DOI:
10.1039/C6RA06017J
(Paper)
RSC Adv., 2016,
6, 39972-39981
Influence of anchoring group numbers in an efficient pyridine-anchor co-adsorbent of pyridinecarboxaldimine substituted aminonaphthalene on the performance of N719 sensitized solar cells†
Received
7th March 2016
, Accepted 14th April 2016
First published on 15th April 2016
Abstract
Unsymmetrical (N-(2-pyridinecarboxaldimine)-1-aminonaphthalene, USP) and symmetrical (N,N′-bis-(2-pyridinecarboxaldimine)-1,8-diaminonaphthalene, SDP) pyridine-anchor co-adsorbents with different numbers of anchoring groups are synthesized and employed in combination with a ruthenium complex N719 in dye sensitized solar cells (DSSCs). Both the prepared co-adsorbents can overcome the deficiency of N719 absorption in the low wavelength region of the visible spectrum, offset competitive visible light absorption of I3−, suppress the charge recombination and prolong the electron lifetime. The SDP co-adsorbent containing double anchoring groups shows better electronic coupling with TiO2 than the USP co-adsorbent with a single anchoring group. This enhanced electronic coupling leads SDP to exhibit better performance for DSSCs. A short circuit current density of 13.19 mA cm−2, an open circuit voltage of 0.75 V and a fill factor of 0.66 corresponding to an overall conversion efficiency of 6.51% under AM 1.5G solar irradiation are achieved when SDP is used as a co-adsorbent, which is much higher than that for DSSCs only sensitized by N719 (5.28%) under the same conditions.
1. Introduction
Dye-sensitized solar cells (DSSCs) based on nanoporous TiO2 photoanodes have attracted considerable interest as next-generation solar cells due to their low-cost production, non-vacuum process, environment friendly energy conversion, and fairly high performance since the outstanding breakthroughs made by the Grätzel group.1–8 However, much improvement in efficiency is desired for these devices to overcome the challenge of other types of solar cells before they could take the place of the Si based solar cells. Typical DSSCs are constructed with a dye-absorbed wide band gap semiconductor electrode, such as TiO2, an electrolyte containing the I−/I3− redox couple, and a Pt-coated counter electrode.1 The photo-sensitizing dye, as an important component of DSSCs, plays an important role in capturing photons, generating electron–hole pairs, and subsequently transferring them to the semiconductor and electrolyte, respectively. The most successful photosensitizers for DSSCs are ruthenium polypyridyl complexes (Ru-complex), which yield solar-to-electric power conversion efficiencies of above 11% under AM 1.5G.9,10 However, it is still possible to further improve the efficiency of the cells based on ruthenium sensitizers. To realize this, a co-sensitization method using two kinds of dyes has been often utilized and a record high performance of 12.3% was obtained by Grätzel et al. using this method,11 which stimulating investigation of the development of new co-sensitizers to promote performance of ruthenium complexes dyes sensitized DSSCs by co-sensitization. For example, the colleagues of Han and Arakawa obtained a high efficiency of above 11.0% by co-sensitization of black dye with organic complexes, respectively.12,13 Dehghani co-sensitized N719 with a porphyrin dye ZnTCPP, and the efficiency improved to 6.35% in comparison to N719 that the efficiency was 4.75%.14 Holliman co-sensitized N719 with triarylamine dyes, DSSC efficiency of 7.5% have been achieved which exceed those recorded for single dye devices.15 Lee reported a co-sensitized system using commercial ruthenium dye N719 with metal-free organic dye FL. The optimum cell efficiency of 5.10% was obtained, and this value is higher than that of the cell soaking only in N719 (4.89%).16 Fan published a co-sensitization study using Ru-complex JK-142 and organic JK-62 dye. The DSSC exhibited a 10.2% cell efficiency surpassing that of cells using only JK-142 dye (7.28%) or JK-62 dye (5.36%).17 Sharma obtained a cell efficiency of 7.35% by co-sensitization of zinc–porphyrin and thiocyanate-free ruthenium(II) terpyridine dyes.18 Wu co-sensitized N719 with an organic dye resulting in an efficiency of 7.91%, 8.6% higher than that of N719 sensitized alone.19 Chang reported efficient panchromatic light harvesting by co-sensitization of a porphyrin molecule and N719 in dye sensitized solar cells, the co-sensitized devices shows a considerably enhanced power conversion efficiency of 8.89%, higher than individually sensitized by N719.20 For the effective enhancement of the conversion efficiency of DSSCs by this method, one of the most important points is the select of co-adsorbent. It should has a compensate adsorption to ruthenium dye, a large molar molar extinction coefficient, and suitable energy level etc. It is expected that the conversion efficiency of the DSSCs with one kind of dye is improved effectively by the addition of a co-adsorbent with suitable features. However, the conversion efficiency quite often decreases with the use of a co-adsorbent. One of the main reasons for this is thought to be the decrease in the amount of the main dye adsorbed on the TiO2 photoelectrode due to competitive adsorption between the main and co-adsorbed dyes.21 Therefore, co-adsorbents having different molecular shapes and sizes from those of the main dyes have been employed to reduce the competitive adsorption between the two dyes in the above mentioned studies. Recently co-adsorbents containing pyridine-anchor unit instead of the conventional carboxyl-anchor group is proved to be effective in co-sensitized DSSCs and these pyridine-anchor dyes were confirmed to adsorb preferentially at the Lewis acid sites of the TiO2 surface (the sites of exposed Ti atoms), which explore a new type of co-sensitizer in improving the performance of DSSCs.22–26 On the other hand, most dyes containing the conventional carboxyl-anchor unit adsorb at the Brösted acid sites of the TiO2 surface (the sites of hydroxyl groups bound to Ti atoms) via dehydration reactions.27 Therefore, it is expected that competitive adsorption will not occur between pyridine-anchor dyes and conventional carboxyl anchor dyes, such as N719.
Accordingly, in this study, two pyridine-anchor dyes with different numbers of anchoring groups (SDP and USP, Scheme 1) were synthesized and employed as co-adsorbents for ruthenium dye N719 based DSSCs to obtain insight into the effective combination of two different dyes in the co-sensitization method, and the effect of the number of anchoring groups on the photovoltaic performance is investigated systemically by comparing the photo physical properties of SDP and USP. By co-sensitizing these co-adsorbents with N719, respectively, all the co-sensitized devices exhibited enhancements of photovoltaic performance. Spectral and electrochemical characterizations indicate that the prepared co-adsorbents can be used to absorb light in the low wavelength region of the visible spectrum, overcome the competitive light absorption by I−/I3−, reduce charge recombination and prolong electron lifetime. What's more, these co-adsorbents possess stronger adsorption in the low wavelength region of 300–450 nm, are easier to be produced with high yields and cheaper than noble metal dyes, therefore they are promising candidates as co-sensitizers for highly efficient DSSCs and do help to improve the devices performance.
