DOI:
10.1039/C6RA06614C
(Paper)
RSC Adv., 2016,
6, 58371-58379
Accessible fabrication of Bi2MoO6/BiOCl for effectively conducting thermally-responsive catalytic decontamination of model pollutants
Received
12th March 2016
, Accepted 6th June 2016
First published on 7th June 2016
Abstract
Bi2MoO6/BiOCl (BMB) with thermally-responsive catalytic properties was synthesized by a one-step hydrothermal approach under a low-temperature environment. The influence of initial concentration, reaction temperature, pH, and ions on the thermally-responsive catalytic degradation of methylene blue (MB) was systematically investigated. The experimental evidence shows that an excellent thermally-responsive catalytic activity on decomposing MB is found using BMB under low-heat excitation. Diverse ions introduced toward bare solutions could significantly improve the succeeding degradation effect. The degradation products from thermally-responsive catalytic reactions were identified by GC-MS, demonstrating that this pattern possesses a substantial ability to achieve the destruction of water pollutants. Recycling evaluation reveals that the total degradation rate of MB over BMB remains at a level of >70% even after three use cycles, implying that BMB could be reused without severe loss of activity. A rational mechanism responsible for deteriorating pollutants is also proposed. Accordingly, BMB triggered with heat may provide a viable alternative catalyst for the abatement of organic contaminants from future chemical industries.
1. Introduction
Industrial plants have generated increasing amounts of wastewater, causing severe environmental problems in our modern industrialized society. In particular, organic pollutants derived from effluent, highly toxic for human beings, have become one of the most serious global environmental issues nowadays.1–3 To date, the mitigation methods concerning dye substances have developed a variety of possible routes such as adsorption,4 sonolysis,5 Fenton-like reactions,6 biological degradations7 and photocatalysis.8,9 Ultimately, more and more attention is focused on photocatalysis as the mainstream treatment method for hazardous organics, and may provide a promising approach to the implementation of the large-scale removal of persistent contaminants at different levels in waste water.10,11 It is well-known that TiO2 has been widely employed for photocatalytic applications owing to its cheapness, non-toxicity, and stability.12,13 However, it has greatly limited the practical applications of solar energy due to its low visible-light response and high photogenerated charge-carrier combination.14–16 In this case, by means of a deep investigation of photocatalysis, numerous improved techniques are explored for other novel efficient photocatalysts, for instance, Bi-based semiconductor materials, which have a unique layered structure and high chemical activity, have attracted great concern.17
Bismuth oxyhalides has a structure-dependent photocatalytic property that favors the transfer of electrons and holes generated inside the crystal surface so as to promote electron/hole separation.18 Nevertheless, BiOCl is a wide band-gap (Eg = 3.4 eV) semiconductor and can only absorb ultraviolet light, which accounts for less than 5% of solar energy, leading to a poor photocatalytic performance under visible-light irradiation.19–21 Thus, a reasonable modification of BiOCl with a narrow band-gap may be an effective way to obtain the visible-light-induced photocatalytic activity. On the other hand, many kinds of heterojunction semiconductors (such as antisotype p–n, isotype n–n, and crystal-phase junctions)22–26 have been extended to the field of photocatalysis for environmental decontamination. Among these, the bismuth-based photocatalyst, Bi2MoO6, is thought to be an ideal photocatalyst from the viewpoint of visible light. However, the high recombination rate of electron–hole pairs that exist in Bi2MoO6 badly hinders its application in pollutant decomposition in a way similar to that of TiO2.27
Any physical or chemical changes in materials are attributed to corresponding environmental factors such as light, heat, moisture, chemical condition and biological activity.28,29 To overcome the shortcomings of photocatalytic property above, here we offer a thermally-responsive catalytic route under near room temperature instead of the light-driven excitation to make up the shortcomings. Inspired by the synthesis of many other metal oxide-based heterojunction composites, BiOCl heterojunction composites covered with amorphous Bi2MoO6 are highly desirable and anticipated as potential superior catalysts because of the high interfacial charge-transfer ability between those components. Since the overlapped energy band from BMB composite may make the electrons and holes more easily transfer and jump in favor of joining in the thermally-responsive catalytic reactions. Herein, we report on a facile one-step method to synthesize hierarchical BiOCl covered with amorphous Bi2MoO6 composites to achieve a possible treatment for organic dyes. To the best of our knowledge, there are a few reports on several photocatalysts, such as BiOCl/Bi2MoO6,30 BiOCl/Bi2WO6,31 and AgCl/BiOCl,32 that possess superior photocatalytic activities in the abatement for contaminants. However, no information about a thermally-responsive catalytic degradation course using BiOCl covered with amorphous Bi2MoO6 has been reported previously. In detail, in this work we prepared the heat-sensitive Bi2MoO6/BiOCl (BMB) to accomplish the degradation of organics in the dark, demonstrating its remarkable thermally-responsive catalytic potential. Meanwhile, the effect of the existence of PO43−, CO32−, Cl−, SO42− and NO3− on thermally-responsive catalytic degradation of methylene blue (MB) was also investigated, including the exploration of the main by-products after the degradation. In conclusion, it may be a very promising approach to deal with dye-contaminated wastewater and provide new insights into the degradation of water pollutants by means of an ambient-heat stimulus catalysis.
