative increase of a secondary peak, which is parallel to our observations. This indicates the spatial interactions of the Ph(DPP3) and supports the notion that there is a low π–π interaction.
Cyclic voltammetry has been conducted to determine the position of the frontier orbitals, and the results can be seen in Table 1. The orbitals were determined to be −5.22 eV and −3.27 eV for the HOMO and LUMO, respectively (the CV diagram can be seen in ESI Fig. 2†). The HOMO/LUMO levels of P3HT have been estimated to be located at −5.24/−3.20 eV, respectively.38
Table 1 Summarization of electron and photo physical properties of Ph(DPP)3, Ph(DPP)2 and PhDMe(DPP)2 (reported in ref. 38)
Sample name |
Absorbance onset [nm] |
Bandgap [eV] |
Oxidation onset [V] |
HOMO level [eV] (calc.) |
LUMO level [eV] (calc.) |
Electron mobilitya [cm2 V−1 s−1] |
Annealed spin coated film. |
Ph(DPP)3 |
637 |
1.95 |
0.825 |
−5.22 (−4.99) |
−3.27 (−2.71) |
1.1 × 10−10 |
Ph(DPP)2 |
707 |
1.75 |
— |
−5.16 |
−3.52 |
1.2 × 10−11 |
PhDMe(DPP)2 |
676 |
1.82 |
— |
−5.18 |
−3.65 |
8 × 10−10 |
The relative small energy difference between the LUMOdonor and LUMOacceptor might give raise to a reduced charge separation, as the LUMO–LUMO difference is the driving force for the separation and in literature the optimal difference have been pronounced to be 0.3 eV.52 While our previously reported LUMO level for Ph(DPP)2 and PhDMe(DPP)2 (−3.52 and −3.65 eV, respectively, also as shown in Table 1) fits that for P3HT better than Ph(DPP)3 does, which could lead to better charge separation.
The space charge limited current (SCLC) method was employed to determine the charge carrier mobility of the active layers containing both the P3HT and the three DPP non-fullerene acceptor molecules. Devices with a structure of ITO/Al/P3HT:Ph(DPP)3/Al were fabricated for measuring the electron mobility. The mobility was determined to be 1.1 × 10−10 cm2 V−1 s−1, which lies in between those of Ph(DPP)2 and PhDMe(DPP)2. It is just such low electron mobilities that result in a large degree of recombination in the active layer and therefore be responsible for their rather low current densities, which in turn lower the PCE of the devices. In the spin-coated small area OPV devices, PhDMe(DPP)2 becomes the best acceptor materials mainly due to its better aligned LUMO level, higher charge carrier mobility, and higher FFs than the other two as concluded in our previous paper.38
Device performance
In order to investigate the photovoltaic properties of OPV devices from the DPP-based series of non-fullerene acceptors in large area fabricated under ITO- and vacuum-free conditions, inverted geometry solar cells were made using a mini-roll coater setup. In Fig. 3 the best performing devices (under a single exposure) are shown for the three different SM Ph(DPP)3, Ph(DPP)2 and PhDMe(DPP)2. It is clear that the Voc of these devices are rather low compared to the Voc we reported earlier.38 For the spin coated devices there was only a small difference between the Voc (spanning from 1.10 to 1.13 V for Ph(DPP)2 and PhDMe(DPP)2, respectively). There were large differences between the Voc of the roll-coated devices, where the Voc for Ph(DPP)2 and Ph(DPP)3 are approximately 0.65–0.70 V while PhDMe(DPP)2 only has a Voc of 0.40 V, which is under half reported previously. This difference is most likely due to the recombination mechanisms in the active layer of the device.
