DOI:
10.1039/C6RA06450G
(Paper)
RSC Adv., 2016,
6, 47741-47752
Mg2+ ion as a tuner for colorimetric sensing of glyphosate with improved sensitivity via the aggregation of 2-mercapto-5-nitrobenzimidazole capped silver nanoparticles†
Received
10th March 2016
, Accepted 5th May 2016
First published on 9th May 2016
Abstract
Silver nanoparticles based analyte recognition has received a wide range of interest in the assaying of trace target analytes in environmental samples. In this work, a simple colorimetric assay was developed for the rapid and selective detection of glyphosate in water and food samples via the aggregation of 2-mercapto-5-nitrobenzimidazole capped silver nanoparticles (MNBZ-Ag NPs) using Mg2+ ions as a tuner and trigger. Initially, Ag NPs were synthesized with sodium borohydrate as a reducing agent and capped with MNBZ which showed a yellow color. The addition of Mg2+ ions did not cause aggregation of MNBZ-Ag NPs, but the subsequent addition of glyphosate resulted a drastic decrease in interparticle distance through complex formation between MNBZ-Ag NPs–Mg2+ ion and glyphosate, yielding a color change from yellow to orange-red, which results in a red-shift in the characteristic surface plasmon resonance (SPR) peak from 399 to 517 nm. Based on this, sensitive and selective detection of glyphosate was achieved with a limit of detection of 17.1 nM. This probe was successfully applied to detect glyphosate in water and food samples, which proves a very simple and selective platform for on-site monitoring of glyphosate in agriculture samples.
1. Introduction
Glyphosate (N-(phosphonomethyl)glycine) is a broad-spectrum, non-selective, postemergence and nonselective organophosphorus herbicide that widely used for weed and vegetation control in agricultural, industrial, and domestic uses.1 It inhibits the activity of 5-enolpyruvylshikimate-3-phosphate synthase in the shikimic acid pathway, which is essentially needed in the biosynthesis of aromatic acids precursors of many proteins.2 It shows high herbicidal activity and exhibits relatively low toxicity to biological systems. Due to its indiscriminate use in the agriculture sector, its residue accumulation in both soil through adsorption, and water, leading to create possible health hazard and environmental contamination. As a result, the US Environmental Protection Agency (EPA)3 and the European Union4 have set maximum residual level contaminant level (MRL) of glyphosate in water at 0.7 μg mL−1 (4.14 μM) and in most crops at 0.1 μg g−1. Similarly, China also fixed the MRL of glyphosate in fruits at 0.5 mg kg−1.5 Moreover, the World Health Organization has set the maximum permissible concentration of glyphosate in drinking water is 0.9 μg mL−1.6 Therefore, the development of glyphosate probe has recently attracted intense attention due to the global security concern on the water and food products.
In this connection, many efforts have been made to develop efficient analytical methods to detect glyphosate in water and food samples. For instance, high-performance liquid chromatography (HPLC),7,8 capillary electrophoresis (CE),9 gas chromatography-mass spectrometry (GC/MS),10,11 HPLC-MS,12 coulometry13 and voltammetry14 have been used for the detection of glyphosate in water and food samples. Even though these methods have effectively detected glyphosate in various samples unfortunately some of the techniques is often required derivatization or addition of chromophores or fluorophores. Some methods do not require the derivatization step, but these methods suffer from specificity and sensitivity. Apart from these, there are still some limitations with existing approaches such as the dependence on expensive equipment and requirement of long-time for samples pretreatment, limiting the application of these methods for the rapid detection of glyphosate in water and food samples.
Recent years, NPs-based fluorescent and colorimetric probes are becoming more and more important for their integration with various analytical techniques for the quantification of various chemical and biological species with high sensitivity.15–18 Recently, considerable efforts have been devoted to design the nanomaterials-based fluorescent and colorimetric probes for sensing of glyphosate in water and food samples with high selectivity and sensitivity. For instance, Ding and Yang developed Au NPs-based SPR biosensor for the rapid detection of glyphosate with good selectivity and sensitivity.19 Guo et al. functionalized thioglycolic acid on the surfaces of CdTe QDs and used as a fluorescent probe for the detection of glyphosate in apple samples.20 Lee and co-workers have reported a sandwich-type immunosensor for the detection of glyphosate using antigen-double target/probe DNA-coated Au NPs as a probe.21 The same group described the use of fluorescence magnetic nanoparticle as a probe for the detection of glyphosate via sandwich format.22 Similarly, Zheng's group functionalized Au NPs with cysteamine and used as a colorimetric probe for the rapid and low-cost detection of glyphosate in water samples.23 Recently, Pletschke's team prepared copper doped poly(vinyl alcohol) (cd-PVP) and used as a nanofiber sensor strips for the detection of glyphosate in water samples.24 Moreover, our group also functionalized Ag NPs with various organic molecules and used as probes for the selective and sensitive detection of mancozeb,25 tricyclazole,26 carbendazim,27 thiram and paraquat28 in environmental and food samples.
In recent years, the incorporation of metal ions into functional nanomaterials has received increasing attention in the development of advanced optical materials for the selective and sensitive detection of various trace level target species from complex samples.29–31 Further, such metal ions integration on the surfaces of functional nanomaterials may lead to an improvement of the selectivity and sensitivity of the nanoprobes, as has been noted previously.32 As a result, metal ions have been incorporated on the surfaces of functional nanomaterials for their interaction with both functional groups of nanomaterials and target analytes, which can facilitate to form supramolecular nano-network systems through coordinate covalent bonding. For example, Zhang's group incorporated Zn2+ ions on the surfaces of dipicolylamine-capped Au NPs for the colorimetric detection of bis-phosphorylated peptides.33 Yu and co-workers developed simple and rapid colorimetric method for the highly selective and sensitive detection of Hg2+ ion using polythymine oligonucleotide T33-citrate-capped Au NPs in the presence of Mn2+ ion as stabilizer.34 Pezzato's group described the role of Zn2+ ion for the self-sorting of phosphate and carboxylate-containing molecules on the surface of monolayer-protected Au NPs.35 Hainfeld et al., synthesized Ni2+–nitrilotriacetic acid–Au clusters and used as a probe for the specific targeting of 6-His region of tagged proteins.36 Elmes's group illustrated the use of Ru(II)-polypyridyl stabilized luminescent Au NPs as probes for imaging of HeLa cells.37 Similarly, Massue and co-workers functionalized Au NPs with heptadendate macrocyclic Eu(III) cyclen conjugate possessing an alkyl thiol group for sensing of phosphate anions.38 These studies motivated us to speculate that when metal ion is incorporated on the functional metallic NPs that bind specifically to the phosphate group of organophosphorus pesticides, resulting the aggregation of metal ion-functionalized NPs induced by target analytes via coordination covalent bonding between metal ion and functional groups of metallic NPs and phosphate group molecules where metal ion can act as linker.