2. Experimental
2.1. Materials
Bismuth nitrate (Bi(NO3)3·5H2O), ammonium molybdate ((NH4)6Mo7O24·4H2O), hydrochloric acid (HCl), and methylene blue (MB) were purchased from Chengdu Kelong Chemical Reagent Factory, China. All reagents were analytical grade and use without further purification.
2.2. Synthesis of Bi2MoO6/BiOCl
Bi2MoO6/BiOCl (BMB) samples were synthesized using a one-step hydrothermal method. In a typical synthesis process, Bi(NO3)3·5H2O (12.3 g) and (NH4)6Mo7O24·4H2O (4.42 g) were firstly dissolved in 100 mL deionized water under magnetic stirring. After stirring for 10 min, HCl was added dropwise to adjust the pH of the above mixed solution to 7.2, and the solution became brown and was then transferred into a Teflon-lined stainless steel autoclave at 70 °C for 10 h. Subsequently, the autoclave was cooled to room temperature naturally. The products were washed several times by deionized water thoroughly, and dried at 60 °C for 10 h.
2.3. Adsorption course and thermally-responsive catalytic reactions
Considering the effect of physical adsorption on thermally-responsive catalytic reactions, the dark adsorption experiments were engaged until a constant value in terms of the absorbance of corresponding pollutants was achieved, and subsequently thermally-responsive catalytic processes were employed. Thermally-responsive catalytic activities of the samples were evaluated by thermodegradation of methylene blue (MB) in the dark in a slurry reactor containing 80 mg of catalyst and 80 mL of MB solution. At every 10 min interval, 4 mL of the suspension was periodically withdrawn and analyzed after centrifugation. The concentration of MB solutions was analyzed by recording the optical absorption intensity at 660 nm using a UV3900 UV-Vis spectrophotometer.
2.4. Measurements
Powder XRD patterns were collected with a PANalytical X'Pert Pro X-ray diffractometer using Cu Kα radiation (1.54 Å), and the working voltage was 40 kV. The morphologies and microstructures of the products were examined by a field emission scanning electron microscope (FSEM, Zeiss Ultra 55) equipped with an EDX and transmission electron microscope (TEM, Zeiss Libra 200 FE). The surface compositions of the samples were obtained by X-ray photoelectron spectroscopy (XPS, XSAM-800), using Al Kα radiation (hν = 1486.6 eV, Kratos). The optical spectra of all the samples were obtained using an ultraviolet visible (UV-Vis) spectrometer (UV3900, Hitachi Corporation, Japan). The products were acquired on a gas chromatograph-mass spectrometer (GC-MS, Varian 3900, GC-Saturn 2100T).
3. Results and discussion
3.1. Structural monitoring of thermocatalyst
3.1.1. Morphological observation. FESEM was used to investigate the morphology and particle size of as-prepared samples. Fig. 1 shows the extraordinary size, shape, morphology and organization of BMB. As shown in Fig. 1a and b, BMB is a spherical particle with a diameter of 1 μm. It can be seen that the microspheres are fabricated by hundreds of small and elongated strips with a length of about 100 nm. In brief, BMBs are composed of sphere-like BiOCl tightly aggregating Bi2MoO6 outside, which indicate strongly an intimate contact between the two phases above. Fig. 1c displays that BMB are micro-spheres, which reveal that the particle surface is covered uniformly with small portions of an amorphous phase of Bi2MoO6. The dispersing diffraction peaks in Fig. 1d is the presentation of BiOCl. The SAED pattern unravels that BiOCl is polycrystal without any doubt. Further, some tiny crystallites with lattice spacing (0.27 nm) corresponding to the (110) plane of BiOCl33 are clearly observed in BMB particles (Fig. 1d). On the basis of the experimental results, we believe that the tiny crystallites are the original source of those trace Bragg peaks surviving in the XRD pattern, demonstrating that the particles belong to BiOCl covered with amorphous Bi2MoO6 once again.
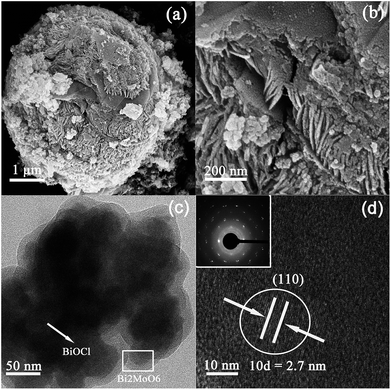 |
| Fig. 1 FESEM images of BMB (a and b), TEM image of BMB (c) and HRTEM image of BiOCl (inset: SAED pattern) (d). | |
3.1.2. Crystalline structure. The composition and crystallographic structure of BMB were determined by XRD. As shown in Fig. 2, all of the diffraction peaks of BiOCl were well matched with the tetragonal phase of the BiOCl phase (JCPDS card no. 06-0249, lattice constants a = b = 3.891 Å and c = 7.369 Å). The four intense diffraction peaks at 2θ = 25.8°, 32.5°, 33.3°, 46.6° and three minor peaks at 2θ = 23.9°, 40.8°, 49.6°, corresponding to the (101), (110), (102) and (200) along with the (002), (112) and (113) crystal planes, respectively. It should be noted that some trace Bragg peaks still survived in the pattern of BMB, while the positions of the peaks imply the presence of a Bi2MoO6 phase. Fig. 2 shows that the reflection indexed to Bi2MoO6 (JCPDS card no. 22-0113), but only as a broad hump (28–61°), was observed for the as-prepared BMB. The XRD results suggest that Bi2MoO6 is amorphous and some tiny crystallites in nanoscale may exist in the amorphous form. XRD patterns of BMB show a combination of BiOCl and Bi2MoO6, indicating the successful synthesis of BMB.