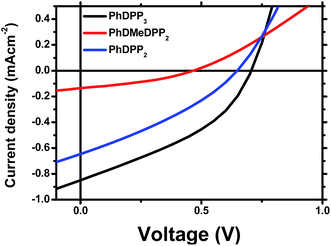 |
| Fig. 3 I–V characteristics of the OSC with the active layer consisting of P3HT : small molecule acceptor in the ratio 1 : 1.5 (Ph(DPP)3 (black), PhDMe(DPP)2 (red) and Ph(DPP)2 (blue)). | |
The I–V performance of the roll-coated (RC) devices in comparison with the spincoated cells can be seen in Table 2. We found higher Jsc's spanning from 0.15 mA cm−2 to 0.83 mA cm−2 obtained when comparing to those of the as-cast spincoated devices ranging from 0.13 to 0.27 mA cm−2. While FF of the devices fabricated on RC all exhibit almost 50% higher value from ca. 26% to around 32–36%. Both the elevated Jsc and FF could be explained by a more efficient charge separation at D–A interfaces and charge transport, and both of these could be ascribed to the improved thin film morphology caused by RC fabrication method. Whilst PhDMe(DPP)2 performed best for the spincoated device with an efficiency of 0.65% compared to the other two. The EQE of the best performing device with each population of the RC SM can be seen in Fig. 4, and as expected the PhDMe(DPP)2 performs significantly worse than Ph(DPP)2 and Ph(DPP)3. The maximum EQE for PhDMe(DPP)2 is around 1% (at 520 nm) compared to the approximately 4.5% (at 510 nm) and 5.25% (at 530 nm) for Ph(DPP)2 and Ph(DPP)3, respectively. It is clear that the reason for the much better performance of the Ph(DPP)3 is found in the charge transfer, as the maximum EQE around 500 nm corresponds to the absorbance maximum of P3HT, while the EQE onset is higher than absorbance onset of P3HT.48 This difference is therefore attributed to the absorbance of the SM acceptors.
Table 2 The PV performance of the roll coated OSC, all in the ratio donor
:
acceptor 1
:
1.5. The value presented are all averages of 4 measured devices, (* annealed at 90 °C for 10 min, ** thermally treated at 140 °C for 2 min)
SM acceptor |
Spin-coated OSCs* (ref. 38) |
Roll-coated OSCs** |
Jsc [mA cm−2] |
Voc [V] |
FF [%] |
PCE [%] (best) |
Jsc [mA cm−2] |
Voc [V] |
FF [%] |
PCE [%] |
PCE [%] (best) |
Ph(DPP)3 |
1.17 |
1.07 |
24.8 |
0.31 |
0.83 ± 0.04 |
0.69 ± 0.03 |
36.4 ± 2.6 |
0.20 ± 0.03 |
0.23 |
Ph(DPP)2 |
1.45 |
1.10 |
30.0 |
0.48 |
0.58 ± 0.07 |
0.65 ± 0.01 |
32.7 ± 0.5 |
0.12 ± 0.02 |
0.14 |
PhDMe(DPP)2 |
1.54 |
1.13 |
37.3 |
0.65 |
0.15 ± 0.01 |
0.40 ± 0.05 |
32.3 ± 2.0 |
0.02 ± 0.00 |
0.02 |
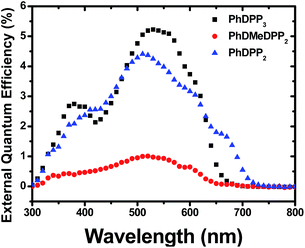 |
| Fig. 4 External quantum efficiency of the three best performing devices with the three different small molecules Ph(DPP)3 (black square), PhDMe(DPP)2 (red circle) and Ph(DPP)2 (blue triangle). | |
The current density of the RC devices indicates, that Ph(DPP)3 has a better charge transfer than the other two, which we speculate to more efficient charge transport and also probably more efficient charge separation due to its higher FF, meaning its improved thin film morphology, even though its LUMO level does not match that of the donor material (P3HT) well for efficient charge separation. We further investigated the possible formation of dead zones of the OSCs by means of LBIC maps, and the best performing cell of each of the three small molecule acceptor based devices are shown in Fig. 5. Despite being the worst performing cell the PhDMe(DPP)2 has the most homogenous response, whilst Ph(DPP)2 and Ph(DPP)3 both have areas with a low current response. The red zones indicate the highest light induced current intensity, whereas the green is medium response and blue is no response.