With this in mind, and with the knowledge, in this work we have improved the sensitivity of MNBZ-Ag NPs by adding Mg2+ ion as trigger that can capture the glyphosate at nanomolar concentration with high selectivity. The MNBZ molecules are covalently attached on the surfaces of Ag NPs via Ag–S bond, allowing the Ag NPs with uniform size distribution. However, Mg2+ ion tuners and triggers the aggregation of MNBZ-Ag NPs induced by glyphosate through complex formation (Scheme 1), resulting a red-shift in their SPR peak and a color change from yellow to orange-red, which is rare observation in colorimetric assays using Ag NPs as a probe. This approach was successfully applied to detect glyphosate in water and food samples.
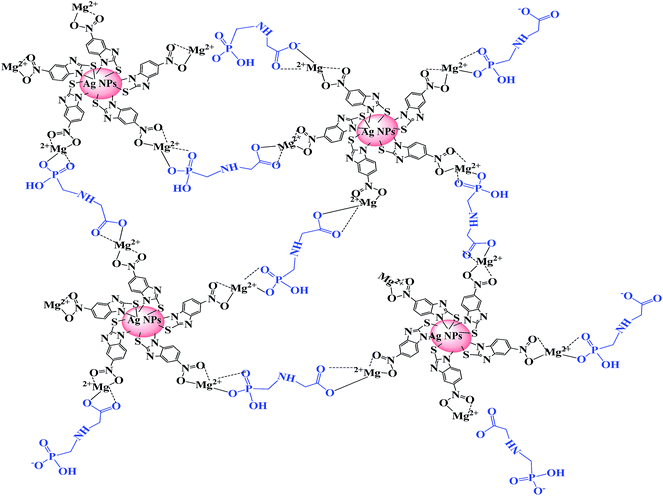 |
| Scheme 1 Schematic representation for the colorimetric detection of glyphosate based on the aggregation of MNBZ-Ag NPs induced by glyphosate using Mg2+ ion as a tuner. | |
2. Materials and methods
2.1. Reagents
All the reagents used were of analytical grade. Silver nitrate (AgNO3) and sodium borohydride (NaBH4) were purchased from Sigma-Aldrich. 2-Mercapto-5-nitro benzimidazole (MNBZ) was purchased from Alfa Aesar. Metal salts (Cd(NO3)2·4H2O, Co(NO3)2·6H2O, CaCl2·2H2O, Cu(NO3)2·3H2O, Hg(NO3)2·H2O, Mg(NO3)2·6H2O and NiSO4·6H2O) were purchased from Sigma-Aldrich, USA. Acephate, monocrotophos, and dichlorvos were received from Super Crop Safe Limited, India. Glyphosate was received from United Phosphorus Limited, India. Chlorpyrifos, quinalphos and triazophos were received from Gujarat Insecticides Limited, India. Profenofos was received from Atul Limited, India. Milli-Q-purified water was used in entire practical work.
2.2. Preparation of MNBZ-Ag NPs
Silver NPs were prepared and functionalized with MNBZ by the following procedure. Briefly, 4.5 mg of NaBH4 was added into 50 mL of 0.1 mM AgNO3 solution under constant stirring at 400 rpm for 5 min. The color of the solution turned into yellow, which confirmed that the formation of bare Ag NPs. Then, 50 μL of 1.0 mM MNBZ solution was added into 25 mL of Ag NPs and stirred for 2 h at room temperature. The resultant MNBZ-Ag NPs solution was stored at 4 °C and used as a probe for colorimetric sensing of glyphosate. The schematic representation for the preparation of MNBZ-Ag NPs was shown in ESI of Fig. S1.†
2.3. Colorimetric detection of glyphosate
Various concentrations of glyphosate were prepared using serial dilution of the stock solution to test the sensitivity limits of the MNBZ-Ag NPs. Using the stock solution, 0.1–1000 μM of glyphosate were prepared. The colorimetric detection of glyphosate was performed at room temperature. Briefly, 750 μL of the as-prepared MNBZ-Ag NPs was added into 7.5 μL of Mg2+ ion solution. To this, 750 μL of glyphosate with different concentrations were added at phosphate buffered saline (PBS) pH 4, yielding a color change from yellow to orange-red, which indicates that the aggregation of MNBZ-Ag NPs induced by glyphosate. In the experiments of selectivity and practical assay, all samples were tested in a similar way. We investigated the selectivity of our new approach for glyphosate over other pesticides (acephate, glyphosate, monocrotophos, chlorpyrifos, quinalphos, triazophos, dichlorvos and profenofos), anions (Cl−, I−, Br−, NO3−, CH3COO−, SO42−, S2− and Cr2O72−, 1.0 mM) and metal ions (Na+, K+, Cu2+, Zn2+, Cd2+, Fe2+, Mn2+, Mg2+, Co2+, Pb2+, Hg2+, Ni2+, Ca2+, Ba2+, Cr3+, Fe3+ and Al3+, 1.0 mM) under the same conditions.
2.4. Determination of glyphosate in environmental water, corn and potato samples
Water samples were collected from three different sources, tap water (Department of Applied Chemistry, SVNIT, India), river water (Tapi river, Surat, India) and canal water (Agriculture canal, Surat, India). To remove solid particulate from the environmental water samples, samples were filtered through 0.45 μm syringe filter. The resulting samples were spiked with different concentration of glyphosate (500, 750 and 1000 nM) and analyzed by aforesaid procedure.
Potato and corn samples were fortified with different concentrations of glyphosate (500, 750 and 1000 nM) for 24 h at room temperature. Then, glyphosate was extracted with 25 mL of methanol in an ultrasonic bath for 20 min. The extracts were decanted and repeated the same procedure twice. The extracted solution was concentrated to 2.0 mL and filtrated through 0.45 μm syringe filter. The concentration of glyphosate was detected by the aforesaid procedure.
2.5. Instrumentation
UV-Vis spectra were measured with a Maya Pro 2000 spectrophotometer (Ocean Optics, USA) at room temperature. FT-IR were recorded with FT-IR 8400S (Shimadzu, Japan). TEM images were taken by using Tecnai 20 (Philips, Holland). DLS were measured by using Zetasizer Nano ZS90 (Malvern, UK).
3. Results and discussion
3.1. Characterization of MNBZ-Ag NPs
The surface plasmon resonance absorption of bare Ag NPs was measured using a UV-visible spectrophotometer and its maximum absorption was located at 405 nm. At a constant concentration of AgNO3 (0.1 mM) and NaBH4 (4.5 mg), MNBZ-Ag NPs were synthesized under various volumes (10 to 100 μL) of 1.0 mM MNBZ as a capping agent (ESI of Fig. S2†). It was noticed that, as the volume of MNBZ increased from 10 to 30 μL, the characteristic SPR band of Ag NPs was very slightly shifted with less intensity. In addition, at higher volume of MNBZ (75–100 μL), the intensity of Ag NPs absorption band reduced and produced broaded peak at 425 nm. Such changes in the absorption response can be easily justified in terms of the morphology of the particles. Therefore, we selected 50 μL of 1.0 mM MNBZ as an optimum concentration for the functionalization of Ag NPs. The main spectral bands differences between free MNBZ and MNBZ-Ag NPs were studied by FT-IR spectroscopy (ESI of Fig. S3†). It can be noticed that the characteristic mercapto (–SH group) stretching frequency of MNBZ at 2521 cm−1 was completely disappeared in MNBZ-Ag NPs, confirming that the covalent bonding between mercapto group of MNBZ and surfaces of Ag NPs (–S–Ag NPs). The peak at 3738 cm−1 corresponding to –NH stretching disappears in ESI of Fig. S3b† showing its role in formation of MNBZ-Ag NPs. Furthermore, the shifting of –NO2 stretching frequency from 1348 to 1385 cm−1 confirms that the MNBZ grafted on the surfaces of Ag NPs. Although the above data confirms the functionalization of Ag NPs with MNBZ, the morphology and size distribution of MNBZ-Ag NPs were studied by transmission electron microscope (TEM) and dynamic light scattering (DLS). As shown in Fig. 3a, the synthesized MNBZ-Ag NPs with an average size of 9.5 nm were estimated from DLS. TEM micrograph shows that the MNBZ-Ag NPs are nearly monodisperse in nature. The TEM image revealed that the particle size of MNBZ-Ag NPs ∼7.2 nm, with most particle sizes falling in the range of 5.9–8.9 nm (Fig. 4a and b).