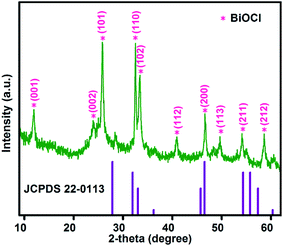 |
| Fig. 2 XRD patterns of BMB. | |
3.1.3. Chemical composition. In order to reveal the essential composition of the BMB composite, the chemical states of as-synthesized samples were carefully checked by XPS. As depicted in Fig. 3a, this clearly indicates that the product is mainly composed of elements such as Bi, O, Cl and Mo. The Bi 4f, O 1s, Cl 2p, and Mo 3d are shown in Fig. 3 (panels b, c, d and e, respectively). The high-resolution XPS spectrum of the Bi 4f region is seen in Fig. 3b and the peaks centered at 158.4 and 163.6 eV can be assigned to Bi 4f7/2 and Bi 4f5/2, respectively, indicating that the Bi is in the form of Bi3+. It is worth pointing out that the Bi 4f5/2 and Bi 4f7/2 binding energies of BMB are not exactly the same as those of BiOCl or Bi2MoO6 (159.3 and 164.6 eV for BiOCl, 164.5 and 159.50 eV for pure Bi2MoO6). These results indicate the formation of an interfacial structure between BiOCl and Bi2MoO6, which leads to a local environment and electron density of Bi that is different from those of BiOCl or Bi2MoO6. The O 1s peak is displayed in Fig. 3c, which is fitted to the peak centering at 532.2 eV and mainly assigned to the oxygen in the prepared sample lattice. In Fig. 3d, the Cl 2p spectrum is resolved into two peaks at 198.5 and 200.1 eV, which are assigned to Cl 2p3/2 and Cl 2p1/2 for BiOCl, respectively.34 Additionally, Fig. 3e shows the binding energies of Mo 3d3/2 and Mo 3d5/2 in the Bi2MoO6, which are located at 235.6 and 232.6 eV, respectively, suggesting that a six-valent oxidation state for Mo6+ exists in the Bi2MoO6. The peaks corresponding to Mo 3d in the XPS spectra (Fig. 3e) of the hierarchical BMB composites lightly shift (about 0.2 eV) toward a higher binding energy compared to pure Bi2MoO6 due to the strong interaction with BiOCl. This evidence matches up with the XRD data as well, further proving the coexistence of Bi2MoO6 and BiOCl.
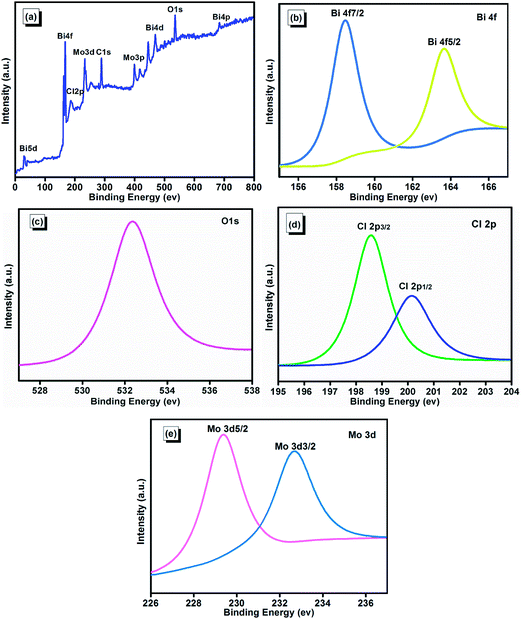 |
| Fig. 3 XPS spectra of BMB. (a) Full XPS spectrum; (b) Bi 4f; (c) O 1s; (d) Cl 2p; (e) Mo 3d. | |
3.2. Thermally-responsive catalytic degradation property
Before the degradation reactions, the dark adsorption tests were conducted for an adsorption–desorption equilibrium, which was strongly magnetically stirred for 30 min in the dark. Fig. 4 shows the adsorption capacity of BMB to MB in different MB concentrations. It is found that the adsorption capacity generally increases with the increasing concentration, and an adsorption content of around 10% concerning MB is adsorbed onto the BMB in a concentration of 80 mg L−1 at most. This adsorption capability of BMB to the dye might be attributed to the BMB sphere, owing to the fine micro-structures. In other words, a moderate physical adsorption ability would be beneficial to drive the subsequent degradation effect.