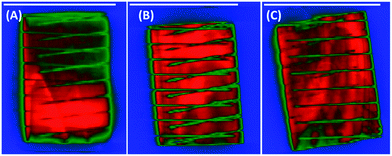 |
| Fig. 5 Light beam-induced current image of OPVs containing the three different SM containing devices; Ph(DPP)3 (A), PhDMe(DPP)2 (B) and Ph(DPP)2 (C). | |
As shown in the Table 3, the efficiency increases as function of exposure time to light. The efficiency increases from 0.02% to 0.04% for PhDMe(DPP)2, 0.14% to 0.43% for Ph(DPP)2 and 0.23% to 0.54% for Ph(DPP)3. This is due to the increase in both voltage and current density, especially in current density. Lafalce et al.53 showed that this phenomenon of an increasing efficiency as function of the exposure of photons is three sided, one part is the photo induced desorption of O2, the second part being trap filling, and thirdly thermal activation of trapped charges.
Table 3 I–V performance of the roll-coated SM acceptor solar cells after photo annealing
SMa acceptor |
Time [s] |
Jsc [mA cm−2] |
Voc [V] |
FF [%] |
PCE [%] |
Small molecule. |
Ph(DPP)3 |
16 750 |
1.48 |
0.82 |
35.8 |
0.54 |
PhDMe(DPP)2 |
564 |
0.18 |
0.54 |
32.6 |
0.04 |
Ph(DPP)2 |
16 470 |
1.22 |
0.80 |
35.2 |
0.43 |
According to Lafalce et al.53 the initial illumination affords desorption of oxygen resulting in a reversible oxidation of the active layer (especially the P3HT) resulting in a p-doped active layer. This is counteracted by the irreversible oxidation of the photo active polymer that in turn reduces and finally destroys the device. This process can be seen rather clearly in Fig. 6, where it is evident there is an initial increase in the efficiency of ∼50%, followed by a rather large drop in efficiency. The duration of this process known as burn-in is approximately 10 days, at which the downwards slope of the efficiency stabilizes. We ascribe the large increase of efficiency to the reversible oxidation (thus p-doping) of the polymer, most likely due to the manufacturing of the device being conducted under ambient conditions.
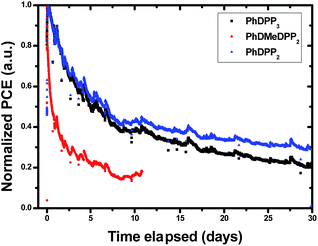 |
| Fig. 6 The normalized PCE (%) as a function of time (days) for devices consisting of P3HT and a given small molecule (Ph(DPP)3 (black square), PhDMe(DPP)2 (red circle) and Ph(DPP)2 (blue triangle)). PhDMe(DPP)2 showed a terminal defect at approximately the 11th day. | |
Morphology studies via AFM and GIWAXS
Fig. 7 presents the topographic and phase AFM images of the slot die-coated films of Ph(DPP)3, PhDMe(DPP)2 and Ph(DPP)2. It is clear that the surface morphology of the three films are rather different, for the PhDMe(DPP)2 (a and d) the peak-to-peak variation is 86.5 nm. This is rather high compared to (b and e) Ph(DPP)2 and (c and f) Ph(DPP)3 with much less peak-to-peak variation of 39.5 nm and 29.3 nm, respectively. This variation is much less pronounced for the RMS as it is 2.7 nm for Ph(DPP)3, 4.6 nm Ph(DPP)2 and 8.2 nm for PhDMe(DPP)2, indicating a large degree of phase separation. This large separation can somehow account for the low overall efficiency of the PhDMe(DPP)2. We speculate that the fast evaporation of spin coating versus the slower evaporation of the roll coated film is the major difference, with regards to the difference in such different processing methods. In roll-coating, Ph(DPP)3 is the best performing acceptor, and PhDMe(DPP)2 is the worst, while the opposite is true for the spin coated devices. Overall the difference in FF, Jsc and Voc can primarily be ascribed to the large size difference and the different geometry of devices. To further understand the crystal structure and possible π–π stacking of either P3HT donor polymers or SM acceptors in the thin film coated on Si wafer and ITO substrates, we performed 2D X-ray scattering in grazing incidence geometry (GIWAXS).54–56 The measurements were taken with the X-ray incident angle below the critical angle for total reflection from the substrate to minimize background scattering. The packing along the side chains of the P3HT crystal is denoted as the a-axis, (h00), and the π–π stacking direction within the P3HT crystal is denoted as b-axis, (0k0).
