DOI:
10.1039/C6RA05906F
(Paper)
RSC Adv., 2016,
6, 55463-55471
Partial oxidation of ethylbenzene by H2O2 on VOx/HZSM-22 catalyst†
Received
5th March 2016
, Accepted 30th May 2016
First published on 31st May 2016
Abstract
A series of VOx/HZSM-22 catalysts for the partial oxidation of ethylbenzene was prepared with 0.09–2.86 wt% V loading by an incipient wetness impregnation method, and the catalysts were characterized by inductively coupled plasma-atomic emission spectrometer, X-ray diffraction, Fourier transform infrared spectroscopy, N2 physisorption analysis, X-ray photoelectron spectroscopy, diffuse reflectance ultraviolet-visible spectroscopy, temperature-programmed calcination-mass spectroscopy, and temperature-programmed hydrogen reduction. The results showed that the chemical nature of vanadium species on HZSM-22 depended on V loading. The amount of vanadium species incorporated into the framework of HZSM-22 increased with V loading firstly and reached a relatively stable value of about 0.2 wt%. The decomposed ammonia might reduce V5+ to V4+ in the calcination process. Highly dispersed extra-framework vanadium species, especially surface extra-framework V4+, promised a good effect on the oxidation of ethylbenzene, under optimized conditions, a high yield of 17.5% with 72.5% selectivity to acetophenone was obtained.
1. Introduction
Traditionally, the production of aromatic ketones was primarily achieved by the reaction of Friedel–Crafts acylation utilizing acid halides or acid anhydrides as reactants employing a stoichiometric amount of AlCl3 as catalyst, which led to a large volume of environmentally hazardous and corrosive waste.1–3 Consequently, great efforts have been devoted to producing the phenyl ketones from the oxidation of alkylbenzenes through a more efficient and environmentally friendly method in the past few decades.4–9 It is of profound significance to find more sustainable methods using friendly oxidants such as H2O2 and recyclable catalysts to obtain the aromatic ketones because conventional procedures bear some drawbacks, such as multiple-step reactions, corrosion of equipment, harsh process conditions, unfriendly oxidants etc.10,11 Acetophenone is regarded as an important intermediate in the production of agrochemicals, perfumes, esters, pharmaceuticals, drugs, tear gas and resins.12–15 A great deal of work associated with this one-pot reaction has been done to obtain good activity and selectivity, including homogeneous catalysts and heterogeneous catalysts.12,16–20
Heterogeneous catalysts for the oxidation of ethylbenzene were highly expected. Typically, Chuan-De Wu and his coworkers incorporated metal 5,10,15,20-tetrakis(3,5-biscarboxylphenyl)porphyrin (M-H8OCPP) into porous metal–organic framework, and this catalyst gave out more than 99% conversion of ethylbenzene and selectivity to the acetophenone.21 M. Ghiaci and his co-workers used SiO2/Al2O3-supported manganese catalyst and obtained a conversion of 91% and a selectivity of 98% in the presence of supercritical carbon dioxide with good catalyst stability.22 V. Murugesan et al. synthesized bimetallic Mn–Ti-SBA-15 and got a ethylbenzene conversion of 92% with 87% selectivity to acetophenone.23 However, these studies used tert-butyl hydroperoxide as the oxidant, which is expensive, poisonous and explosive at high temperature.
Therefore, using H2O2 or O2 as oxidant is the present focus in the oxidation of ethylbenzene. In this aspect, homogeneous catalysts were mostly used. Bernhard Gutmann et al. reported that the oxidation of ethylbenzene catalyzed by CoBr2 in acetic acid obtained 87% conversion of ethylbenzene and 36% selectivity to the acetophenone at 80 °C.20 Zaihui Fu et al. employed 8-quinolinolato manganese(III) complexes to get 26.1% ethylbenzene conversion and 65% oxidation yield.12 These processes generally suffered from the separation of reaction mixture and the difficulty of the reuse of the catalyst.
Thus, developing heterogeneous catalyst using H2O2 as oxidant under mild reaction conditions to realize the target partial oxidation is highly desired. The pioneer work of Mona Hosseini-Sarvari et al.24 and Rajaram Bal et al.25 stimulated us to exploit broader kinds of heterogeneous catalysts using H2O2 as oxidant.