3.2. Study of MNBZ-Ag NPs interactions with various pesticides
To evaluate the selectivity of MNBZ-Ag NPs towards various organophosphorus pesticides (acephate, glyphosate, monocrotophos, chlorpyrifos, quinalphos, triazophos, dichlorvos and profenofos, 1.0 mM), the absorption spectra of MNBZ-Ag NPs were measured in the presence of above pesticides without addition of Mg2+ ion. The structures of organophosphorus pesticides were shown in ESI of Fig. S4.† As shown in ESI of Fig. S5,† glyphosate was the only pesticide that caused a SPR absorption band to shift from 405 to 500 nm. This red shift was also observed as a color change from yellow to orange-red. Other pesticides did not influence the absorption spectra, indicating that no aggregation occurred. The nitro group on MNBZ-Ag NPs function as a key unit for the non-covalent interactions with glyphosate. As a result, glyphosate binding between –NO2 group on MNBZ-Ag NPs induced the aggregation of the MNBZ-Ag NPs (Scheme 1). The degree of aggregation of MNBZ-Ag NPs depended on the concentration of glyphosate. As shown in ESI of Fig. S6,† the SPR absorption changes with the addition of different concentrations of glyphosate, resulting a decrease in the absorbance at 405 nm decreased with increasing concentration of glyphosate, which yields to generate a new SPR band at 500 nm. A linear relationship was found when the concentration of glyphosate was between 100 and 1000 μM, indicating that the limit of detection is very high (32.1 μM), which is not falling in the maximum permissible limits suggested by US-EPA and European Union.
3.3. Tuning of linear range with the addition of Mg2+ ion
It is well known that metal ions not only coordinate with functional groups of target analytes but also act as a linker by coordinating both target analytes and functional groups on the surfaces of metal NPs.34–39 These binding sites are highly dependent of the valence and coordination geometry of metal ions.39 In order to tune the linear range and to use it in practical applications as per the environmental protection agencies, we hypothesize that the presence of metal ions may affect the interaction of glyphosate and MNBZ-Ag NPs, resulting in the enhancement of glyphosate-induced aggregation of MNBZ-Ag NPs using metal ion as a linker, which can improve the sensitivity of the probe. At the same time, the addition of metal ions can induce the change in refractive index of the surrounding medium of metal NPs and result in the change in both absorbance intensity and position of the SPR peak.40 Thus, UV-visible spectra of MNBZ-Ag NPs were studied in the presence of various metal ions including monovalent (Li+, Na+ and K+), divalent (Ca2+, Cd2+, Co2+, Cu2+, Hg2+, Ni2+ and Mg2+, 1.0 mM), trivalent (Al3+, As3+, Cr3+ and Fe3+) and tetravalent (Pt4+) metal ions at 50 μM without addition of glyphosate. As shown in ESI of Fig. S7a† and 1a, these metal ions alone did not induce any spectral and the color changes of MNBZ-Ag NPs. Next, we have investigated the effect of various metal ions including monovalent (Li+, Na+ and K+), divalent (Ca2+, Cd2+, Co2+, Cu2+, Hg2+, Ni2+ and Mg2+, 1.0 mM), trivalent (Al3+, As3+, Cr3+ and Fe3+) and tetravalent (Pt4+) metal ions at 50 μM in the presence of glyphosate. As shown in ESI of Fig. S7b† and 1b, the characteristic SPR band of MNBZ-Ag NPs is observed at 399 nm, however the addition of glyphosate with Mg2+ ion caused a red shift of the SPR band of MNBZ-Ag NPs in UV-visible spectrum at longer wavelength (517 nm), yielding a color change from yellow to orange-red, which is indicative of the aggregation of MNBZ-Ag NPs induced by glyphosate using Mg2+ ion as a tuner and trigger. Furthermore, we also investigated the sensing ability of MNBZ-Ag NPs with organophosphorus pesticides using Mg2+ ion as a tuner (Fig. 2a). As compared to other metal ions, the presence of Mg2+ ion is accelerated and enhanced the glyphosate-induced aggregation of MNBZ-Ag NPs, resulting a red-shift from 399 to 517 nm and a color change from yellow to orange red, which indicates that MNBZ-Ag NPs–Mg2+ ion system is selectively only for glyphosate (Fig. 2b). Since the addition of Mg2+ ion either into MNBZ-Ag NPs or glyphosate solutions (without MNBZ-Ag NPs) did not cause any color or spectral changes thereby confirming that Mg2+ ion acts as a trigger for the aggregation of MNBZ-Ag NPs induced by glyphosate. In order to know that is there any changes in the size of MNBZ-Ag NPs with the addition of Mg2+ ion, we studied the DLS of MNBZ-Ag NPs in the presence of Mg2+ ion (50 μM) without addition of glyphosate (Fig. 3b). It can be observed that there is no change in the size of MNBZ-Ag NPs after addition of Mg2+ ion, indicating that Mg2+ ion is not induced the aggregation of MNBZ-Ag NPs (Fig. 3b).