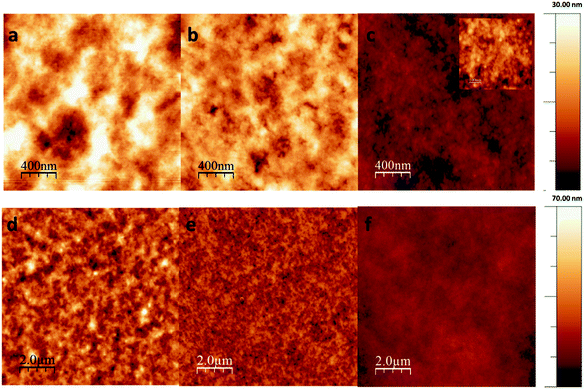 |
| Fig. 7 AFM topography of active layer blends on roll-coated Flextrode (2 × 2 μm (upper row) and 10 × 10 μm (lower row) scale): (a and d) PhDMe(DPP)2, (b and e) Ph(DPP)2 and (c and f) Ph(DPP)3. The vertical scale for the images in the upper and lower rows is 30 nm and 70 nm, respectively. The inset shows an enlarged section of the Ph(DPP)3 surface, presented with a vertical scale of 8 nm. | |
From the GIWAXS results illustrated in Fig. 8, we can see that when only SM acceptor materials are coated on Si wafer substrates, Ph(DPP)3 is totally X-ray amorphous (Fig. 8(A1)), which is in accordance with the results of interfered co-planarity among the three DPP moieties via computer modelling; Ph(DPP)2 shows some degree of crystallinity intermediate between Ph(DPP)3 and PhDMe(DPP)2 (Fig. 8(A2)). Similar to what is seen for typical thiophene polymers, the planar Ph(DPP)2 shows a lamellar peak, but corresponding to a slightly shorter distance than for P3HT, at about 14 Å. Some mixed index peaks indicate 3D ordering. PhDMe(DPP)2 presents the best crystallinity and texturing (Fig. 8(A3)), showing clear 3D ordering. A similar lamellar peak on the surface normal is found as seen for Ph(DPP)2, whereas PhDMe(DPP)2 also shows peak in the substrate plane which could represent π–π stacking (010). When being coated on ITO substrates, both two components within P3HT/Ph(DPP)2 and P3HT/PhDMe(DPP)2 present crystalline structure at the same time (as shown in Fig. 8(B2) and (B3)): for Ph(DPP)3 and P3HT, they show only one family of diffraction planes (100), textured along the surface normal, corresponding to a stacking distance of about 17 Å, slightly longer than what is normally seen for neat P3HT. The (100) lamellar peaks of both Ph(DPP)2 and PhDMe(DPP)2, and P3HT appears to be strongly overlapped in the measurement on ITO substrates, with the combined peak covering 14–15 Å spacing, which may indicate that SMs are close intercalated with P3HT. For coating on ITO, Ph(DPP)2 behaves very similar to PhDMe(DPP)2 while not as well crystallized as the latter. In general, devices spun on ITO showed the order of overall crystallinity of the blended P3HT and SM acceptor materials as: (P3HT:PhDMe(DPP)2) > (P3HT:Ph(DPP)2) > (P3HT:Ph(DPP)3), which is the result of their molecular geometry and benefits the charge transport, thus the same order of their PV performance, PCE, is found. On the contrary, reversed order of power conversion efficiency of the devices roll-coated on Flextrode surface was observed, which we attributed to the possible better coating properties in roll-coating because of the amorphous nature of Ph(DPP)3.