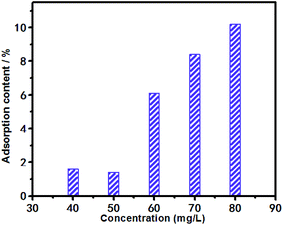 |
| Fig. 4 The adsorption capacity of BMB for MB in different substance concentrations. | |
3.2.1. Effect of concentration and temperature. At first, it is worthwhile to state that all the degradation tests were carried out in a dark environment throughout the trial, including the dark adsorption measurements. The evidence obtained shows that the separate catalysts (BiOCl or Bi2MoO6) hardly has a degradation of performance in the dark (Table 1). The degradation property is not reflected until BMB is add to the reaction system as seen in Fig. 5, strongly signifying the success of the thermally-responsive catalytic approach over BMB. Therefore, the significant drop in the detected absorbance could be attributed to the decomposition of water pollutants by a thermally-responsive catalytic action.
Table 1 The changes of C/C0 of MB throughout the degradation process via separate catalysts (BiOCl or Bi2MoO6)
Catalyst |
C/C0 |
10 min |
20 min |
30 min |
40 min |
50 min |
60 min |
BiOCl |
99.5 |
99.0 |
98.7 |
98.1 |
97.8 |
96.9 |
Bi2MoO6 |
99.7 |
99.3 |
98.8 |
98.3 |
97.9 |
97.5 |
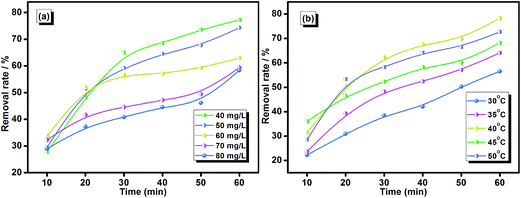 |
| Fig. 5 Thermally-responsive catalytic degradation of MB using BMB. (a) Different concentrations at 25 °C; (b) different temperatures at 40 mg L−1. | |
Thermally-responsive catalytic performances of BMB were investigated through degradation tests of MB. Fig. 5a shows the results for the degradation of MB solutions in different concentrations such as 40, 50, 60, 70 and 80 mg L−1. It can be clearly seen that the removal rate reaches as high as 77.3, 74.3, 62.3, 59.4 and 58.3% after 60 min only in a dark environment, respectively, wherein the best degradation efficiency is gained at a concentration of 40 mg L−1. The above phenomena may be attributed to the appropriate active sites on the BMB surface. Too high a concentration of MB solution would possibly compete with the thermocatalyst for the limited sites, and at the same time too low a concentration of MB would not provide enough sites with the degradation reactions. The rate difference seems to be owed to the increase in total surface area, and many more active sites are formed, which are available to the subsequent degradation reactions. Namely, there still exist many vacant active sites for thermally-responsive catalytic reactions. From Fig. 5b, the trends of various curves all show a dramatic increase with the degradation treatment continuing. The removal rate reaches up to 56.5, 64.1, 78.3, 68.2 and 72.7, at temperatures of 30, 35, 40, 45 and 50 °C, respectively, and the case of 40 °C presents the optimal efficiency compared with the other cases. The degradation effect is strengthened gradually from a general view. Because a plenty of active species could be produced upon the temperature excitation, which join in the degradation reactions, resulting in the improvement of thermally-responsive catalytic activity. However, a decrease of degradation rate was also monitored under the higher temperature condition in Fig. 5b, owing to an intensive collision between molecules in adsorbing MB. Specifically, MB attached to active sites is not fixed itself in reacting with BMB, partly because heat is transferred to MB rather than BMB, not exerting a thermal responsive. Additionally, the intermediates formed from degradation reactions may compete with MB for the restricted active sites in the higher temperature environment, partly losing the degradation capacity.
3.2.2. Effect of pH. As is well known, the solution pH plays an important role in the degradation of organic pollutants.35 As shown in Fig. 6, after undergoing 60 min, degradation rates of 61.9, 66.9, 74.9, 84.3, 84.5 and 90.6% MB occur at pH 2.5, 3.4, 5.7, 7.1, 9.0 and 10.0, respectively. The results show that an alkaline atmosphere is better than an acid one in terms of the degradation efficiency of MB. The reason for this is that pH variation could improve the surface charge case of the catalysts.36 Particularly, the positive charges of the cation make the adsorption process occur more readily at the negative sites on the catalyst that exists in the alkaline solution. On the contrary, the acid solution would naturally lead to less adsorption content, which is disadvantageous to the degradation of MB.
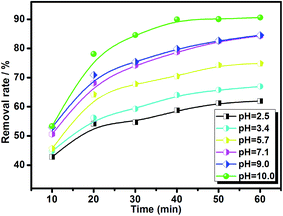 |
| Fig. 6 Thermally-responsive catalytic degradation efficiency of MB (40 mg L−1, 40 °C) by BMB under different pH. | |
3.2.3. Effect of different ions. Generally speaking, there are a few different ions in the actual wastewater; as a result we investigated the effect of some common anions on the thermally-responsive catalytic degradation of MB via BMB in a heterogeneous system, as shown in Fig. 7. The degradation rate of MB increases evidently under the existence of these ions and the optimal removal rate reaches up to as high as 99.9%. The affecting extent follows the sequence: PO43− > CO32− > Cl− > SO42− > NO3− > control. This consequence may be ascribed to the reduction of the recombination of electrons and holes over thermal excitation. For example, the presence of Cl− could obviously accelerate the thermally-responsive catalytic rate owing to the occurrence of the reaction: h+ + Cl− → Cl˙, which could reduce the recombination and prolong the lifetime of thermal-generated electrons. The function of these ions in the MB degradation course is concentrated upon the acceleration of quenching of hydroxyl radicals. We notice that the anions with a high adsorption ability, such as PO43− (the specifically-adsorbed anions can make the surface negatively charged37), have a greater impact on the thermally-responsive catalytic process in comparison with the less or non-specifically-adsorbed ones (SO42− and NO3−).