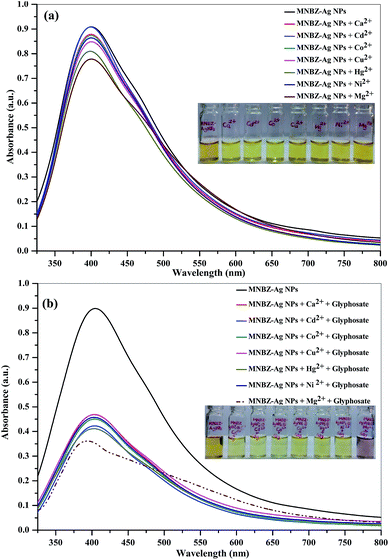 |
| Fig. 1 (a) UV-visible absorption spectra of MNBZ-Ag NPs in the presence of various metal ions (Ca2+, Cd2+, Co2+, Cu2+, Hg2+, Ni2+ and Mg2+, 50 μM) without addition of glyphosate. (b) UV-visible absorption spectra of MNBZ-Ag NPs in the presence of glyphosate (10 μM) using various metal ions (Ca2+, Cd2+, Co2+, Cu2+, Hg2+, Ni2+ and Mg2+, 50 μM) as tuners. Inset is the photographic images illustrating the corresponding colorimetric response. | |
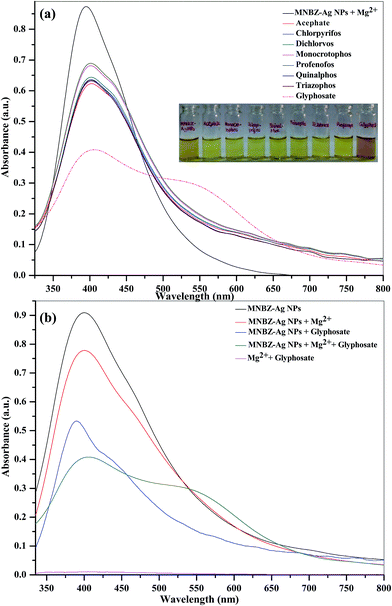 |
| Fig. 2 (a) UV-visible absorption spectra of (a) MNBZ-Ag NPs with Mg2+ ion in presence of different organophosphorus pesticides (acephate, chlorpyriphos, dichlorvos, monocrotophos, profenophos, quinalphos, triazophos, 1.0 mM) and glyphosate (10 μM). Inset is the photographic image illustrating the corresponding colorimetric response. (b) The SPR band spectral changes of MNBZ-Ag NPs with and without glyphosate (10 μM) and Mg2+ ion, glyphosate (without MNBZ-Ag NPs) with Mg2+ ion (50 μM). | |
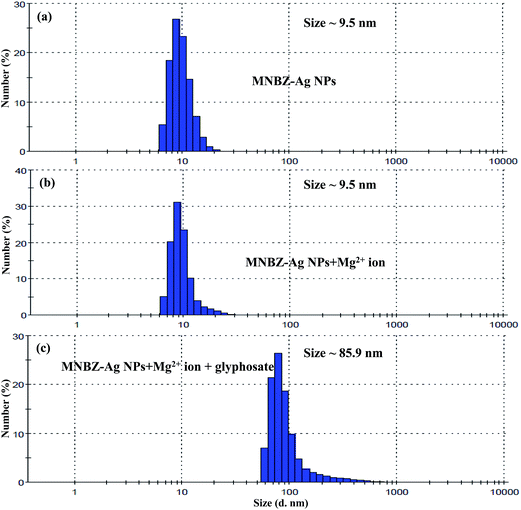 |
| Fig. 3 DLS data of (a) MNBZ-Ag NPs, (b) MNBZ-Ag NPs with Mg2+ and (c) the aggregation of MNBZ-Ag NPs induced by glyphosate using Mg2+ ion as a tuner. | |
3.4. Effect of buffer
To test the effect of the buffer system, we studied the absorption spectra of MNBZ-Ag NPs with glyphosate in the presence of Mg2+ ion at PBS and Tris–HCl buffers pHs from 2.0 to 12.0. To investigate the suitable pH range in which MNBZ-Ag NPs can effectively detect glyphosate using Mg2+ ion as a tuner, the absorption and photographic image of MNBZ-Ag NPs with glyphosate (10 μM) in the presence of Mg2+ ion (50 μM) were studied and shown in Fig. 5. As can be seen in Fig. 5a, the maximum absorption ratio (A517/A399) for the detection of glyphosate was obtained when PBS used as buffer media. It can be noticed that the intensity of the characteristic SPR band of MNBZ-Ag NPs at 399 nm was decreased and broaded at various pHs 2.0–9.0, resulting a change in color from yellow to orange-red. Moreover, at pH 2, the absorption spectrum and color of MNBZ-Ag NPs was also greatly changed due to the self-aggregation of metallic NPs at lower pH (2–3). However, it can be noticed that the characteristic SPR band of MNBZ-Ag NPs at 399 nm was red-shifted to 517 nm, resulting a high absorbance ratio (A517/A399), which induces a change in color from yellow to orange-red. These observations indicate that the pH 4 is selected as the optimal pH condition for analysis of glyphosate by MNBZ-Ag NPs using Mg2+ ion as a tuner.
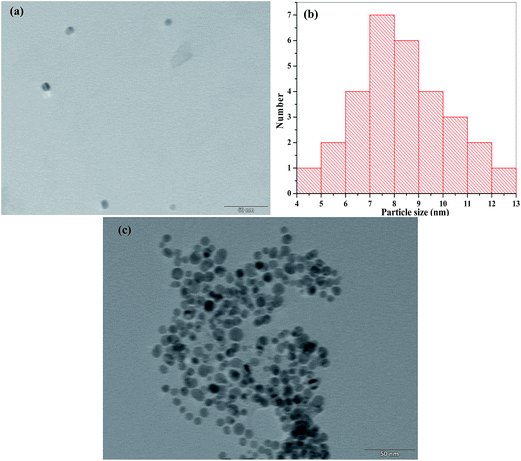 |
| Fig. 4 TEM images of (a) MNBZ-Ag NPs, (b) particle size histogram of MNBZ-Ag NPs and (c) aggregation of MNBZ-Ag NPs induced by glyphosate (1.0 mM). | |
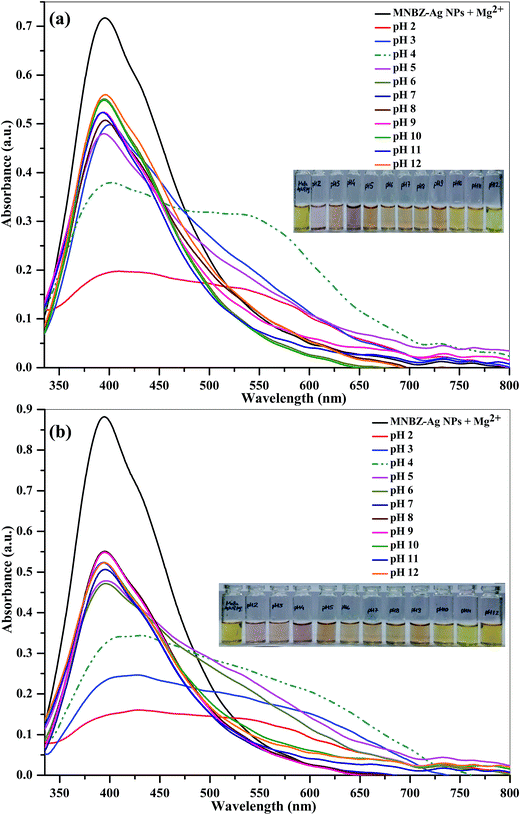 |
| Fig. 5 UV-visible absorption spectra of MNBZ-Ag NPs in the presence of glyphosate (10 μM) using Mg2+ ion (50 μM) as a trigger at (a) PBS and (b) Tris–HCl buffer systems pH in range of 2.0 to 12.0. Inset is the photographic images illustrating the corresponding solutions. | |
3.5. Sensing mechanism
In order to tune dynamic range for the detection of glyphosate using MNBZ-Ag NPs, we studied the various metal ions including (monovalent – Li+, Na+ and K+; divalent – Ca2+, Cd2+, Co2+, Cu2+, Hg2+, Ni2+ and Mg2+; trivalent – Al3+, As3+, Cr3+ and Fe3+; and tetravalent – Pt4+) as tuners for high degree of aggregation of MNBZ-Ag NPs induced by glyphosate. Among these metal ions, Mg2+ ion acts as a tuner for high degree of MNBZ-Ag NPs aggregation induced by glyphosate. Since, magnesium is one of the most abundant metal-ions in biology that can form complexes with nucleotides and the mode of coordination in binary adenosine-5′-diphosphate, adenosine-5′-triphosphate, and in various ternary systems (e.g. using aromatic amines or other biomolecules as additional ligands).41 Furthermore, Mg2+ ion can also be formed complex with nitro substituted salicylic acid at pH 3.4.42,43 It is also confirmed that the significant coordination between Mg2+ ion and phosphate group only takes place above pH 3.5,41 since the phospate and carboxyl group oxygens become deprotonated and donate electrons to the metal ion. With these concepts, we believe that the Mg2+ ion can bind with the nitro group of MNBZ-Ag NPs and phosphate group of glyphosate at PBS pH 4. As a result, the high degree of aggregation of MNBZ-Ag NPs induced glyphosate using Mg2+ ion as a tuner, resulting a red-shift from 399 to 517 nm and a color change from yellow to orange-red, which is rare observation of MNBZ-Ag NPs-based colorimetric assays. To confirm this hypothesis, we studied the UV-visible absorption spectra of MNBZ-Ag NPs in the presence of glyphosate with and without addition of Mg2+ ion. Even though, there is a decrease in the characteristic SPR band of MNBZ-Ag NPs at 399 nm without generation of new SPR peak, indicating that the aggregation of MNBZ-Ag NPs induced by glyphosate via noncovalent interaction that may cause incomplete aggregation. At the same time, it can be noticed that the characteristic SPR band at 399 nm was decreased and generated a new SPR band at 517 with good intensity with addition of Mg2+ ion, indicating that the high degree of MNBZ-Ag NPs aggregation