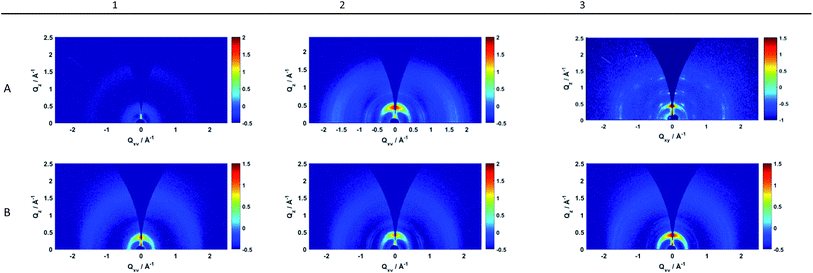 |
| Fig. 8 GIWAXS data represented in reciprocal space coordinates for (A1) Ph(DPP)3 roll-coated on Si wafer; (A2) Ph(DPP)2 roll-coated on Si wafer; (A3) PhDMe(DPP)2 roll-coated on Si wafer; (B1) P3HT : Ph(DPP)3 (1 : 1.5) roll-coated on ITO; (B2) P3HT : Ph(DPP)2 (1 : 1.5) roll-coated on ITO; (B3) P3HT : PhDMe(DPP)2 (1 : 1.5) roll-coated on ITO. Intensities are on a false color log scale to emphasize weak scattering. | |
Conclusion
Based on our recently reported two DPP based non-fullerene acceptors Ph(DPP)2 and PhDMe(DPP)2, we further synthesized a 3D acceptor Ph(DPP)3 and characterized these three homologous series with comparable chemical structure but different molecular geometries. Due to their different aggregation states and crystallization properties, the three compounds show different light absorption, energy levels, electron mobility and morphology when blended with P3HT. For PhDMe(DPP)2, when two methyl groups are attached to 2,5-positions of phenyl group, the dihedral angle between the core and the DPP units increases to 44.46° in the ground state due to the steric hindrance. Therefore, PhDMe(DPP)2 shows more blue-shifted absorption bands, higher electron mobility, and better miscibility with P3HT than Ph(DPP)2 and Ph(DPP)3 and shows best performance of 0.65% paired with P3HT, twice as good as the P3HT/Ph(DPP)3 based device, when OSCs were fabricated on the ITO-coated glass substrates with spin-coating process. When fabricated as roll coated, large area, ITO- and vacuum-free flexible solar cells, the P3HT/Ph(DPP)3 based device shows the best performance of 0.54%, which is 10 times higher than the P3HT/PhDMe(DPP)2 based device. Our preliminary results highlight the influence of geometry structure for non-fullerene acceptors.
Acknowledgements
The authors would like to gratefully acknowledge the financial support from the Innovation Fund Denmark (IFD) through the WAPART project (Water-based particulate approach to organic photovoltaics with controlled morphology, 0603-00493B), the Danish National Research Foundation (DNRF) for the Danish-Chinese Center for Organic based Photovoltaic Cells with Morphological Control, and the National Natural Science Foundation of China (Nos. 21474088, 51261130582, 91233114), Zhejiang Province Natural Science Foundation (No. LR13E030001). The work was also partly supported by 863 program (No. 2011AA050520) and 973 program (No. 2014CB643503). Support from the Sino-Danish Center for Education and Research (SDC) is also fully acknowledged.
References
- V. A. Trukhanov and D. Y. Paraschuk, Polym. Sci., Ser. C, 2014, 56, 72–83 CrossRef CAS.
- P. Sonar, J. P. Fong Lim and K. L. Chan, Energy Environ. Sci., 2011, 4, 1558 CAS.
- G. J. Dutton and S. W. Robey, Phys. Chem. Chem. Phys., 2015, 17, 15953–15962 RSC.