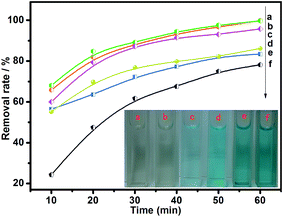 |
| Fig. 7 Influence of different ions on thermally-responsive catalytic degradation of MB in solutions containing 80 mL MB solution and 80 mg BMB at 40 °C: (a) 0.1 mol L−1 PO43−; (b) 0.1 mol L−1 CO32−; (c) 0.1 mol L−1 Cl−; (d) 0.1 mol L−1 SO42−; (e) 0.1 mol L−1 NO3−; (f) the blank without any ions. (Inset: photographs of the dye solutions throughout the process). | |
3.2.4. Catalytic stability evaluation. To further demonstrate the recycling property of BMB, three-cycle tests were executed using the cycled BMB. Apparently, the degradation rate increases steadily with increasing time (Fig. 8) after the first use of BMB, which is up to >90%. The degradation activity for removing MB does not much decrease after the second use as shown in Fig. 8. Even if three cycling processes are employed, the degradation efficiency remains at a level of >70%, meaning that BMB could be reused for repeated cycles without a large loss of activity. Besides, it is necessary to mention that the drying course was not unavoidably implemented throughout the three cycles. In summary, this BMB catalyst may exhibit an appreciable suitability for practical industrial applications in the disposal for water pollutants in consideration of the evidence of its property stability and moderate recycling usage.
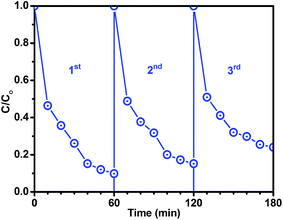 |
| Fig. 8 Repetition assessment of BMB during experiencing three cycling uses. | |
3.3. Identification of degradation products
Potential products after undergoing an MB degradation process were measured using GC-MS and statistical analysis. The MB (30 mg) was placed in a 50 mL glass vial, tightly capped with polytetrafluoroethylene bottle caps, and then the degradation experiment was performed for 40 min at 40 °C in the dark to allow for the equilibration of the volatiles in the headspace. After the equilibration time, the bottle caps were pierced with a SPME needle and the fiber was exposed to the headspace for 30 min. Fig. 9 shows a representative GC-MS chromatogram of already degraded MB, which contain seven major products. Many intermediates could be formed as ˙OH is unable to exhibit a high degree of selectivity towards various functional groups, resulting in multiple oxido-reductive pathways. All of the products present smaller molecular weights compared with MB, and some small characteristic mass losses such as the Rt at 2.50, 8.20 and 14.28 min are observed from the MS fragments, suggesting oxygen insertion into the structure, and eventually leading to the formation of carboxyl, carbonyl, and alcohol groups. The SPME method is very sensitive and the degradation by-products of MB are very complex, and so merely the main products were analyzed in this work. Seven main compounds detected in the solution are presented in Table 2, which are methanedithione, toluene, 4-methylpent-4-en-2-ol, o-xylene, 3-methylhexanal, (E)-2,6-dimethylhept-3-ene and hex-1-en-3-ol, respectively. Finally, it is worthwhile noting that most of the accurate mass measurements are monitored with an error of less than 2 ppm, thus providing a high degree of certainty in the assignment of formulas.