induced by glyphosate via coordinate covalent bonds using Mg2+ ion as a tuner, which confirms that the high degree of MNBZ-Ag NPs aggregation. Therefore, the Mg2+ ion imparts its high affinity for phosphate group of glyphosate and nitro group of MNBZ-Ag NPs through complexation and leads to form supramolecular nanostructures, indicating that the Mg2+ ion acts as a linker to reduce interparticle distance of MNBZ-Ag NPs, which yields both a substantial shift in the plasmon band to longer wavelength and a yellow-to-violet color change (Scheme 1). The as-synthesized MNBZ-Ag NPs with an average size of 9.5 nm were confirmed by DLS and TEM. However, the size and morphology of MNBZ-Ag NPs were greatly changed by the addition of glyphosate, conforming that the aggregation of MNBZ-Ag NPs induced by glyphosate (Fig. 3c and 4c).
3.6. Colorimetric detection of glyphosate
To evaluate the detectable minimum concentration of glyphosate in aqueous solution by color change, we added the different concentrations of glyphosate (0.1–1.2 μM) into MNBZ-Ag NPs solution using Mg2+ ion (50 μM) as a tuner and trigger. The SPR band shift relative to MNBZ-Ag NPs–Mg2+ ion, the absorption ratio at A517/A399 is used to quantify the concentration of glyphosate in this system. Since, the SPR of MNBA-Ag NPs solution at 399 and 517 nm are related to the quantities of dispersed and aggregated MNBZ-Ag NPs in the presence of Mg2+ ion, respectively. Thus, we used the absorption ratio at A517/A399 to express the molar concentration of glyphosate based on the aggregated and dispersed MNBZ-Ag NPs using Mg2+ ion. As shown in Fig. 6a, when the concentration of glyphosate increased from 0.1 to 1.2 μM, the characteristic SPR band at 399 nm was gradually decreased and generated new SPR band at 517 nm, yielding a color change from yellow to orange and then orange-red. As shown in Fig. 6b, the absorption ratio (A517/A399) increases linearly with the increase of the logarithm of glyphosate concentration from 0.1 to 1.2 μM (R2 = 0.991) and reaches a plateau at 1.2 μM. The detection limit was calculated to be 17.1 nM (S/N ratio = 3) and the achieved detection limit is very lower to the maximum contaminant level in drinking water set by United States Environmental Protection Agency (U.S. EPA, 4.14 μM).3,4 It is note to worthy that the MNBZ-Ag NPs–Mg2+ ion-based assay provides lower limit of detection (17.1 nM) for glyphosate detection, which is 1000-times lower LOD than that of MNBZ-Ag NPs system (without Mg2+ ion) (ESI of Fig. S6†).
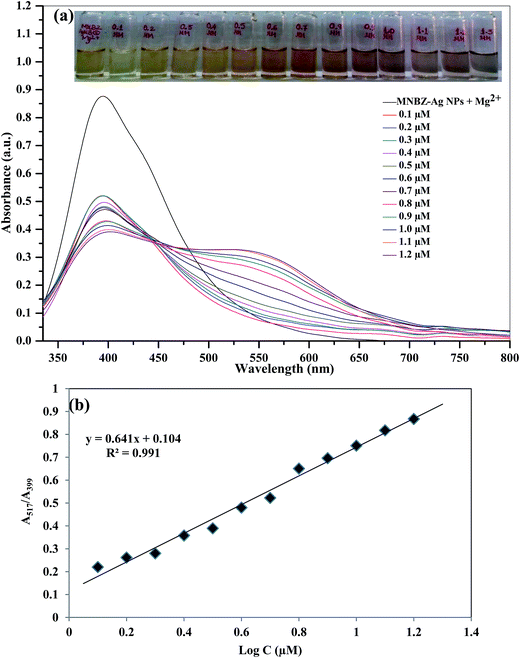 |
| Fig. 6 (a) UV-visible absorption spectra of MNBZ-Ag NPs after the addition of different concentrations of glyphosate (0.1, 0.2, 0.3, 0.4, 0.5, 0.6, 0.7, 0.8, 0.9, 1.0, 1.1, 1.2 μM) using Mg2+ ion as a tuner (50 μM) at PBS pH 4. Inset is the photographic images illustrating the corresponding colorimetric response. (b) A calibration plot of the SPR band shift relative to MNBZ-Ag NPs–Mg2+ ion (A517/A399) versus the logarithm of concentration of glyphosate in the range of 0.1–1.2 μM at PBS pH 4. | |
As shown in Fig. 6a and 7, and ESI of Fig. S7b,† the SPR peak has an obvious red-shift from 405 to 500 nm (without Mg2+ ion) and from 399 to 517 nm (with Mg2+ ion) by the addition of glyphosate, and the peak is broaden after the addition of glyphosate with Mg2+ ion. These spectral characteristics indicate that the aggregation of Ag NPs induced by glyphosate with Mg2+ ion as a tuner, which typically causes a massive redshift in the SPR peak of Ag NPs as well as a peak broadening, facilitating to enhance the near-field coupling of adjacent Ag NPs. Furthermore, the degree of the interparticle distance and the aggregation of Ag NPs are highly influenced on the position of SPR peak and shape,44 resulting a red-shift in their SPR peak with band broadening based on their degree of aggregation and distance.45 Therefore, the MNBZ-Ag NPs show the SPR band at 405 nm and the SPR band was red-shifted to 500 nm by the addition of glyphosate without Mg2+ ion (ESI of Fig. S7b†). However, the SPR band at 399 nm was red-shifted to 517 nm and the SPR band was broadening by the addition of glyphosate with Mg2+ ion as a tuner (Fig. 6a and 7), suggesting that the formation of MNBZ-Ag NPs–glyphosate–Mg2+ ion aggregates with large size, which is due to the dominate contributions from higher order electron oscillations. This is probably because Mg2+ ion has strong affinity to the phosphate group of glyphosate and nitro group of MNBZ-Ag NPs, thereby reducing interparticle distance via complexation, which leads to improve the colorimetric sensitivity and accelerate the color change. Compared with reported works on glyphosate detection, this MNBZ-Ag NPs–Mg2+ ion-based colorimetric method possesses high detection reliability, fast response, and lower detection limits than the CE,9 GC-MS,10 and colorimetric method24 and fair dynamic range (Table 1), and showing great potential for practical on-site glyphosate detection. Moreover, this method is particularly valuable for practical preliminary on-site screening application, as it shows a sharp and rapid color change at the threshold of 0.6 μM, which could be clearly differentiated by naked-eye without the assistance of any instrument.