Zeolites is the most abundant nanoporous materials with great pore channels and surface area, the mostly famous being ZSM-5, silicalite-1, zeolite beta, zeolites A, X and Y.26 In order to modulate the performance on different kinds of reactions of the zeolites, the incorporation of transition metals to the extra-framework and framework is crucial for the catalytic activity for the targeted product.
Supported vanadium oxides catalysts have been widely studied for catalyzing different reactions.27–31 These vanadium oxides catalysts favored to the side-chain oxidation of ethylbenzene.32,33 The vanadium species incorporated into the framework of SBA-16 was mainly in the form of highly dispersed tetrahedral coordination.32 The highly dispersed tetrahedral V
O species with lower vanadium loading are associated with the conversion of oxidation activity and the formation of Ce–O–Ce and the V–O–V structure are related to the production of acetophenone.33 Centi et al. reported a polynuclear vanadium species with three different states (V3+, V4+ and V5+) and an octahedral VO2+ prefers to interact with the OH groups, also a symmetrical framework tetrahedral V5+ species on VS-1 via direct hydrothermal method.34
Some papers reported that HZSM-22 zeolite has TON structure type and three different kinds of framework topology rings, including 5, 6 and 10-membered-ring structures, which are smaller than ZSM-5, ZSM-11, and ZSM-35, and they also have acidic properties and shape selectivity on the surface of zeolites.35–37 Besides, impregnation method is useful to gain a high percentage of metal loading.38
In the present work, a series of VOx/HZSM-22 catalysts was synthesized and used to catalyze the ethylbenzene to acetophenone using hydrogen peroxide as oxidant. To gain a further insight into the nature of the vanadium species as well as the connection between vanadium species and the catalytic performance on ethylbenzene, we studied the correlation with conversion of ethylbenzene, yield of products as well as selectivity of products and the structure of vanadium species in the HZSM-22.
2. Experimental
2.1. Catalyst preparation
VOx/HZSM-22 catalyst was prepared by incipient wetness impregnation method using commercially available HZSM-22 as support, which was calcined at 600 °C for 4 h before impregnation. Firstly, the HZSM-22 support was evacuated to 0.8–0.9 Torr for 0.5 h in a 100 mL test tube with side neck. Then aqueous solution of NH4VO3 was dropped onto the HZSM-22 support, and the slurry was stood for 24 h at room temperature. The excess H2O was removed by heating the tube in a water bath at 80 °C, and the resultant solid was dried overnight in electric oven with forced convection at 100 °C, and then calcined at 550 °C for 6 h in air to obtain the catalysts, denoted as x wt% support (x was the controlled V content).
Temperature-programmed calcination of the impregnated sample was also performed using multichannel Hiden HP R-20QIC mass spectrometer to monitor the gaseous products formed. Firstly, 100 mg sample was added into a stainless steel U-tube. Then the sample was swept using helium at room temperature for nearly 2 h until the baseline of MS signals stabilized. After that, the temperature was raised to 550 °C with a ramp of 5 °C min−1 in flow air (20 mL min−1). The signals of exhausted gas were monitored and a quantity of ammonia, a small amount of nitrous oxide and nitric oxide with MS signals of m/z = 17, 44 and 30 were observed. The evolution of the NOx demonstrated the oxidation of ammonia, probably by V5+ species, which was reduced to V4+.
2.2. Catalyst characterization
The actual V content loaded on the catalysts was determined by Inductively Coupled Plasma-Atomic Emission Spectrometer (ICP-AES) (IRIS Advantage ER/S).
X-ray diffraction (XRD) measurement of the catalysts were performed on a SHIMAZU XRD-6100 diffractometer using Cu-kα radiation (λ = 0.15406 Å) with a scanning angle (2θ) from 5 to 80°. The tube voltage was 40 kV and the current was 30 mA.
Fourier transform infrared (FTIR) spectra were collected at 2 cm−1 resolution using a Nicolet Nexus 670 FTIR spectrometer equipped with a mercury cadmium telluride detector. The powder samples were mixed with KBr (1 wt%) and pressed into translucent disks at room temperature.
The surface area, pore volume, and average pore size distribution were measured by N2 physisorption analysis at liquid nitrogen temperature (77 K) using a Quantachrome Autosorb-iQ instrument. The samples were degassed at 573 K for 4 h before adsorption measurement. The surface area was calculated by BET equation with a Micropore BET Assistant, and the micropore surface area and micropore volume were determined by V–t method. Moreover, the pore size distribution was calculated by Saito–Foley method.