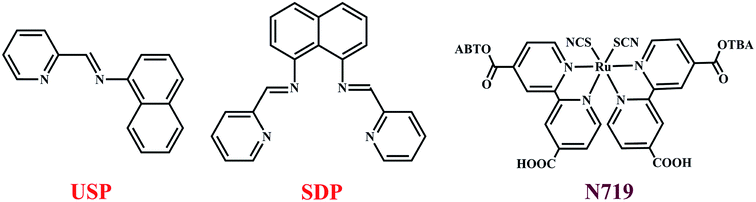 |
| Scheme 1 Molecular structures of USP, SDP and N719. | |
2. Experimental section
2.1. Synthesis of SDP and USP
N-(2-Pyridinecarboxaldimine)-1-aminonaphthalene (USP): a mixture of 2-pyridinecarboxaldehyde (1.0 mL, 10.51 mmol) and 1-aminonaphthalene (1.51 g, 10.53 mmol) was refluxed in anhydrous methanol (30 mL) in the presence of a catalytic amount of formic acid for 5 h. After the reaction was over, the resulting solution was concentrated under reduced pressure (oil pump) to obtain the brown products. The crude product was recrystallized from n-hexane and vacuo dried. Yield: 1.96 g (80%). Anal. calcd for USP [C16H12N2 (232.19)]: C, 82.73; H, 5.21; N, 12.06%. Found: C, 82.69; H, 5.25; N, 12.08%. 1H NMR (400 MHz, CDCl3, 298 K): δ 8.63 (s, 1H, CH
N), 8.37 (d, 1H, Py-H6), 7.30–7.81 (m, 7H, Ar-H), 7.46–7.73 (m, 3H, Py-H3, H4 and H5) ppm. 13C NMR (150 MHz, CDCl3, 298 K): δ 120.79, 121.36, 123.65, 124.59, 124.86, 125.84, 126.32, 127.89, 128.53, 129.73, 132.58, 134.36, 138.11, 142.02, 148.38, 149.44, 164.12 ppm. HRMS (ESI): m/z = 233.17 [M + H]+. FT-IR (KBr): ν 3356(w), 2949(w), 2865(w), 1625(m), 1586(m), 1514(w), 1463(w), 1434(w), 1406(m), 1376(w), 1346(w), 1290(w), 1250(w), 1220(w), 1149(w), 1119(w), 1054(s), 1033(vs), 1016(s), 857(w), 791(w), 772(s), 696(w), 623(w), 571(w) cm−1. UV-vis (in ethanol, 298 K): 211, 243, 331 nm.
N,N′-Bis-(2-pyridinecarboxaldimine)-1,8-diaminonaphthalene (SDP): the procedure is similar to that of USP, except that 1,8-diaminonaphthalene (0.85 g, 5.33 mmol) was used instead of 1-aminonaphthalene. The product was filtered, washed several times with methanol and vacuo dried. Yield: 3.01 g (85%). Anal. calcd for SDP [C22H16N4 (336.40)]: C, 78.55; H, 4.79; N, 16.66%. Found: C, 78.58; H, 4.75; N, 16.63%. 1H NMR (400 MHz, CDCl3, 298 K): δ 8.53 (s, 2H, CH
N), 8.33 (d, 2H, Py-H6), 7.61–8.12 (m, 6H, Ar-H), 7.24–7.49 (m, 6H, Py-H3, H4 and H5) ppm. 13C NMR (150 MHz, CDCl3, 298 K): δ 121.62, 122.77, 124.10, 125.76, 135.62, 137.20, 138.17, 148.08, 148.87, 150.12, 164.89 ppm. HRMS (ESI): m/z = 337.37 [M + H]+. FT-IR (KBr): ν 3338(w), 2949(w), 2820(w), 1624(w), 1601(s), 1571(w), 1521(w), 1470(m), 1417(m), 1377(m), 1341(w), 1310(w), 1289(w), 1248(w), 1165(w), 1089(w), 1033(m), 996(w), 813(m), 761(m), 689(w), 622(w), 563(w) cm−1. UV-vis (in ethanol, 298 K): 203, 235, 345 nm. The synthesis of USP and SDP are summarized in Scheme 2.
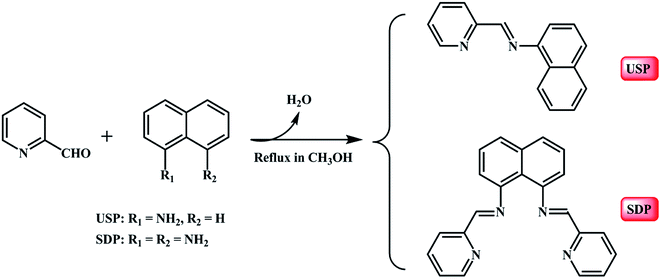 |
| Scheme 2 Synthesis routes of USP and SDP. | |
2.2. Fabrication of devices
TiO2 paste for screen printing was prepared according to the literature.28 It was printed onto conductive glass (FTO, 15 Ω sq.−1, 90% transmittance in the visible, NSG, Japan) and then dried at 100 °C for 5 min. The above process was repeated for six times. The obtained TiO2 film was then sintered at 500 °C for 15 min, after which process a transparent TiO2 photoelectrode with the thickness of 10 μm was obtained. Stepwise co-sensitization of the TiO2 film was accomplished by dipping it in various solutions for different time intervals at room temperature. Typically, the photoanode was immersed in a solution of 0.3 mM USP in absolute ethanol for 2 h and then washed with ethanol. It was further immersed in a solution of 0.3 mM N719 [di-tetrabutylammonium cis-bis(isothiocyanato)bis(2,2′-bipyridyl-4,4′-dicarboxylato)ruthenium(II)] in absolute ethanol for 12 h and then washed with ethanol. The sandwich-type solar cell device was assembled by placing a platinum-coated conductive glass as counter electrode on the co-sensitized photoanode, a drop of liquid electrolyte containing 0.5 M LiI, 0.05 M I2, 0.1 M 4-tert-butylpyridine was added to fill the void between two electrodes and clipped together as open cells for measurement.