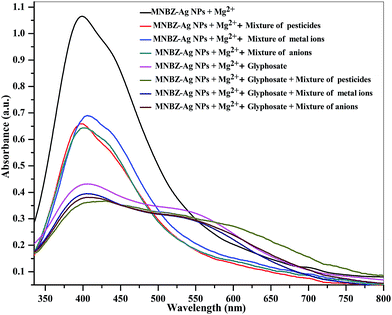 |
| Fig. 7 UV-visible absorption spectra of MNBZ-Ag NPs with glyphosate (10 μM) upon the addition of mixture of pesticides (acephate, monocrotophos, quinalphos, chlorpyrifos, triazophos, profenophos and dichlorvos, 1.0 mM), metal ions (Na+, K+, Cu2+, Zn2+, Cd2+, Fe2+, Mn2+, Mg2+, Co2+, Pb2+, Hg2+, Ni2+, Ca2+, Ba2+, Cr3+, Fe3+ and Al3+, 1.0 mM) and anions (Cl−, I−, Br−, NO3−, CH3COO−, SO42−, S2−, Cr2O72−, 1.0 mM) using Mg2+ ion (50 μM) as a tuner at PBS pH 4. Inset is the photographic image illustrating the corresponding solutions. | |
Table 1 Comparison of present method linear range and detection of limit of glyphosate with reported methods
Name of the nanomaterials |
Linear range (M) |
LOD (M) |
Technique |
Application |
Reference |
— |
— |
2.9 × 10−5 |
CE |
Natural water |
9 |
— |
1.8 × 10−5 to 5.9 × 10−4 |
5.9 × 10−6 |
GC-MS |
Human serum |
10 |
— |
1.2 × 10−10 to 2.9 × 10−9 |
2.9 × 10−11 |
LC-ESI-MS/MS |
Water |
12 |
— |
— |
2.2 × 10−7 |
Coulometry |
|
13 |
Oligopeptide-Au NPs-based chip |
0–6.4 × 10−5 |
5.8 × 10−7 |
SPR-based sensor |
— |
19 |
TGA-CdTe-QDs |
1.2 × 10−10 to 1.2 × 10−8 |
5.8 × 10−11 |
Fluorescence |
Apples |
20 |
DNA-labeled Au NPs |
5.9 × 10−8 to 5.9 × 10−4 |
5.9 × 10−8 |
Fluorescence |
— |
21 |
Fluorescence magnetic NPs |
1.0 × 10−9 to 1.0 × 10−5 |
1.0 × 10−9 |
Fluorescence |
— |
22 |
Cysteamine–Au NPs |
5.0 × 10−7 to 7 × 10−6 |
5.8 × 10−8 |
UV-visible |
Water |
23 |
Cd–PVA |
5.9 × 10−7 to 2.9 × 10−3 |
5.9 × 10−7 |
UV-visible |
Water |
24 |
MNBZ-Ag NPs–Mg2+ ion |
1.0 × 10−7 to 1.2 × 10−6 |
1.7 × 10−8 |
UV-visible |
Water, potato and corn |
Present work |
3.7. Selectivity of the method
A major shortcoming associated with some conventional methods for quantitation of glyphosate was the serious interference arising from other pesticides, and inorganic species because of their similar properties and structures. Under optimum conditions, the influence of other pesticides and inorganic species (metal ions and anions) was investigated on the aggregation of MNBZ-Ag NPs induced by glyphosate using Mg2+ ion as a tuner. Fig. 7 shows the UV-visible absorption spectra and the color changes of MNBZ-Ag NPs in the presence of glyphosate with mixture of pesticides (acephate, monocrotophos, quinalphos, chlorpyrifos, triazophos, profenophos and dichlorvos, 1.0 mM), metal ions (Na+, K+, Cu2+, Zn2+, Cd2+, Fe2+, Mn2+, Mg2+, Co2+, Pb2+, Hg2+, Ni2+, Ca2+, Ba2+, Cr3+, Fe3+ and Al3+, 1.0 mM) and anions (Cl−, I−, Br−, NO3−, CH3COO−, SO42−, S2−, Cr2O72−, 1.0 mM) at PBS pH 4. It can be noticed that the glyphosate was only induced the aggregation of MNBZ-Ag NPs–Mg2+ ion, resulting a red-shift from 399 to 517 nm and a color change from yellow to orange red. This result suggested that the coexistence of pesticides and inorganic species in the system of MNBZ-Ag NPs–Mg2+ did not affect the detection of glyphosate. The nature of response of the chemical species (pesticides, metal ions and anions) did not match with that of glyphosate, which makes that the system is selective for glyphosate detection. Overall, this finding suggests that the normally encountered similar pesticides and inorganic species do not pose a problem in the quantitative detection of glyphosate.