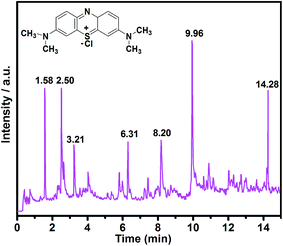 |
| Fig. 9 A representative chromatogram of oxidized samples obtained during 15 min by a solid-phase microextraction and gas chromatography-mass spectrometry (SPME-GCMS) method. | |
Table 2 GC-MS information on the major products after experiencing degradation reactions
Products |
Rt (min) |
Calculated mass |
Structures |
Molecular formula |
Methanedithione |
1.58 |
76 |
S C S |
CS2 |
Toluene |
3.21 |
92 |
 |
C7H8 |
4-Methylpent-4-en-2-ol |
2.50 |
100 |
 |
C6H12O |
o-Xylene |
6.31 |
106 |
 |
C8H10 |
3-Methylhexanal |
8.20 |
114 |
 |
C7H14O |
(E)-2,6-Dimethylhept-3-ene |
9.96 |
126 |
 |
C9H18 |
Hex-1-en-3-ol |
14.28 |
100 |
 |
C6H12O |
3.4. Possible thermally-responsive catalytic mechanism
Upon the thermal activation, two semiconductors (BMB) with overlapping band potentials are considered to be suitable components for constructing an effective heterojunction, which could drive an efficient separation of thermoexcited electrons and holes and then promote the thermally-responsive catalytic activation.38,39 To better understand the formation of BMB, the initial energy band structures of Bi2MoO6 and BiOCl are provided in Fig. 10. Meanwhile, the band positions of Bi2MoO6 and BiOCl are also calculated (Fig. 10) by the following empirical formulas (eqn (1) and (2)):where EVB is the valence band edge potential, ECB is the conduction band edge potential, Ee is the energy of free electrons on the hydrogen scale (Ee = 4.5 eV), and Eg is the band gap energy of the semiconductor. X is the electronegativity of the semiconductor, which is the geometric mean of the electronegativity of the constituent atoms. The X values for BiOCl and Bi2MoO6 are 6.36 and 6.31 eV, respectively. Particularly, the conduction band level (ECB) of BiOCl is 0.26 eV, which is more positive than that of Bi2MoO6 (ECB = −0.32 eV) and the valence band (EVB) energy level of BiOCl (3.55 eV) is higher than that of Bi2MoO6 (2.6 eV).40,41 Besides, the CB energy levels of BiOCl and Bi2MoO6 are mainly dominated by unoccupied Bi 6p states, and the identical energy level distribution of BMB exhibits high delocalized electron states. Due to the special band structure of the BMB composite, the thermogenerated electrons on Bi2MoO6 could inject more freely into the CB of BiOCl and subsequently be trapped by O2 to produce O2−. For this reason, a large number of holes from the VB of Bi2MoO6 would react with OH− to produce ˙OH, making the electrons and holes separate.
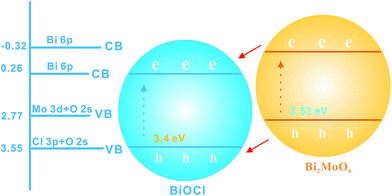 |
| Fig. 10 The schematic diagram of the band structures for the BMB composite. | |
4. Conclusions
A one-step hydrothermal approach was used to synthesize Bi2MoO6/BiOCl (BMB) under low-temperature conditions. The influence of initial concentration, reaction temperature, solution pH, and common ions on the thermally-responsive catalytic degradation property of methylene blue (MB) was studied in detail using XRD, FESEM, TEM, and XPS. The results obtained show that BMB exists in a spherical form and is covered with an amorphous Bi2MoO6 layer. An outstanding thermally-responsive catalytic activity on decomposing MB was measured in the presence of BMB in the dark at a relative low temperature, ultimately suggesting the optimal conditions (40 °C, 40 mg L−1, and pH at 11). Intriguingly, it is found that the addition of PO43−, CO32−, Cl−, SO42− and NO3− anions in MB solutions could harvest a better subsequent degradation effect. In addition, the degradation products from thermally-responsive catalytic MB reactions were analyzed by GC-MS, showing that the thermally-responsive catalytic pattern has the capacity to execute the destruction of organic dyes. Recycling tests reveal that the total degradation efficiency of MB using BMB still maintains a level of >70% even after three degradation cycles, so BMB may be recovered and reused without appreciable loss in the catalytic activity. A likely mechanism for the destruction of pollutants using BMB is also discussed. Thus, this BMB responsive to heat excitation may be very useful for the highly efficient degradation of water pollutants in future environmental remediation.
Conflict of interest
The authors declare no competing financial interest.
Acknowledgements
The authors acknowledge the financial support from the Key Program of National Nuclear Facility Decommissioning and Radioactive Waste Treatment (Grant no. 2014ZG6101) and Hunan Provincial Innovation Foundation For Postgraduate (Grant no. CX2016B002).
References
- D. P. Mohapatra, S. K. Brar, R. D. Tyagi, P. Picard and R. Y. Surampalli, Analysis and advanced oxidation treatment of a persistent pharmaceutical compound in wastewater and wastewater sludge-carbamazepine, Sci. Total Environ., 2014, 470, 58–75 CrossRef PubMed.
- J. L. Liu and M. H. Wong, Pharmaceuticals and personal care products (PPCPs): a review on environmental contamination in China, Environ. Int., 2013, 59, 208–224 CrossRef CAS PubMed.
- J. Levec and A. Pintar, Catalytic wet-air oxidation processes: a review, Catal. Today, 2007, 124(3), 172–184 CrossRef CAS.
- X. Zhuang, Y. Wan and C. Feng, Highly efficient adsorption of bulky dye molecules in wastewater on ordered mesoporous carbons, Chem. Mater., 2009, 21, 706–716 CrossRef CAS.
- C. C. Wang, J. R. Li, X. L. Lv, Y. Q. Zhang and G. S. Guo, Photocatalytic organic pollutants degradation in metal–organic frameworks, Energy Environ. Sci., 2014, 7, 28–31 Search PubMed.
- G. M. S. ElShafei, F. Z. Yehia and O. I. H. Dimitry, Degradation of nitrobenzene at near neutral pH using Fe2+–glutamate complex as a homogeneous Fenton catalyst, Appl. Catal., B, 2010, 99, 242–247 CrossRef CAS.