- Y. He, H.-Y. Chen, J. Hou and Y. Li, J. Am. Chem. Soc., 2010, 132, 1377–1382 CrossRef CAS PubMed.
- Y. He and Y. Li, Phys. Chem. Chem. Phys., 2011, 13, 1970–1983 RSC.
- C.-Z. Li, H.-L. Yip and A. K.-Y. Jen, J. Mater. Chem., 2012, 22, 4161 RSC.
- J.-L. Wang, Z. He, H. Wu, Y. Cao and J. Pei, New J. Chem., 2012, 36, 1583 RSC.
- J. T. Bloking, T. Giovenzana, A. T. Higgs, A. J. Ponec, E. T. Hoke, K. Vandewal, S. Ko, Z. Bao, A. Sellinger and M. D. McGehee, Adv. Energy Mater., 2014, 4, 1301426 Search PubMed.
- A. Sharenko, C. M. Proctor, T. S. van der Poll, Z. B. Henson, T.-Q. Nguyen and G. C. Bazan, Adv. Mater., 2013, 25, 4403–4406 CrossRef CAS PubMed.
- H. Li, T. Earmme, G. Ren, A. Saeki, S. Yoshikawa, N. M. Murari, S. Subramaniyan, M. J. Crane, S. Seki and S. A. Jenekhe, J. Am. Chem. Soc., 2014, 136, 14589–14597 CrossRef CAS PubMed.
- X. Zhao and X. Zhan, Chem. Soc. Rev., 2011, 40, 3728 RSC.
- Y. Lin and X. Zhan, Mater. Horiz., 2014, 1, 470 RSC.
- H. Bai, Y. Wang, P. Cheng, J. Wang, Y. Wu, J. Hou and X. Zhan, J. Mater. Chem. A, 2015, 3, 1910–1914 CAS.
- Y. Lin, Y. Wang, J. Wang, J. Hou, Y. Li, D. Zhu and X. Zhan, Adv. Mater., 2014, 26, 5137–5142 CrossRef CAS PubMed.
- X. Zhang, C. Zhan and J. Yao, Chem. Mater., 2015, 27, 166–173 CrossRef CAS.
- W. Liu, S. Liu, N. K. Zawacka, T. R. Andersen, P. Cheng, L. Fu, M. Chen, W. Fu, E. Bundgaard, M. Jørgensen, X. Zhan, F. C. Krebs and H. Chen, J. Mater. Chem. A, 2014, 2, 19809–19814 CAS.
- S. Li, W. Liu, M. Shi, J. Mai, T.-K. Lau, J. Wan, X. Lu, C.-Z. Li and H. Chen, Energy Environ. Sci., 2016, 9, 604–610 CAS.
- X. Zhang, Z. Lu, L. Ye, C. Zhan, J. Hou, S. Zhang, B. Jiang, Y. Zhao, J. Huang, S. Zhang, Y. Liu, Q. Shi, Y. Liu and J. Yao, Adv. Mater., 2013, 25, 5791–5797 CrossRef CAS PubMed.
- P. Cheng, L. Ye, X. Zhao, J. Hou, Y. Li and X. Zhan, Energy Environ. Sci., 2014, 7, 1351–1356 CAS.
- W. Jiang, L. Ye, X. Li, C. Xiao, F. Tan, W. Zhao, J. Hou and Z. Wang, Chem. Commun., 2014, 50, 1024–1026 RSC.
- L. Ye, W. Jiang, W. Zhao, S. Zhang, D. Qian, Z. Wang and J. Hou, Small, 2014, 10, 4658–4663 CrossRef CAS PubMed.
- Y. Lin, J. Wang, Z.-G. Zhang, H. Bai, Y. Li, D. Zhu and X. Zhan, Adv. Mater., 2015, 27, 1170–1174 CrossRef CAS PubMed.
- Y. Lin, Z.-G. Zhang, H. Bai, J. Wang, Y. Yao, Y. Li, D. Zhu and X. Zhan, Energy Environ. Sci., 2015, 8, 610–616 CAS.