3.8. Practical application
It is well known that glyphosate is soluble in water, polar organic solvents (MeOH and EtOH) and insoluble in non-polar solvents (toluene, benzene and cyclohexane). Furthermore, glyphosate was extracted from food samples using various solvents including water (pH 6.5), MeOH and acetonitrile.10,46 Therefore, in order to know the best solvent for the extraction of glyphosate from food samples, initially we studied the extraction efficiency of glyphosate using water and polar organic solvents (MeOH and EtOH) and its recovery was determined by afore said procedure. To this, the collected potato sample was fortified with 500, 750 and 1000 nM of glyphosate for 24 h at room temperature. Then, the extraction efficiency of glyphosate was investigated using water, MeOH and EtOH as extracting solvents and its recoveries were shown in ESI of Table S1.† It can be observed that the recovery ranges were found to be either almost same or slightly higher using methanol and water as extracting solvents. Furthermore, methanol is widely used as a solvent for the extraction of various polar compounds because of its low boiling point (65 °C). Therefore, we selected methanol as the best solvent for the extraction, easy evaporation and rapid concentration of glyphosate from the food samples. We employed our proposed method, which has high sensitivity and selectivity, for the practical analyses of glyphosate in water, potato and corn samples. To this, the collected water (tap, river and canal water), potato and corn samples were spiked with glyphosate (500, 750 and 1000 nM) and the amount of glyphosate was estimated by the standard addition method and the results were shown in Table 2 and ESI of Fig. S8.† It can be seen that the recoveries of this assay vary from 95.6% to 101.0% for intra-day and from 96.6 to 101.6% for inter-day, indicating that the proposed assay exhibit good precision and accuracy and has promising feasibility for the rapid and sensitive detection of glyphosate in real samples. The obtained results show this probe was capable of analyzing glyphosate with high selectivity and sensitivity and shown obvious advantage such as without complicated sample preparations and expensive apparatus, short analysis time and samples at microvolume. Therefore, the proposed method was able to be utilized for detection of glyphosate in complex samples with miniaturized sample preparations within 1.0 min.
Table 2 Analysis of glyphosate in spiked water, potato and corn samples at inter- and intra-day using MNBZ-Ag NPs as a probe and Mg2+ ion as a tuner
Analyte |
Known concentration (nM) |
Intra-day |
Inter-day |
Found concentrationa (nM) |
% recoveryb |
Precisionc (%) |
Accuracyd (%) |
Found concentrationa (nM) |
% recoveryb |
Precisionc (%) |
Accuracyd (%) |
Mean ± standard deviation (n = 3). % recovery = (found concentration/known concentration) × 100. Precision was calculated (RSD, %) from standard deviation/mean × 100. Accuracy was calculated from (found concentration − known concentration)/known concentration × 100. |
Tap water |
500 |
487 ± 0.88 |
97.4 |
1.9 |
−2.6 |
483 ± 0.58 |
96.6 |
2.1 |
−3.3 |
750 |
742 ± 0.97 |
98.3 |
1.2 |
−1.1 |
732 ± 0.57 |
97.6 |
0.9 |
−2.4 |
1000 |
1010 ± 0.87 |
101.0 |
1.2 |
+1.0 |
1010 ± 0.65 |
101.0 |
0.4 |
+1.1 |
River water |
500 |
478 ± 0.09 |
95.6 |
1.2 |
−4.3 |
483 ± 0.21 |
96.6 |
0.2 |
−3.4 |
750 |
737 ± 0.70 |
97.3 |
0.7 |
−1.6 |
744 ± 0.18 |
99.2 |
0.2 |
−1.5 |
1000 |
1003 ± 0.87 |
100.3 |
0.9 |
+0.3 |
993 ± 0.53 |
99.3 |
1.4 |
−0.6 |
Canal water |
500 |
493 ± 0.87 |
98.6 |
3.2 |
−1.3 |
492 ± 0.73 |
98.4 |
1.1 |
−1.4 |
750 |
749 ± 0.77 |
99.8 |
1.2 |
−0.1 |
740 ± 0.51 |
98.6 |
2.1 |
−1.3 |
1000 |
997 ± 0.50 |
99.7 |
0.9 |
−0.3 |
1002 ± 0.54 |
100.2 |
1.1 |
+0.2 |
Potato |
500 |
498 ± 0.61 |
99.6 |
1.2 |
+0.2 |
501 ± 0.83 |
100.2 |
1.7 |
+0.3 |
750 |
750 ± 0.42 |
100.0 |
1.2 |
+0.4 |
742 ± 0.21 |
98.9 |
1.8 |
+0.3 |
1000 |
1004 ± 0.55 |
100.4 |
0.8 |
+0.9 |
998 ± 0.61 |
99.8 |
1.8 |
−0.4 |
Corn |
500 |
499 ± 0.73 |
99.8 |
1.0 |
−0.1 |
508 ± 0.24 |
101.6 |
0.5 |
+1.1 |
750 |
752 ± 0.21 |
100.2 |
0.4 |
+0.3 |
749 ± 0.05 |
99.9 |
0.1 |
−0.1 |
1000 |
1008 ± 0.90 |
100.8 |
0.9 |
+0.8 |
997 ± 0.89 |
99.7 |
1.5 |
−0.3 |
4. Conclusions
In this work, we have reported a new simple and sensitive colorimetric assay for glyphosate based on the fact that glyphosate can induce the aggregation of MNBZ-Ag NPs using Mg2+ ion as a tuner, resulting in yellow to orange to orange-red change which can be easily measured with UV-visible spectrophotometer. The use of Mg2+ ion as a tuner allows selective binding of glyphosate with the surfaces of MNBZ-Ag NPs, which leads to improve the sensitivity of method 103 time lower than that of the only MNBZ-Ag NPs. It is demonstrated that the affinity of Mg2+ ion toward the deprotonated phosphate group of glyphosate and nitro group of MNBZ-Ag NPs induces the aggregation of MNBZ-Ag NPs following by distinct color change at nanomolar concentration of glyphosate, providing lower detection limit of 17.1 nM. The probe is successfully applied for sensing glyphosate in water, potato and corn samples with reduced sample preparations. We envision that MNBZ-Ag NPs–Mg2+ ion-based nanosensor technology for fabricating organophosphorus pesticides sensors with dynamic optical ranges will lead to practical applications from sophisticated equipments to equipment-free colorimetric sensors.
Acknowledgements
The authors greatly thank Gharda Chemicals Limited, Atul Ltd, Super Crop Safe Ltd and United Phosphorus Limited for providing pesticide samples to this work. This work was financially supported by S. V. National Institute of Technology, Surat under Institute Research Grant (Dean (R&C)/1503/2013-2014). We also thank Department of Science and Technology for providing Maya Pro 2000 spectrophotometer under the Fast-Track Young Scientist Scheme (SR/FT/CS-54/2010). Authors also thank the Head, Chemical Engineering Department, SVNIT, Surat for providing DLS facilities to this work.
References
- C. F. B. Coutinho, L. F. M. Coutinho, L. H. Mazo, S. L. Nixdorf, C. A. P. Camara and F. M. Lancas, Anal. Chim. Acta, 2007, 592, 30–35 CrossRef CAS PubMed.
- M. Piriyapittaya, S. Jayanta, S. Mitra and N. Leepipatpiboon, J. Chromatogr. A, 2008, 1189, 483–492 CrossRef CAS PubMed.
- Drinking Water Standards and Health Advisories, United States Environmental Protection Agency (USEPA), Washington, DC, 2011.
- The EU-MRLs set in Regulation (EC) No 396/2005 from the European Union pesticide database, http://www.ec.europa.eu/sanco_pesticides/public/index.cfm.
- Chinese National Standards GB 2763-2012, National food safety standard maximum residue limits for pesticides in food, Standards Press of China, Beijing, 2012 Search PubMed.
- WHO, Summary Statement: Glyphosate and AMPA in Drinking Water, WHO Guidelines for Drinking Water Quality, World Health Organization, Geneva, 3rd edn, 2004 Search PubMed.