X-ray Photoelectron spectroscopy (XPS) was used to determine the surface species and their composition on the samples. XPS experiments were carried on an AXIS Ultra DLD (KRATOS) high performance electron spectrometer with Al Kα radiation (1486.6 eV) and the source being operated at 12 kV and 12 mA. The obtained binding energy (BE) was calibrated using the C 1s peak at 284.6 eV as the reference, and the linear background was subtracted from all the spectra and peak fitting was performed using an 80/20 Lorentz–Gauss function.
Diffuse reflectance ultraviolet-visible spectroscopy (DR UV-vis spectroscopy) spectra was obtained on a SHIMADZU UV-3600 in the range from 190 to 850 nm using BaSO4 as the reference. The spectra were deconvoluted into sub-bands by Peakfit v4 software.
Temperature-programmed hydrogen reduction (H2-TPR) was utilized to characterize the samples with different vanadium loading. The sample (100 mg) was placed in a fixed-bed reactor and pretreated at 423 K for 30 min in N2 with a flow rate of 30 mL min−1. After the temperature was decreased to 353 K, the sample was heated from 353 K to 1103 K with a ramp of 5 K min−1 in 5% H2/Ar flow. The consumption of H2 was recorded by a GC (FULI-9790) equipped with a thermal conductivity detector (TCD).
2.3. Measurement of catalytic activity
Partial oxidation of ethylbenzene (EB) was carried out in a 50 mL two-necked round flask equipped with a reflux condenser and a stirrer in a water bath. 8.2 mmol of substrate was added into acetonitrile solvent with the designed amount of catalyst. When the water bath temperature reached 70 °C and kept constant, hydrogen peroxide (HP) aqueous solution (30 wt%) was added to the reaction flask. The resulting mixture was refluxed for designed time, and then cooled to room temperature. After filtration of the reaction mixture, the liquid products were identified by GC-MS analyses (Agilent, 5973 NETWORK 6890 N), and analyzed quantitatively by GC (FULI, GC-9790 equipped with hydrogen flame detector and a capillary column AT, SE-54) using nitrobenzene as internal standard. The products included acetophenone (AP), benzaldehyde (BD), 1-phenylethanol (1-PE), 2-phenylethanol, ethylphenols and 2-ethylcyclohexa-2,5-diene-1,4-dione (EQ). The yields were calculated by the amount of product (mmol) over the amount of initial ethylbenzene (mmol) added. The selectivity was calculated by the amount of product (mmol) over the amount of all the products (mmol), ignoring the ring-opening products, tar and polycondensed aromatic hydrocarbons because no such products were observed, avoiding the interference of volatility of ethylbenzene.39 The reaction scheme of ethylbenzene is in Scheme S1 (see ESI†). The conversion (conv.) of EB is the sum of all yields of the products. The yield (Yi, i = AP, BD, 1-PE, 2-PE, ethylphenols and EQ) of products, the selectivity (Si, i = AP, BD, 1-PE, 2-PE, ethylphenols, EQ, side-chain and ring) are calculated as follows:
3. Results and discussion
3.1. Catalyst characterization
3.1.1. ICP-AES. The results of ICP-AES are displayed in Table 1. Ammonium acetate can dissolve the extra-framework vanadium species while aqua regia can dissolve both the extra-framework and framework vanadium species.40,41 Thus, vanadium content obtained from aqua regia dissolution might represent the actual total vanadium content, while that from ammonium acetate dissolution may represent the content of the extra-framework vanadium species. With the controlled vanadium contents increasing from 0.10 to 3.50 wt%, the total actual content of vanadium on the support rose monotonically from 0.09 to 2.86 wt%. Similarly, the content of extra-framework vanadium increased from 0.07 to 2.08 wt%. At low controlled vanadium loading of 0.10 and 0.50 wt%, only small amount of vanadium (0.02 and 0.04 wt% respectively) could be incorporated into the framework of HZSM-22. With the controlled vanadium varied from 1.00 to 2.50 wt%, the amount of framework vanadium kept almost the same (about 0.20 wt%). While further increase of controlled vanadium content made a significant increase of both extra-framework and framework vanadium species. It was indicated that the distribution of vanadium species varies with the controlled amount of vanadium in the preparation process, which dominated the amount of vanadium incorporated in the framework of HZSM-22 and that of extra-framework. In the preparation of vanadium silicate-1 catalyst, a threshold value of about 1.0 wt% vanadium was found to be favored to incorporate into the framework of silicate-1.40 The 0.20 wt% vanadium incorporation over the range of 1.00 to 2.50 wt% controlled vanadium content is similar to the previous work.40 However, with higher controlled amount of vanadium, the amount of incorporated vanadium greatly increased. This might be caused by the nature of the support.