- S. Holliday, R. S. Ashraf, C. B. Nielsen, M. Kirkus, J. A. Röhr, C.-H. Tan, E. Collado-Fregoso, A.-C. Knall, J. R. Durrant, J. Nelson and I. McCulloch, J. Am. Chem. Soc., 2015, 137, 898–904 CrossRef CAS PubMed.
- Y. Liu, C. Mu, K. Jiang, J. Zhao, Y. Li, L. Zhang, Z. Li, J. Y. L. Lai, H. Hu, T. Ma, R. Hu, D. Yu, X. Huang, B. Z. Tang and H. Yan, Adv. Mater., 2015, 27, 1015–1020 CrossRef CAS PubMed.
- J. Zhao, Y. Li, H. Lin, Y. Liu, K. Jiang, C. Mu, T. Ma, J. Y. Lin Lai, H. Hu, D. Yu and H. Yan, Energy Environ. Sci., 2015, 8, 520–525 CAS.
- Y. Zang, C.-Z. Li, C.-C. Chueh, S. T. Williams, W. Jiang, Z.-H. Wang, J.-S. Yu and A. K.-Y. Jen, Adv. Mater., 2014, 26, 5708–5714 CrossRef CAS PubMed.
- J. D. Yuen, J. Fan, J. Seifter, B. Lim, R. Hufschmid, A. J. Heeger and F. Wudl, J. Am. Chem. Soc., 2011, 133, 20799–20807 CrossRef CAS PubMed.
- H. Bronstein, Z. Chen, R. S. Ashraf, W. Zhang, J. Du, J. R. Durrant, P. Shakya Tuladhar, K. Song, S. E. Watkins, Y. Geerts, M. M. Wienk, R. A. J. Janssen, T. Anthopoulos, H. Sirringhaus, M. Heeney and I. McCulloch, J. Am. Chem. Soc., 2011, 133, 3272–3275 CrossRef CAS PubMed.
- J. B. Sherman, B. Purushothaman, S. R. Parkin, C. Kim, S. Collins, J. Anthony, T.-Q. Nguyen and M. L. Chabinyc, J. Mater. Chem. A, 2015, 3, 9989–9998 CAS.
- A. J. Ferguson, J. L. Blackburn and N. Kopidakis, Mater. Lett., 2013, 90, 115–125 CrossRef CAS.
- Y.-F. Lim, Y. Shu, S. R. Parkin, J. E. Anthony and G. G. Malliaras, J. Mater. Chem., 2009, 19, 3049 RSC.
- F. C. Krebs, Sol. Energy Mater. Sol. Cells, 2009, 93, 1636–1641 CrossRef CAS.
- K. Cnops, B. P. Rand, D. Cheyns, B. Verreet, M. A. Empl and P. Heremans, Nat. Commun., 2014, 5, 1–6 Search PubMed.
- D. Sun, D. Meng, Y. Cai, B. Fan, Y. Li, W. Jiang, L. Huo, Y. Sun and Z. Wang, J. Am. Chem. Soc., 2015, 137, 11156–11162 CrossRef CAS PubMed.
- M. R. Chen, C. C. Fan, T. R. Andersen, H. F. Dam, W. F. Fu, Y. Z. Lin, E. Bundgaard, F. C. Krebs, X. W. Zhan and H. Z. Chen, Synth. Met., 2014, 195, 299–305 CrossRef CAS.
- W. Liu, H. Shi, T. R. Andersen, N. K. Zawacka, P. Cheng, E. Bundgaard, M. Shi, X. Zhan, F. C. Krebs and H. Chen, RSC Adv., 2015, 5, 36001–36006 RSC.
- F. Zhang, R. G. Brandt, Z. Gu, S. Wu, T. R. Andersen, M. Shi, D. Yu and H. Chen, Synth. Met., 2015, 203, 249–254 CrossRef CAS.
- I. Horcas, R. Fernández, J. M. Gómez-Rodríguez, J. Colchero, J. Gómez-Herrero and A. M. Baro, Rev. Sci. Instrum., 2007, 78, 013705 CrossRef CAS PubMed.