- M. V. Khrolenko and P. P. J. Wieczorek, J. Chromatogr. A, 2005, 1093, 111–117 CrossRef CAS PubMed.
- M. Kim, J. Stripeikis, F. Inon and M. A. Tudino, Talanta, 2007, 72, 1054–1058 CrossRef CAS PubMed.
- M. Corbera, M. Hidalgo, V. Salvado and P. P. Wieczorek, Anal. Chim. Acta, 2005, 540, 3–7 CrossRef CAS.
- M. Motojyuku, T. Saito, K. Akieda, H. Otsuka, I. Yamamoto and S. Inokuchi, J. Chromatogr. B: Anal. Technol. Biomed. Life Sci., 2008, 875, 509–514 CrossRef CAS PubMed.
- T. Saito, H. Aoki, A. Namera, H. Oikawa, S. Miyazaki, A. Nakamoto and S. Inokuchi, Anal. Sci., 2011, 27, 999–1005 CrossRef CAS PubMed.
- M. Ibáñez, O. J. Pozo, J. V. Sancho, F. J. López and F. Hernández, J. Chromatogr. A, 2005, 1081, 145–155 CrossRef.
- C. F. B. Coutinho, L. F. M. Coutinho, L. H. Mazo, S. L. Nixdorf and C. A. P. Camara, J. Chromatogr. A, 2008, 1208, 246–249 CrossRef CAS PubMed.
- G. P. Martínez, N. Laguarda-Miro, J. S. Camino and R. M. Peris, Talanta, 2013, 115, 702–705 CrossRef PubMed.
- V. N. Mehta, S. K. Kailasa and H. F. Wu, J. Nanosci. Nanotechnol., 2014, 14, 447–459 CrossRef CAS PubMed.
- S. K. Kailasa and H. F. Wu, TrAC, Trends Anal. Chem., 2015, 65, 54–72 CrossRef CAS.
- S. K. Kailasa, V. N. Mehta and H. F. Wu, RSC Adv., 2014, 4, 16188–16205 RSC.
- S. K. Kailasa and H. F. Wu, Microchim. Acta, 2013, 180, 405–413 CrossRef CAS.
- X. Ding and K. L. Yang, Anal. Chem., 2013, 85, 5727–5733 CrossRef CAS PubMed.
- J. Guo, Y. Zhang, Y. Luo, F. Shen and C. Sun, Talanta, 2014, 125, 385–392 CrossRef CAS PubMed.
- H. U. Lee, H. Y. Shin, J. Y. Lee, Y. S. Song, C. Park and S. W. Kim, J. Agric. Food Chem., 2010, 58, 12096–12100 CrossRef CAS PubMed.
- H. U. Lee, D. U. Jung, J. Y. Lee, Y. S. Song, C. Park and S. W. Kim, Sens. Actuators, B, 2013, 177, 879–886 CrossRef CAS.
- J. Zheng, H. Zhang, J. Qu and Q. Z. X. Chen, Anal. Methods, 2013, 5, 917–924 RSC.
- L. K. S. De Almeida, S. Chigome, N. Torto, C. L. Frost and B. I. Pletschke, Sens. Actuators, B, 2015, 206, 357–363 CrossRef CAS.
- J. V. Rohit, J. N. Solanki and S. K. Kailasa, Sens. Actuators, B, 2014, 200, 219–226 CrossRef CAS.
- J. V. Rohit and S. K. Kailasa, Anal. Methods, 2014, 6, 5934–5941 RSC.
- G. M. Patel, J. V. Rohit, R. K. Singhal and S. K. Kailasa, Sens. Actuators, B, 2015, 206, 684–691 CrossRef CAS.
- J. V. Rohit and S. K. Kailasa, J. Nanopart. Res., 2014, 16, 2585–2600 CrossRef.
- L. D. Carlos, R. A. S. Ferreira, V. de Zea Bermudeza and S. J. L. Ribeiro, Adv. Mater., 2009, 21, 509–534 CrossRef CAS PubMed.
- L. D. Carlos, R. A. S. Ferreira, V. de Zea Bermudez, B. Julián-Loópez and P. Escribano, Chem. Soc. Rev., 2011, 40, 536–549 RSC.
- C. Pezzato, S. Maiti, J. L.-Y. Chen, A. Cazzolaro, C. Gobbo and L. J. Prins, Chem. Commun., 2015, 51, 9922–9931 RSC.
- B. Makhinson, A. K. Duncan, A. R. Elam, A. de Bettencourt-Dias, C. D. Medley, J. E. Smith and E. J. Werner, Inorg. Chem., 2013, 52, 6311–6318 CrossRef CAS PubMed.
- S. Zhang, J. Wang, L. Han, C. Li, W. Wang and Z. Yuan, Sens. Actuators, B, 2010, 147, 687–690 CrossRef CAS.
- C.-J. Yu, T.-L. Cheng and W.-L. Tseng, Biosens. Bioelectron., 2009, 25, 204–210 CrossRef CAS PubMed.
- C. Pezzato, P. Scrimin and L. J. Prins, Angew. Chem., Int. Ed., 2014, 53, 2104–2109 CrossRef CAS PubMed.
- J. F. Hainfeld, W. Q. Liu, C. M. R. Halsey, P. Freimuth and R. D. Powell, J. Struct. Biol., 1999, 127, 185–198 CrossRef CAS PubMed.
- R. B. P. Elmes, K. N. Orange, S. M. Cloonan, D. C. Williams and T. Gunnlaugsson, J. Am. Chem. Soc., 2011, 133, 15862–15865 CrossRef CAS PubMed.
- J. Massue, S. J. Quinn and T. Gunnlaugsson, J. Am. Chem. Soc., 2008, 130, 6900–6901 CrossRef CAS PubMed.
- T. Huang and R. W. Murray, Langmuir, 2002, 18, 7077–7081 CrossRef CAS.
- Y. Zhou, T. S. Zhou, M. Zhang and G. Y. Shi, Analyst, 2014, 139, 3122–3126 RSC.
- Z. Szabo, Coord. Chem. Rev., 2008, 252, 2362–2380 CrossRef CAS.
- A. L. R. Mercê, A. S. Mangrich, B. Szpoganicz, N. M. Levya and J. Felcman, J. Braz. Chem. Soc., 1996, 7, 239–245 CrossRef.
- G. R. Cayley and D. N. Hague, J. Chem. Soc., Faraday Trans. 1, 1972, 68, 2259–2265 RSC.
- Y. Liu, Y. Liu, R. L. Mernaugh and X. Zeng, Biosens. Bioelectron., 2009, 24, 2853–2857 CrossRef CAS PubMed.
- X. Huang and M. A. El-Sayed, J. Adv. Res., 2010, 1, 13–28 CrossRef.
- J. Han, H. Moon, Y. Hong, S. Yang, W. J. Jeong, K. S. Lee and H. Chung, Forensic Sci. Int., 2016, 265, 41–46 CrossRef CAS PubMed.
Footnote |
† Electronic supplementary information (ESI) available. See DOI: 10.1039/c6ra06450g |
|
This journal is © The Royal Society of Chemistry 2016 |