2.3. Instrumentation and measurements
UV-visible absorption spectra were recorded on SPECORD S600 spectrophotometer (Jena, Germany) for samples in ethanol solution and UV-2250 spectrophotometer (Shimadzu, Japan) for sensitized TiO2 films, respectively. Cyclic voltammetry were recorded using a CHI660D electrochemical potentiostat. The measurements were carried out in a three-electrode cell under argon. The working electrode was a planar platinum working electrode and the auxiliary electrode was a platinum wire. The reference electrode was a saturated calomel reference electrode in saturated KCl solution. A solution of 0.1 M TBAPF6 in dry ethanol was used as electrolyte. Time-dependent density functional theory (TD-DFT) calculations were conducted by using the B3LYP/6-31G basis set. The theoretical molecular orbital levels of HOMO and LUMO in ethanol were achieved with the B3LYP/6-31G basis set implemented in the Gaussian 03 package. Photocurrent–photovoltage (I–V) curves were recorded by Keithley model 2400 digital source meter under AM 1.5G irradiation. The incident light intensity was 100 mW cm−2 calibrated by a standard silicon solar cell. The working areas of the cells were masked to 0.16 cm−2. Based on I–V curve, the fill factor (FF) is defined as: FF = (Jmax × Vmax)/(Jsc × Voc), where Jmax and Vmax are the photocurrent density and photovoltage for maximum power output; Jsc and Voc are the short-circuit photocurrent density and open-circuit photovoltage, respectively. The overall energy conversion efficiency η is defined as: η = (FF × Jsc × Voc)/Pin where Pin is the power of the incident light. The measurement of the incident photon-to-current conversion efficiency (IPCE) was performed by an EQE/IPCE spectral response system (Newport). The amounts of absorbed dye were measured by desorbing the dye from the dye-sensitized films in a 0.1 M NaOH solution in water and ethanol (1
:
1, v/v), and then the concentration of desorbed dye in the NaOH solution was measured using an UV-visible spectrophotometer. EIS were recorded by CHI660D Electrochemical Analyzer (Chenhua, China), and the measurements were taken over a frequency range of 0.1–100 kHz under standard global AM1.5 solar irradiation (100 mW cm−2) or in the dark by applying a forward bias of −0.75 V. Open-circuit voltage decay curves (OCVD) and dark current were also recorded by CHI660D Electrochemical Analyzer. 1H NMR (400 MHz) spectra were recorded on a Bruker Avance-400 spectrometer using Si(CH3)4 as an internal standard at room temperature. 13C NMR (150 MHz) spectra were recorded on a Bruker Avance-600 spectrometer. HRMS (ESI) spectra were recorded on a Bruker Esquire LC mass spectrometer using electrospray ionization. Infrared spectra (IR) were obtained from KBr pellets on a Nicolet Avatar-360 Ingrared spectrometer in the 4000–400 cm−1 region. Elemental analyses were performed on a Perkin-Elmer 2400 element analyzer.
3. Results and discussions
3.1. Synthesis and optical properties of USP and SDP
USP and SDP are synthesized with a different number of anchoring groups, using the synthetic routes depicted in Scheme 2 and were obtained in good yields (ca. 80–85%). Both USP and SDP were characterized by 1H NMR, 13C NMR, ESI-HRMS, UV-vis, and IR spectroscopy as well as element analysis and all the data are listed in the experimental section. The 1H NMR, 13C NMR and ESI-HRMS spectra of USP and SDP are shown in Fig. S1–S3,† respectively (see the ESI†).
The absorption spectra of USP, SDP and N719 in ethanol solutions are shown in Fig. 1a, and the corresponding data are listed in Table 1. As shown in Fig. 1a, each of the prepared compounds USP and SDP exhibits two major prominent bands. The first one at ca. 200–250 nm is attributed to localized aromatic π–π* transitions and the other one at ca. 300–400 nm is attributed to intramolecular charge transfer (ICT). Compared with the absorption spectrum of N719, apparently, the absorption spectra of USP and SDP could compensate for that of N719 in the low wavelength region, especially in the region of 300–400 nm. Furthermore, the molar extinction coefficients in the visible region are 33
903 M−1 cm−1 for USP and 35
899 M−1 cm−1 for SDP. All of these are much higher than that of the ruthenium complex N719.29 A higher molar extinction coefficient means a higher light harvesting ability in the wavelength region of 300–400 nm compared with N719 and I3− (25
000 M−1 cm−1).30 Hence it can be predicted that the photon lost due to the light absorption by I3− will be suppressed by employing prepared compound as a co-adsorbent due to the competition between the prepared compound and I3− to absorb light.
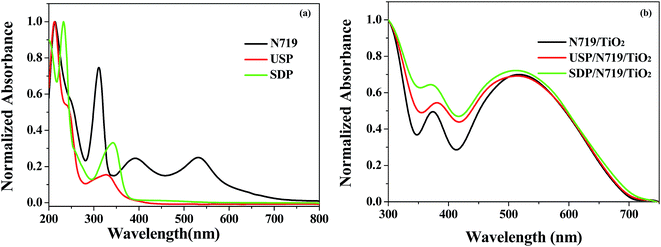 |
| Fig. 1 UV-visible absorption spectra of USP, SDP and N719 (a) in ethanol, (b) on TiO2 film. | |
Table 1 Experimental data for spectral and electrochemical properties of the prepared co-adsorbents
Compounds |
λabsa (nm) |
εa (M−1 cm−1) |
λema,b (nm) |
E0–0c (eV) |
EHOMOd (eV) |
ELUMOd (eV) |
HOMOe (eV) |
LUMOe (eV) |
Absorption and emission spectra were recorded in ethanol solution (3 × 10−4 M) at room temperature. Dyes were excited at their absorption maximum value. Optical band gap calculated from intersection between the absorption and emission spectra. The values of EHOMO and ELUMO were calculated with the following formula: EHOMO = −(Eoxonset + 4.4) (eV); ELUMO = EHOMO + E0–0. B3LYP/6-31G calculated values. |
USP |
331 |
33 903 |
429 |
2.91 |
−5.02 |
−2.11 |
−5.09 |
−2.15 |
SDP |
345 |
35 899 |
451 |
2.98 |
−5.06 |
−2.08 |
−5.12 |
−2.06 |
To confirm the compensated and suppressed function of USP and SDP, the absorption spectra of USP/N719 and SDP/N719 sensitized TiO2 films are recorded and shown in Fig. 1b. As shown in Fig. 1b, the absorption of N719 on TiO2 film in visible light region is remarkably broadened due to the electronic coupling of the dyes on the TiO2 surface.31 What's more, the wider and higher adsorption intensity of SDP co-sensitized photoanode indicates that stronger electronic coupling is formed between SDP and TiO2 than between USP and TiO2. And when N719 was combined with USP and SDP, respectively, its absorption in the region of 300–500 nm on TiO2 film was enhanced. The enhancement of absorption in the region of 300–500 nm is consistent with the result that the prepared compounds could compensate for that of N719 in the low wavelength region of the visible spectrum based on the spectra shown in Fig. 1a.