Table 1 The distribution of vanadium species on VOx/HZSM-22 depending on the controlled loading
Entry |
Vanadium content (wt%) |
Controlled |
Total |
Extra-framework |
Framework |
1 |
0.10 |
0.09 |
0.07 |
0.02 |
2 |
0.50 |
0.36 |
0.32 |
0.04 |
3 |
1.00 |
0.71 |
0.52 |
0.19 |
4 |
1.50 |
0.96 |
0.75 |
0.21 |
5 |
2.50 |
1.81 |
1.59 |
0.22 |
6 |
3.50 |
2.86 |
2.08 |
0.78 |
3.1.2. XRD. Fig. 1 shows the XRD patterns of the fresh catalysts with different V loading. The diffraction patterns of the samples with actual vanadium loading of 0.09–0.96 wt% exhibited no significant difference from those of HZSM-22, indicating that the vanadium species were highly dispersed because of the low loading. However, crystalline V2O5 with diffraction peaks at around 15.4°, 21.6° based on the literature42–44 was observed when the vanadium content increased from 1.81 to 2.86 wt%. It is indicated that higher loading led to the aggregation of vanadium species on HZSM-22. The formation of crystalline V2O5 with increasing vanadium content was also observed on mesoporous SBA-15, and the critical content was found to be about 2.8 wt%, which was higher than the value observed in the present work (1.81 wt%).45 The different properties of the supports might be the reason for this variation.
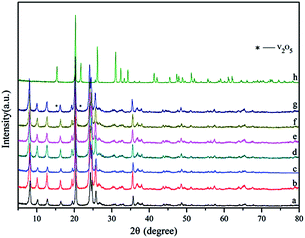 |
| Fig. 1 The XRD patterns of the fresh catalysts with different V loading and pure vanadium pentoxide. (a) HZSM-22, (b) 0.09 wt% VOx/HZSM-22, (c) 0.36 wt% VOx/HZSM-22, (d) 0.71 wt% VOx/HZSM-22, (e) 0.96 wt% VOx/HZSM-22, (f) 1.81 wt% VOx/HZSM-22, (g) 2.86 wt% VOx/HZSM-22, (h) V2O5. | |
3.1.3. FTIR. The FTIR spectra of the sample are shown in the Fig. 2 and S1.† The bands at about 550 cm−1 and 640 cm−1 were attributed to the bending vibration of the framework Si–O–Si bond of HZSM-22, while the bands at around 1090 cm−1 were assigned to asymmetric stretching vibration of Si–O–Si.46,47 The bands at around 1200 cm−1 were ascribed to Al–O bond, and the shifts of these bands to higher wavenumbers were indicative of vanadium species incorporated into framework of HZSM-22.48,49 There were no significant changes in FTIR with low actual vanadium loading of 0.09–0.71 wt% compared to HZSM-22. Over the vanadium loading range of 0.96–1.81 wt%, the intensity of the bands at 550, 640 cm−1 increased with increasing vanadium loading, while with the vanadium loading increasing to 2.86 wt%, the intensities of these bands had a further increase. Moreover, the band at around 1090 cm−1 had a blue shift. It might be deduced that the vanadium species that is incorporated into framework vanadium species affected significantly the Si–O–Si bond of HZSM-22.