- K. Pazdzior, A. Klepacz-Smółka, S. Ledakowicz, J. Sójka-Ledakowicz, Z. Mrozinska and R. Zyłła, Chemosphere, 2009, 75, 250–255 CrossRef CAS PubMed.
- M. J. Muñoz-Batista, A. Kubacka, R. Rachwalik, B. Bachiller-Baeza and M. Fernández-García, Green photo-oxidation of styrene over W–Ti composite catalysts, J. Catal., 2014, 309, 428–438 CrossRef.
- M. Niu, F. Huang, L. Cui, P. Huang, Y. Yu and Y. Wang, Hydrothermal synthesis, structural characteristics, and enhanced photocatalysis of SnO2/α-Fe2O3 semiconductor nanoheterostructures, ACS Nano, 2010, 4, 681–688 CrossRef CAS PubMed.
- A. Rey, J. Carbajo, C. Adán, M. Faraldos, A. Bahamonde, J. A. Casas and J. J. Rodriguez, Improved mineralization by combined advanced oxidation processes, Chem. Eng. J., 2011, 174, 134–142 CrossRef CAS.
- A. Dolbecq, P. Mialane, B. Keita and L. Nadjo, Polyoxometalate-Based Materials for Efficient Solar and Visible Light Harvesting: Application to the Photocatalytic Degradation of Azo Dyes, J. Mater. Chem., 2012, 22, 24509–32452 RSC.
- H. Tong, S. X. Ouyang, Y. P. Bi, N. Umezawa, M. Oshikiri and J. H. Ye, Nano-photocatalytic materials: possibilities and challenges, Adv. Mater., 2012, 24, 229 CrossRef CAS PubMed.
- Z. F. Zhao, J. Xu, P. K. Liaw, B. Wu and Y. Wang, One-step formation and photocatalytic performance of spindle-like TiO2 nanorods synthesized by dealloying amorphous Cu50Ti50 alloy, Corros. Sci., 2014, 84, 66–73 CrossRef CAS.
- X. G. Luo, S. Z. Zhang, F. Ding and X. Y. Lin, Low temperature catalytic oxidative aging of LDPE films in response to heat excitation, Phys. Chem. Chem. Phys., 2015, 17, 22272–22285 RSC.
- C. Chen, W. Ma and J. Zhao, Semiconductor-mediated photodegradation of pollutants under visible-light irradiation, Chem. Soc. Rev., 2010, 39, 4206–4219 RSC.
- T. Y. Wang, F. J. Zhang, G. S. Xiao, S. Zhong and C. Lu, Synthesis of Bi2WO6/Bi2O3 Composite with Enhanced Photocatalytic Activity by a Facile One-step Hydrothermal Synthesis Route, Photochem. Photobiol., 2014, 20, 1–7 CrossRef CAS.
- X. M. Mao, C. M. Fan, X. C. Zhang, Y. W. Wang, Y. F. Wang and G. Y. Ding, Effect of chlorine ion on the crystalline and photocatalytic activity of BiOCl for the degradation of rhodamine B, Cryst. Res. Technol., 2013, 4, 96–504 Search PubMed.
- A. Biswas, R. Das, C. Dey, R. Banerjee and P. Poddar, Ligand-Free One-Step Synthesis of {001} Faceted Semiconducting BiOCl Single Crystals and Their Photocatalytic Activity, Cryst. Growth Des., 2014, 14, 236–239 Search PubMed.
- J. Jiang, K. Zhao, X. Y. Xiao and L. Z. Zhang, Synthesis and Facet-Dependent Photoreactivity of BiOCl Single-Crystalline Nanosheets, J. Am. Chem. Soc., 2012, 134, 4473–4476 CrossRef CAS PubMed.
- Z. Q. He, Y. Q. Shi, C. Gao, L. N. Wen, J. M. Chen and S. Song, BiOCl/BiVO4 p–n Heterojunction with Enhanced Photocatalytic Activity under Visible-Light Irradiation, J. Phys. Chem. C, 2014, 118, 389–398 CrossRef CAS.
- S. X. Weng, Z. B. Fang, Z. F. Wang, Z. Y. Zheng, W. H. Feng and P. Liu, Construction of Teethlike Homojunction BiOCl (001) Nanosheets by Selective Etching and Its High Photocatalytic Activity, ACS Appl. Mater. Interfaces, 2014, 6, 18423–18428 Search PubMed.
- Y. Hou, A. B. Laursen, J. Zhang, G. Zhang, Y. Zhu, X. Wang, S. Dahl and I. Chorkendorff, Layered Nanojunctions for Hydrogen-Evolution Catalysis, Angew. Chem., Int. Ed., 2013, 52, 3621–3625 CrossRef CAS PubMed.
- F. Dong, Y. J. Sun, M. Fu, Z. B. Wu and S. C. Lee, Room Temperature Synthesis and Highly Enhanced Visible Light Photo-Catalytic Activity of Porous BiOI/BiOCl Composites Nanoplates Microflowers, J. Hazard. Mater., 2012, 219–220, 26–34 CrossRef CAS PubMed.