- H. F. Dam and F. C. Krebs, Sol. Energy Mater. Sol. Cells, 2012, 97, 191–196 CrossRef CAS.
- J. E. Carlé, T. R. Andersen, M. Helgesen, E. Bundgaard, M. Jørgensen and F. C. Krebs, Sol. Energy Mater. Sol. Cells, 2013, 108, 126–128 CrossRef.
- T. R. Andersen, H. F. Dam, B. Andreasen, M. Hösel, M. V. Madsen, S. a. Gevorgyan, R. R. Søndergaard, M. Jørgensen and F. C. Krebs, Sol. Energy Mater. Sol. Cells, 2014, 120, 735–743 CrossRef CAS.
- D. Angmo, S. a. Gevorgyan, T. T. Larsen-Olsen, R. R. Søndergaard, M. Hösel, M. Jørgensen, R. Gupta, G. U. Kulkarni and F. C. Krebs, Org. Electron., 2013, 14, 984–994 CrossRef CAS.
- F. C. Krebs and M. Jørgensen, Adv. Opt. Mater., 2014, 2, 465–477 CrossRef CAS.
- D. Apitz, R. P. Bertram, N. Benter, W. Hieringer, J. W. Andreasen, M. M. Nielsen, P. M. Johansen and K. Buse, Phys. Rev. E: Stat., Nonlinear, Soft Matter Phys., 2005, 72, 036610 CrossRef CAS PubMed.
- S. Arrechea, A. Molina-Ontoria, A. Aljarilla, P. de la Cruz, F. Langa and L. Echegoyen, Dyes Pigm., 2015, 121, 109–117 CrossRef CAS.
- L. R. Rutledge, S. M. McAfee and G. C. Welch, J. Phys. Chem. A, 2014, 118, 7939–7951 CrossRef CAS PubMed.
- R. G. Brandt, W. Yue, T. R. Andersen, T. T. Larsen-Olsen, M. Hinge, E. Bundgaard, F. C. Krebs and D. Yu, J. Mater. Chem. C, 2015, 1633–1639 RSC.
- S.-W. Yang, A. Elangovan and T.-I. Ho, Photochem. Photobiol. Sci., 2005, 4, 327 CAS.
- H. Wei, W. Chen, L. Han, T. Wang, X. Bao, X. Li, J. Liu, Y. Zhou and R. Yang, Chem.–Asian J., 2015, 10, 1791–1798 CrossRef CAS PubMed.
- W. Chen, X. Yang, G. Long, X. Wan, Y. Chen and Q. Zhang, J. Mater. Chem. C, 2015, 3, 4698–4705 RSC.
- T. Ameri, G. Dennler, C. Lungenschmied and C. J. Brabec, Energy Environ. Sci., 2009, 2, 347 CAS.
- E. Lafalce, P. Toglia, J. E. Lewis and X. Jiang, J. Appl. Phys., 2014, 115, 244511 CrossRef.
- A. P. L. Böttiger, M. Jørgensen, A. Menzel, F. C. Krebs and J. W. Andreasen, J. Mater. Chem., 2012, 22, 22501 RSC.
- Z. Ma, W. Sun, S. Himmelberger, K. Vandewal, Z. Tang, J. Bergqvist, A. Salleo, J. W. Andreasen, O. Inganäs, M. R. Andersson, C. Müller, F. Zhang and E. Wang, Energy Environ. Sci., 2014, 7, 361–369 CAS.
- N. Espinosa, H. F. Dam, D. M. Tanenbaum, J. W. Andreasen, M. Jørgensen and F. C. Krebs, Materials, 2011, 4, 169–182 CrossRef CAS.
Footnotes |
† Electronic supplementary information (ESI) available. See DOI: 10.1039/c6ra06898g |
‡ Current address: Centre for Organic Electronics, University of Newcastle, University Drive, Callaghan, NSW 2308, Australia. |
|
This journal is © The Royal Society of Chemistry 2016 |