3.2. Energy matching analysis of USP and SDP
Energy matching is crucial in selecting sensitizers for DSSCs. Therefore, in order to evaluate if there are favorable energy offsets of the USP and SDP with respect to the TiO2 film and the electrolyte, the HOMO and LUMO energy levels of USP and SDP were investigated by cyclic voltammetry (CV) measurement in a three-electrode cell and an electrochemistry workstation. The experimental data are summarized in Table 1. As estimated from the intersection of the absorption and emission spectra, the excitation transition energy (E0–0) of USP and SDP are 2.91 and 2.98 eV, respectively. Consequently, the HOMO values of USP and SDP are calculated based on their first redox potentials as −5.02 and −5.06 eV, respectively, and the LUMO levels of USP and SDP calculated from EHOMO + E0–0, are −2.11 and −2.08 eV, respectively.32
The HOMO and LUMO energy levels of USP and SDP are shown in Fig. 2. It shows that the energy levels of USP and SDP are appropriate for the DSSCs system containing TiO2. The LUMO levels lied above the conduction band of the TiO2 semiconductor (−4.40 eV vs. vacuum), indicating efficient electron injection, and the HOMO energy levels lied below the I−/I3− redox electrolyte (−4.60 eV vs. vacuum) which is further improved negatively about 0.3 V by adding additives such as 4-tert-butyl pyridine to the I−/I3− redox electrolyte,33 providing sufficient driving force for dye regeneration.34
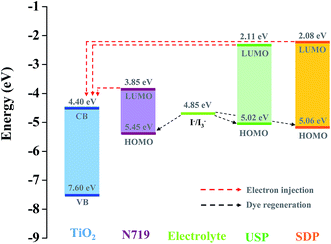 |
| Fig. 2 Energy diagram of HOMO and LUMO for USP and SDP compared to the energy levels of materials in DSSCs. | |
To assist our qualitative understanding of the electrochemical behavior of USP and SDP, we performed time-dependent density functional theory (TD-DFT) calculation to investigate the structure and charge-transfer properties at the B3LYP/6-31G basis set. The electronic distributions of the frontier molecular orbital of USP and SDP are shown in Fig. 3. As shown in the figure, the electronic density of USP and SDP is mostly localized over the aminonaphthalene group at the HOMO, but localized over the anchoring group of pyridine ring at the LUMO. This strong intramolecular charge transfer nature under illumination would result in efficient electron injection from the excited dye to TiO2 conductive band. In contrast, the LUMO of SDP is delocalized in the entire chromophore, which might enhance the electron coupling with TiO2 and the electron extraction pathways. The HOMO and LUMO energy levels of USP and SDP, estimated from DFT calculations are −5.09 and −2.15 eV, −5.12 and −2.06 eV, respectively. It is consistent with the CV results that the energy levels of the synthesized compounds are suitable for electron injection and dye regeneration thermodynamically.
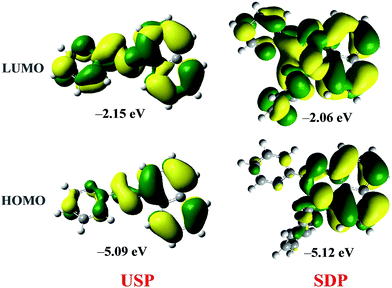 |
| Fig. 3 The frontier molecular orbitals of the HOMO and LUMO levels of USP and SDP calculated with B3LYP/6-31G. | |
3.3. Photovoltaic performance of DSSCs
The co-adsorbents of USP and SDP are applied in DSSCs followed a stepwise co-sensitization procedure by sequentially immersing the TiO2 electrode (with thickness of ca. 10 μm) in separate solution of N719 and USP or SDP. In one case, the TiO2 electrode was firstly immersed into the USP solution and secondly in the N719 solution resulting in device USP/N719/TiO2. The optimum soaking time for the TiO2 photoanode film in USP and N719 was found to be 2 h and 12 h, respectively. The device of SDP/N719/TiO2 is fabricated in the same way. For comparison purpose, device N719/TiO2 sensitized by the individual N719 was also fabricated under the same experimental conditions.
The current–voltage (J–V) characteristic of the DSSCs devices based on different photoanodes under illumination (AM 1.5G, 100 mW cm−2) are shown in Fig. 4, and the corresponding cells performance are summarized in Table 2. The individually N719 sensitized device was found to exhibit η value of 5.28% (with Jsc = 11.22 mA cm−2, Voc = 0.71 V, and FF = 0.66). The co-sensitized solar cell devices USP/N719 and SDP/N719 showed enhanced η value of 6.40% (with Jsc = 12.73 mA cm−2, Voc = 0.75 V, and FF = 0.67), and 6.51% (with Jsc = 13.19 mA cm−2, Voc = 0.75 V, and FF = 0.66), respectively. Obviously, the higher η value of co-sensitized solar cell compared with the individually N719 sensitized devices is attributed to the enhanced photovoltaic parameters Jsc and Voc.