- S. J. Hong, S. Lee, J. S. Jang and J. S. Lee, Heterojunction BiVO4/WO3 Electrodes for Enhanced Photoactivity of Water Oxidation, Energy Environ. Sci., 2011, 4, 1781–1787 Search PubMed.
- N. Liang, J. Zai and M. Xu, Novel Bi2S3/Bi2O2CO3 heterojunction photocatalysts with enhanced visible light responsive activity and wastewater treatment, J. Mater. Chem. A, 2014, 2, 4208–4216 RSC.
- J. Hu, W. Fan and W. Ye, Insights into the photosensitivity activity of BiOCl under visible light irradiation, Appl. Catal., B, 2014, 158, 182–189 CrossRef.
- Y. J. Chen, G. H. Tian, Y. H. Shi, Y. T. Xiao and H. G. Fu, Hierarchical MoS2/Bi2MoO6 composites with synergistic effect for enhanced visible photocatalytic activity, Appl. Catal., B, 2015, 164, 40–47 CrossRef CAS.
- S. Z. Zhang, X. G. Luo, F. Ding and X. Y. Lin, Thermocatalytic degradation of low density polyethylene films by responding to the actuation of heat, RSC Adv., 2014, 4, 41744–41752 RSC.
- S. Z. Zhang, X. G. Luo and X. Y. Lin, New insights on degradation of methylene blue using thermally-responsive reactions catalyzed by low-temperature excitation, J. Hazard. Mater., 2013, 260, 112–121 CrossRef PubMed.
- Y. Zuo, C. Wang, Y. Sun and J. Cheng, Preparation and photocatalytic properties of BiOCl/Bi2MoO6 composite photocatalyst, Mater. Lett., 2015, 139, 149–152 CrossRef CAS.
- W. J. Yang, B. Ma, W. C. Wang, Y. W. Wen, D. W. Zeng and B. Shan, Enhanced photosensitized activity of a BiOCl–Bi2WO6 heterojunction by effective interfacial charge transfer, Phys. Chem. Chem. Phys., 2013, 15, 19387–19394 RSC.
- J. Cheng, C. Wang, Y. Cui, Y. Sun, Y. Zuo and T. Wang, Large improvement of visible-light-driven photocatalytic property in AgCl nanoparticles modified black BiOCl microsphere, Mater. Lett., 2014, 127, 28–31 CrossRef CAS.
- J. L. Hu, W. J. Fan, W. Q. Ye, C. J. Huang and X. Q. Qiu, Insights into the photosensitivity activity of BiOCl under visible light irradiation, Appl. Catal., B, 2014, 158–159, 182–189 CrossRef CAS.
- Y. Yu, C. Cao and H. Liu, A Bi/BiOCl heterojunction photocatalyst with enhanced electron–hole separation and excellent visible light photodegrading activity, J. Mater. Chem. A, 2014, 2, 1677–1681 RSC.
- S. Balachandran and M. Swaminathan, The simple, template free synthesis of a Bi2S3–ZnO heterostructure and its superior photocatalytic activity under UV light, Dalton Trans., 2013, 42, 5338–5347 RSC.
- M. T. Uddin, Y. Nicolas, C. Olivier, T. Toupance, M. M. Müller, H.-J. Kleebe, K. Rachut, J. Ziegler, A. Klein and W. Jaegermann, Preparation of RuO2/TiO2 mesoporous heterostructures and rationalization of their enhanced photocatalytic properties by band alignment investigations, J. Phys. Chem. C, 2013, 117, 22098–22110 CrossRef CAS.
- H. Sheng, Q. Li, W. H. Ma, H. W. Ji, C. H. Chen and J. C. Zhao, Photocatalytic degradation of organic pollutants on surface anionized TiO2: common effect of anions for high hole-availability by water, Appl. Catal., B, 2013, 138–139, 212–218 CrossRef CAS.
- X. G. Luo, S. Z. Zhang, F. Ding and X. Y. Lin, Implementing thermally-excited-catalytic course solely using ambient heat motivation for efficient abatement of water pollutants, RSC Adv., 2016, 6, 18040–18051 RSC.
- S. Z. Zhang, F. Ding, X. G. Luo and X. Y. Lin, Facile synthesis of hierarchical Mo-doped S/BiOCl heterostructured spheres and its excellent photo/thermocatalytic activity under near room temperature, J. Alloys Compd., 2016, 673, 93–101 CrossRef CAS.
- H. W. Huang, L. Y. Liu, Y. H. Zhang and N. Tian, One pot hydrothermal synthesis of a novel BiIO4/Bi2MoO6 heterojunction photocatalyst with enhanced visible-light-drivenphotocatalytic activity for rhodamine B degradation and photocurrentgeneration, J. Alloys Compd., 2015, 619, 80794–81101 Search PubMed.
- X. Lin, D. Liu, X. Y. Guo, N. Sun, S. Zhao, L. M. Chang, H. J. Zhai and Q. W. Wang, Fabrication and efficient visible light-induced photocatalytic activity of Bi2MoO6/BiPO4 composite, J. Phys. Chem. Solids, 2015, 76, 170–177 CrossRef CAS.
Footnote |
† These authors contributed equally to this paper. |
|
This journal is © The Royal Society of Chemistry 2016 |
Click here to see how this site uses Cookies. View our privacy policy here.