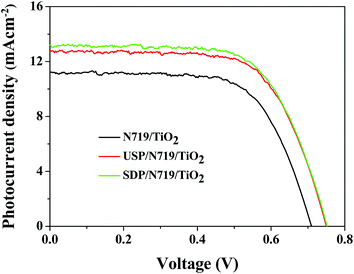 |
| Fig. 4 J–V curves for DSSCs based on different photoelectrodes under irradiation. | |
Table 2 J–V Performance of DSSCs based on different photoelectrodes
Photoelectrode |
Jsc (mA cm−2) |
Voc (V) |
FF |
η (%) |
Dye loading amount/10−7 mol cm−2 |
N719 |
USP or SDP |
Total |
N719/TiO2 |
11.22 |
0.71 |
0.66 |
5.28 |
1.20 |
— |
1.20 |
USP/N719/TiO2 |
12.73 |
0.75 |
0.67 |
6.40 |
1.19 |
1.02 |
2.21 |
SDP/N719/TiO2 |
13.19 |
0.75 |
0.66 |
6.51 |
1.19 |
0.87 |
2.06 |
It is well-known that the amount of dye loading on the photoanode can strongly affect the Jsc. The amounts of absorbed dye were measured by desorbing the dye from the dye-sensitized films in a 0.1 M NaOH solution in water and ethanol (1
:
1, v/v), and the results are listed in Table 2. As shown in Table 2, the amount of N719 dye loading for N719/TiO2 photoanodes is 1.20 × 10−7 mol cm−2, and for USP/N719/TiO2 and SDP/N719/TiO2 it is 1.19 × 10−7 and 1.19 × 10−7 mol cm−2, respectively. The nearly unchanged loading amount of N719 indicates that there was no competitive adsorption between N719 and these pyridine anchor co-adsorbents due to the site-selective adsorption behaviour of the two different dyes. This is confirmed by FT-IR spectroscopic measurement of the co-sensitized TiO2 particles. As shown in Fig. 5, the absorption band at 1695 cm−1 corresponding to the stretching vibration of the carbonyl groups of N719 disappeared, and the peak intensities of absorption bands at 1373 and 1615 cm−1 increased in the case of the N719 sensitized TiO2 particles. As reported previously, these results indicate that N719 adsorbs at the Brösted acid sites of the TiO2 surface (the sites of hydroxyl groups bound to Ti atoms) via dehydration reactions.22 In addition, characteristic absorption bands at around 1615 cm−1 were observed further increased in both co-sensitized TiO2 particles. In pyridine anchor co-adsorbents, these absorption bands are assigned to the absorption of the pyridine ring, which coordinates to the Ti atom at the TiO2 surface (at Lewis acid sites).27 These results suggest that site selective adsorption is achieved successfully using the combination of N719 and the pyridine anchor dye (USP or SDP).
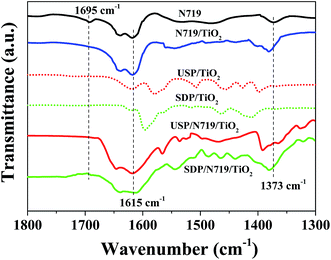 |
| Fig. 5 FT-IR spectra of the dyes adsorbed on TiO2 films. | |
The successfully co-sensitization of N719 and pyridine anchor co-adsorbent (USP or SDP) on the TiO2 surface without competitive adsorption increased the total dye loading amounts after co-sensitization (from 1.20 × 10−7 mol cm−2 to 2.21 × 10−7 and 2.06 × 10−7 mol cm−2 for USP/N719/TiO2 and SDP/N719/TiO2, respectively), which resulted in the improvement of Jsc in co-sensitized solar cells than individual N719 sensitized solar cell.
However, under the same dyeing process conditions, the amount of USP dye (1.02 × 10−7 mol cm−2) loaded on the TiO2 surface is higher than that of the SDP dye (0.87 × 10−7 mol cm−2). This might be caused by the difference in the adsorption mechanism of USP and SDP on the TiO2 surface. Because SDP has two anchoring groups, it might occupy more adsorption sites on the TiO2 surface than the USP dye. The USP co-adsorbent can be adsorbed on the TiO2 surface with a higher density than the SDP because it has mono-anchoring group. Although SDP has a lower loading amount on TiO2 surface, it exhibits much higher Jsc than USP. As discussed above, the extinction coefficient of SDP is higher than that of USP, which benefits for adsorb light and enhances Jsc. Besides, stronger electronic coupling is formed between SDP and TiO2 than between USP and TiO2 because the two linking bridges of SDP are bound to a Ti atom. The strong electronic coupling between light absorbers and TiO2 contribute to enhance the charge transfer kinetics.35 Therefore, this enhancement of electronic coupling between SDP and TiO2 is expected to lead to the injection of photo-generated electrons in SDP into the conduction band of TiO2 with high charge transfer kinetics. For further characterization, the contribution of one mole of dye to photocurrent generation current is estimated by dividing the short circuit current density (Jsc) by the amount of dye adsorbed on the TiO2 surface. It is shown that SDP provides higher levels of photo-excitation than USP, showing high value of Jsc per mol of dye. This means that individual SDP molecule can be expected to transfer larger amounts of photo-excited electrons into the conduction band of TiO2 than the USP molecule due to the enhanced electronic coupling.
The IPCE measurements of different devices were conducted to further understand the effect of pyridine anchor co-adsorbents with different number of anchoring groups on the photocurrent of DSSCs, and the spectra are collected in Fig. 6. The DSSCs containing only N719 dye has a broad IPCE spectrum from 300–750 nm but a decrease in the wavelength range of 340–450 nm, which is due to the competitive light absorption between I3− and N719. When the prepared co-adsorbent is used as co-sensitizer, not only this decrease is restored, but also the IPCE spectrum in the whole visible region is enhanced. This is attributed to the fact that the prepared co-adsorbent has attached to the TiO2 surface effectively and contributed to the electron injection into the conduction band of the TiO2. This means the co-sensitization of N719 and prepared co-adsorbent has a significant synergy and compensatory effect on light harvesting, electron injection and collection on TiO2. What's more, as shown in Fig. 6, the SDP co-sensitized solar cells generate larger photocurrents than the USP co-sensitized ones. This enhancement is caused by the good electronic coupling between SDP and TiO2, which is speculated to lead to remarkable improvement in the photovoltaic performance under the irradiation of solar light. Based on the IPCE and the absorption spectra, the cell's higher Jsc in the case of co-sensitization is mainly ascribed to better light harvesting in the low wavelength region, where the absorption of N719 is compensated and the competitive light absorption of I3− is overcome, and the good electronic coupling between pyridine anchor co-adsorbent (USP or SDP) and TiO2.
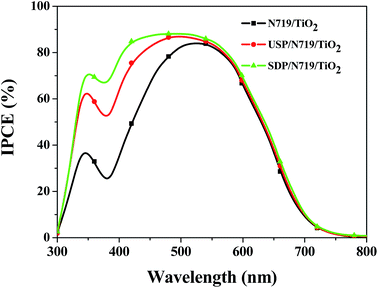 |
| Fig. 6 IPCE spectra of DSSCs based on single N719 sensitized and co-sensitized photoelectrodes. | |
Furthermore, to get the information about the electron transport mechanism in different devices, their electrochemical impedance spectra (EIS) were measured under standard AM 1.5G solar irradiation by applying a forward bias of −0.75 V. Under light illumination, EIS was utilized to analyze the electron transport resistance at the TiO2/dye/electrolyte interface for its significance on the efficiency of DSSCs.36–38 As shown in Fig. 7a, the three semicircles located in high, middle and low frequency regions (left to right) are attributed to the electrochemical reaction at the Pt/electrolyte interface, the electron transfer at the TiO2/dye/electrolyte interface and charge transfer in the electrolyte (Nernst diffusion).39–41 The radius of the large semicircle located in middle frequency regions in the Nyquist plots decrease after co-sensitized with prepared compounds, and the values are in the order of SDP/N719 < USP/N719 < N719, which indicates a decrease of the electron transfer impedance (Rct) and a increase of electron transfer rate at this interface after co-sensitization. This means that the SDP co-sensitized photoanode can provide better photovoltaic performance than the USP co-sensitized one under the same conditions.
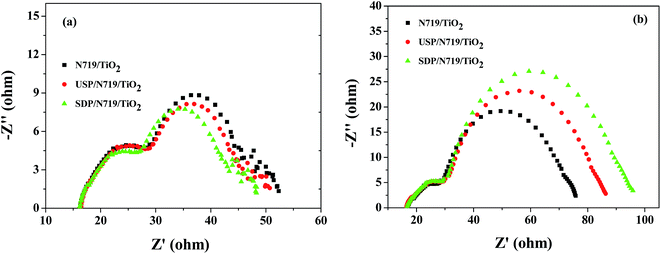 |
| Fig. 7 Nyquist plots Bode plots of EIS for DSSCs based on different photoelectrodes (a) under standard AM 1.5G solar irradiation (b) in dark. | |
In an effort to understand the enhancement of the Voc value in the co-sensitized solar cell and to investigate the kinetics of recombination processes, electrochemical impedance spectra (EIS) of devices based on different photoelectrodes were also measured in dark by applying a forward bias of −0.75 V.42,43 In the dark, the DSSCs behave as a leaking capacitor. Upon forward bias, the electrons are injected from the FTO substrate into the TiO2 and the film is charged by electron propagation through the mesoscopic TiO2 network. Meanwhile, a fraction of the injected conduction band electrons are lost by the reduction of I3− ions present in the electrolyte. Therefore, we could measure impedance spectra of DSSCs in the dark to study the electrons recombination from the conduction band of TiO2 to I3− ions. In dark conditions, as shown in Fig. 7b, the three semicircles located in high, middle and low frequency regions (left to right) of Nyquist plots are attributed to the redox reaction at the Pt counter electrode, the electron transfer at the TiO2/dye/electrolyte interface and charge transfer in the electrolyte (Nernst diffusion).44–46 Therefore the larger semicircle observed in middle frequency region represents the resistances of the charge transfer from the TiO2 to the electrolyte (back recombination resistance Rrec). The radius of this semicircle increase after co-sensitized with prepared co-adsorbents, indicating an increase of Rrec. The increased value of Rrec for DSSCs implies the retardation of the charge recombination between injected electron and I3− ions in the electrolyte, with a consequent increase of Voc. This appears to be consistent with the larger Voc values of co-sensitized cells. Due to the site-selective adsorption behaviour of the N719 and the prepared co-adsorbent, the adsorption of prepared co-adsorbent may form a better dye coverage to help to passivate the TiO2 surface or form an insulating molecular layer composed of prepared co-adsorbent and N719 molecules and thus reduces the recombination due to electron back-transfer between TiO2 and I3−.
The results mentioned above are also supported by dark current–voltage (J–V) measurements of different devices, which are presented in Fig. 8. It shows that the dark current is lower for the co-sensitized system compared with that of single N719 sensitized DSSC, which is in the order of SDP/N719 < USP/N719 < N719. The reduction of the dark current demonstrated that USP and SDP successfully suppress the electron back reaction with I3− in the electrolyte by forming a compact layer with N719, giving rise to Voc and Jsc, resulting in higher overall η value.
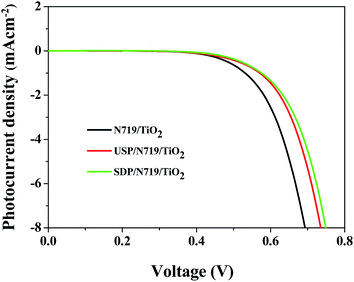 |
| Fig. 8 J–V curves for DSSCs based on co-sensitized photoelectrodes and N719 sensitized photoelectrode in dark. | |
To further probe the recombination kinetics of the devices, open-circuit voltage decay (OCVD) curves were recorded. In order to conduct the OCVD measurement, the simulated solar light is illuminated at DSSCs and a steady-state voltage was obtained. This indicated that equilibrium between electron injection and recombination is attained at the FTO surface. A potentiostat then monitors the decay of Voc after interrupting the illumination. The decay of the photovoltage reflects the decrease of the electron concentration at the FTO surface, which is mainly caused by the charge recombination. In other words, the recombination velocity of photoelectron is proportional to the response of the OCVD.47 Fig. 9a shows the OCVD decay curves of different devices. It was observed that the OCVD response of the co-sensitized DSSCs was much slower than that of individual N719 sensitized DSSCs, especially in the shorter time domain (within 15 s). The correlation between Voc decay and electron lifetime (τe) can be expressed by equation:
where
kB is the Boltzmann constant,
T is temperature, and e is the electron charge.
43,47 Therefore, the electron lifetimes can be extracted from the initial slope of
Voc decay curves.
Fig. 9b compares the results of the dependence of
τe on the open-circuit voltage for DSSCs with and without co-adsorbent. It clearly demonstrates that, at any given open-circuit potential, the electron life of the co-sensitized solar cell was longer than that of individual N719 sensitized solar cell. This suggests that the electrons injection from excited dye can survive longer and hence can facilitate electron transport without undergoing losses at the bare FTO surface. In conclusion, the OCVD measurement in
Fig. 9 demonstrated that co-sensitized with prepared co-adsorbent was able to reduce the photoelectron recombination speed effectively and prolong the lifetime of the photoelectrons. This matches well with the photovoltaic analyses of different devices.
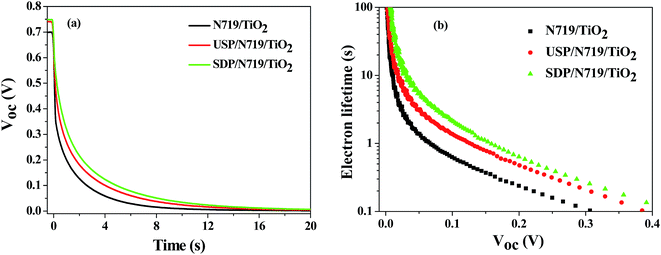 |
| Fig. 9 (a) The OCVD curves of the DSSCs with different photoelectrodes; (b) the electron lifetime as a function of Voc of the DSSCs with different photoelectrodes. | |
4. Conclusions
In conclusion, due to the site-selective adsorption behaviour of pyridine pyridine-anchor co-adsorbents and N719, each of the new synthesized pyridine-anchor co-adsorbents of pyridinecarboxaldimine substituted aminonaphthalene has a significant effect on the performance of DSSCs when it was used as co-sensitizer, it could overcome the deficiency of N719 absorption in the low wavelength region of visible spectrum, offset competitive visible light absorption of I3−, suppress the charge recombination, and prolong the electron lifetime. The co-sensitized device exhibits enhanced performance, and all of them are higher than that of single N719 sensitized solar cells. The anchoring group numbers in pyridine-anchor co-adsorbent has important effect on the performance of N719 sensitized solar cells. SDP co-adsorbent containing double anchoring groups shows better electronic coupling with TiO2 than the USP co-adsorbent with a single anchoring group, which leads SDP to exhibit better performance of DSSCs. A short circuit current density of 13.19 mA cm−2, an open circuit voltage of 0.75 V and a fill factor of 0.66 corresponding to an overall conversion efficiency of 6.51% under AM 1.5G solar irradiation are achieved when SDP is used as co-adsorbent, which is much higher than that for DSSCs only sensitized by N719 (5.28%) under the same condition. Further studies to improve the DSSCs performance through co-sensitization method using Lewis acid sites on the TiO2 surface with pyridine-anchor co-adsorbent containing more anchoring groups are currently underway.
Acknowledgements
This work was supported by National Natural Science Foundation of China (Grant 21371040, 21571042 and 21171044), the National Key Basic Research Program of China (973 Program, No. 2013CB632900).
References
- B. O'Regan and M. Grätzel, Nature, 1991, 353, 737–740 CrossRef.
- A. Hagfeldt and M. Grätzel, Acc. Chem. Res., 2000, 33, 269–277 CrossRef CAS PubMed.
- P. J. Cameron and L. M. Peter, J. Phys. Chem. B, 2003, 107, 14394–14400 CrossRef CAS.
- J. Bisquert, D. Cahen, G. Hodes, S. Rühle and A. Zaban, J. Phys. Chem. B, 2004, 108, 8106–8118 CrossRef CAS.
- M. K. Nazeeruddin, F. D. Angelis, S. Fantacci, A. Selloni, G. Viscardi, P. Liska, S. Ito, B. Takeru and M. Grätzel, J. Am. Chem. Soc., 2005, 127, 16835–16847 CrossRef CAS PubMed.
- K. Guo, M. Li, X. Fang, M. Luoshan, L. Bai and X. Zhao, J. Power Sources, 2014, 249, 72–78 CrossRef CAS.
- J. Chang, C. P. Lee, D. Kumar, P. W. Chen, L. Y. Lin, K. R. J. Thomas and K. C. Ho, J. Power Sources, 2013, 240, 779–785 CrossRef CAS.
- L. Y. Lin, M. H. Yeh, C. P. Lee, J. Chang, A. Baheti, R. Vittal, K. R. J. Thomas and K. C. Ho, J. Power Sources, 2013, 247, 906–914 CrossRef.
- F. Gao, Y. Wang, D. Shi, J. Zhang, M. K. Wang, X. Y. Jing, R. Humphry-Baker, P. Wang, S. M. Zakeeruddin and M. Grätzel, J. Am. Chem. Soc., 2008, 130, 10720–10728 CrossRef CAS PubMed.
- M. K. Nazeeruddin, S. M. Zakeeruddin, R. Humphry-Baker, M. Jirousek, P. Liska, N. Vlachopoulos, V. Shklover, C. H. Fischer and M. Grätzel, Inorg. Chem., 1999, 38, 6298–6305 CrossRef CAS PubMed.
- A. Yella, H. W. Lee, H. N. Tsao, C. Y. Yi, A. K. Chandiran, M. K. Nazeeruddin, E. W. G. Diau, C. Y. Yeh, S. M. Zakeeruddin and M. Grätzel, Science, 2011, 334, 629–634 CrossRef CAS PubMed.
- L. Y. Han, A. Islam, H. Chen, C. Malapaka, B. Chiranjeevi, S. F. Zhang, X. D. Yang and M. Yanagida, Energy Environ. Sci., 2012, 5, 6057–6060 CAS.
- H. Ozawa, R. Shimizu and H. Arakawa, RSC Adv., 2012, 2, 3198–3200 RSC.
- M. Mojiri-Foroushani, H. Dehghani and N. Salehi-Vanani, Electrochim. Acta, 2013, 92, 315–322 CrossRef CAS.
- P. J. Holliman, M. Mohsen, A. Connell, M. L. Davies, K. Al-Salihi, M. B. Pitak, G. J. Tizzard, S. J. Coles, R. W. Harrington, W. Clegg, C. Serpa, O. H. Fontes, C. Charbonneau and M. J. Carnie, J. Mater. Chem., 2012, 22, 13318–13327 RSC.
- K. M. Lee, Y. C. Hsu, M. Ikegami, T. Miyasaka, K. R. J. Thomas, J. T. Lin and K. C. Ho, J. Power Sources, 2011, 196, 2416–2421 CrossRef CAS.
- S. Q. Fan, C. Kim, B. Fang, K. X. Liao, G. J. Yang, C. J. Li, J. J. Kim and J. Ko, J. Phys. Chem. C, 2011, 115, 7747–7754 CAS.
- G. D. Sharma, D. Daphnomili, K. S. V. Gupta, T. Gayathri, S. P. Singh, P. A. Angaridis, T. N. Kitsopoulos, D. Tasis and A. G. Coutsolelos, RSC Adv., 2013, 3, 22412–22420 RSC.
- Z. Wu, Y. Wei, Z. An, X. Chen and P. Chen, Bull. Korean Chem. Soc., 2014, 35, 1449–1452 CrossRef CAS.
- S. Chang, H. Wang, L. T. L. Lee, S. Zheng, Q. Li, K. Y. Wong, W. K. Wong, X. Zhu, W. Y. Wong, X. Xiao and T. Chen, J. Mater. Chem. C, 2014, 2, 3521–3526 RSC.
- K. Sayama, S. Tsukagoshi, T. Mori, K. Hara, Y. Ohga, A. Shinpou, Y. Abe, S. Suga and H. Arakawa, Sol. Energy Mater. Sol. Cells, 2003, 80, 47–71 CrossRef CAS.
- Z. S. Wang, K. Hara, Y. Don-oh, C. Kasada, A. Shinpo, S. Suga, H. Arakawa and H. Sugihara, J. Phys. Chem. B, 2005, 109, 3907–3914 CrossRef CAS PubMed.
- L. G. Wei, Y. L. Yang, R. Q. Fan, P. Wang, L. Li, J. Yu, B. Yang and W. W. Cao, RSC Adv., 2013, 3, 25908–25916 RSC.
- L. G. Wei, Y. L. Yang, R. Q. Fan, Y. Na, P. Wang, Y. W. Dong, B. Yang and W. W. Cao, Dalton Trans., 2014, 43, 11361–11370 RSC.
- Y. Harima, T. Fujita, Y. Kano, I. Imae, K. Komaguchi, Y. Ooyama and J. Ohshita, J. Phys. Chem. C, 2013, 117, 16364–16370 CAS.
- Y. Ooyama, S. Inoue, T. Nagano, K. Kushimoto, J. Ohshita, I. Imae, K. Komaguchi and Y. Harima, Angew. Chem., Int. Ed., 2011, 50, 7429–7433 CrossRef CAS PubMed.
- M. W. Urban, Vibrational Spectroscopy of Molecules and Macromolecules on Surfaces, Wiley, Chichester, 1993, pp. 171–185 Search PubMed.
- P. Y. Reddy, L. Giribabu, C. Lyness, H. J. Snaith, C. Vijaykumar, M. Chandrasekharam, M. Lakshmikantam, J. H. Yum, K. Kalyanasundaram, M. Grätzel and M. K. Nazeeruddin, Angew. Chem., Int. Ed., 2007, 46, 373–376 CrossRef CAS PubMed.
- D. B. Kunag, S. Ito, B. Wenger, C. Klein, J. E. Moser, R. Humphry-Baker, S. M. Zakeeruddin and M. Grätzel, J. Am. Chem. Soc., 2006, 128, 4146–4154 CrossRef PubMed.
- G. D. Sharma, S. P. Singh, R. Kurchania and R. J. Ball, RSC Adv., 2013, 3, 6036–6043 RSC.
- C. Y. Lin, C. F. Lo, L. Luo, H. P. Lu, C. S. Hung and E. W. G. Diau, J. Phys. Chem. C, 2009, 113, 755–764 CAS.
- C. M. Cardona, W. Li, A. E. Kaifer, D. Stockdale and G. C. Bazan, Adv. Mater., 2011, 23, 2367–2371 CrossRef CAS PubMed.
- G. Boschloo, L. Halggman and A. Hagfeldt, J. Phys. Chem. B, 2006, 110, 13144–13150 CrossRef CAS PubMed.
- K. R. Justin Thomas, Y. C. Hsu, J. T. Lin, K. M. Lee, K. C. Ho, C. H. Lai, Y. M. Cheng and P. T. Chou, Chem. Mater., 2008, 20, 1830–1840 CrossRef.
- H. J. Yun, T. Paik, M. E. Edley, J. B. Baxter and C. B. Murray, ACS Appl. Mater. Interfaces, 2014, 6, 3721–3728 CAS.
- C. P. Hsu, K. M. Lee, J. T. W. Huang, C. Y. Lin, C. H. Lee, L. P. Wang, S. Y. Tsai and K. C. Ho, Electrochim. Acta, 2008, 53, 7514–7522 CrossRef CAS.
- K. E. Lee, M. A. Gomez, C. Charbonneau and G. P. Demopoulos, Electrochim. Acta, 2012, 67, 208–215 CrossRef CAS.
- C. Y. Hsu, W. T. Chen, Y. C. Chen, H. Y. Wei, Y. S. Yen, K. C. Huang, K. C. Ho, C. W. Chu and J. T. Lin, Electrochim. Acta, 2012, 66, 210–215 CrossRef CAS.
- J. Bisquert, Phys. Chem. Chem. Phys., 2003, 5, 5360–5364 RSC.
- D. Kuang, S. Uchida, R. Humphry-Baker, S. M. Zakeeruddin and M. Grätzel, Angew. Chem., Int. Ed., 2008, 47, 1923–1926 CrossRef CAS PubMed.
- N. Koide, A. Islam, Y. Chiba and L. Y. Han, J. Photochem. Photobiol., A, 2006, 182, 296–303 CrossRef CAS.
- J. Bisquert, J. Phys. Chem. B, 2002, 106, 325–333 CrossRef CAS.
- J. Bisquert, A. Zaban, M. Greenshtein and I. Mora-Sero, J. Am. Chem. Soc., 2004, 126, 13550–13559 CrossRef CAS PubMed.
- J. Bisquert, Phys. Chem. Chem. Phys., 2003, 5, 5360–5364 RSC.
- D. Kuang, S. Uchida, R. Humphry-Baker, S. M. Zakeeruddin and M. Grätzel, Angew. Chem., Int. Ed., 2008, 47, 1923–1926 CrossRef CAS PubMed.
- N. Koide, A. Islam, Y. Chiba and L. Y. Han, J. Photochem. Photobiol., A, 2006, 182, 296–303 CrossRef CAS.
- A. Zaban, M. Greenshtein and J. Bisquert, ChemPhysChem, 2003, 4, 859–864 CrossRef CAS PubMed.
Footnote |
† Electronic supplementary information (ESI) available. See DOI: 10.1039/c6ra06017j |
|
This journal is © The Royal Society of Chemistry 2016 |
Click here to see how this site uses Cookies. View our privacy policy here.