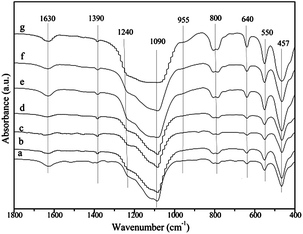 |
| Fig. 2 FTIR spectra of samples with different vanadium content: (a) HZSM-22, (b) 0.09 wt% VOx/HZSM-22, (c) 0.36 wt% VOx/HZSM-22, (d) 0.71 wt% VOx/HZSM-22, (e) 0.96 wt% VOx/HZSM-22, (f) 1.81 wt% VOx/HZSM-22, (g) 2.86 wt% VOx/HZSM-22. | |
3.1.4. N2 physisorption analysis. The N2 adsorption–desorption isotherms and the textural properties of VOx/HZSM-22 are displayed in Table 2, Fig. S2 and S3.† As the vanadium content increased from 0.09 to 2.81 wt%, the BET surface area, micropore surface area and micropore volume decreased significantly from 255.7 to 63.6 m2 g−1, 196.7 to 16.2 m2 g−1 and 0.079 to 0.008 cm3 g−1, respectively, because of the presence of framework and extra-framework vanadium species. Nevertheless, the external surface area only changed in the range of 41.3–67.9 m2 g−1. At low vanadium content of 0.09 wt%, the changes of BET surface area, micropore surface area and micropore volume was not obvious. However, with increasing vanadium content, the surface area and the pore volume decreased significantly. The slight decrease of BET surface area and pore volume with 0.09 wt% vanadium content might be caused by the incorporation of V into the framework and/or the anchor of vanadium on the wall of the pores of HZSM-22. With increase of vanadium content, a certain amount of vanadium species blocked the micropores of the catalysts, causing dramatic drop of surface area and pore volume. The formation of crystalline V2O5 species made further decrease of these properties, which was similar to the results reported in literature.50 The shift of largest pore size to bigger value with increasing vanadium from 0.09 to 2.86 wt% confirmed the above deduction.
Table 2 Physicochemical properties of the catalysts
Samples |
SBET (m2 g−1) |
Smic (m2 g−1) |
Sext (m2 g−1) |
Vmic (cm3 g−1) |
HZSM-22 |
255.7 |
196.7 |
59.0 |
0.079 |
0.09 |
238.4 |
180.3 |
58.1 |
0.074 |
0.36 |
178.3 |
137.0 |
41.3 |
0.055 |
0.71 |
167.0 |
99.1 |
67.9 |
0.041 |
0.96 |
165.4 |
105.5 |
59.9 |
0.043 |
1.81 |
149.2 |
90.0 |
59.3 |
0.037 |
2.86 |
63.6 |
16.2 |
47.3 |
0.008 |
3.1.5. XPS. The XPS results are given in the Table 3 and Fig. S4.† Clearly, as the content of actual vanadium increased from 0.09 to 2.86 wt%, the surface vanadium content increased monotonically. All the peaks at around 517.0 eV were fitted into two peaks. The former one around 517.5 eV was ascribed to the V5+, while the latter one around 516.5 eV was assigned to V4+.40,51 The presence of V4+ suggested that small quantities of framework and extra-framework V5+ were reduced by the ammonia in the calcination process, which was confirmed by the results of mass spectroscopy. The V5+/V4+ decreased monotonically with increasing vanadium content. The co-existence of V4+ and V5+ agreed with citations,52–54 and the balance between the reduction of V5+ to V4+ by NH3 released and the oxidation of V4+ to V5+ by air deserved further investigation.
Table 3 Surface compositions of VOx/HZSM-22 determined by XPS
Catalyst (wt%) |
V 2p3/2 (V) |
V 2p3/2 (IV) |
V 2p3/2 (V)/V 2p3/2 (IV) |
BE (eV) |
Area (%) |
FWHM |
BE (eV) |
Area (%) |
FWHM |
0.09 |
517.5 |
88.49 |
1.4 |
516.5 |
11.51 |
0.8 |
7.7 |
0.36 |
517.5 |
87.30 |
1.4 |
516.5 |
12.70 |
0.8 |
6.9 |
0.71 |
517.5 |
86.94 |
1.5 |
516.5 |
13.06 |
1.0 |
6.7 |
0.96 |
517.4 |
73.65 |
1.6 |
516.4 |
26.35 |
1.2 |
2.8 |
1.81 |
517.5 |
70.45 |
1.6 |
516.2 |
29.55 |
1.2 |
2.4 |
2.86 |
517.6 |
69.23 |
1.6 |
516.5 |
30.77 |
1.2 |
2.2 |
3.1.6. DR UV-vis spectroscopy. Fig. S5† displays the DR UV-vis spectroscopy spectra of all the catalysts. According to the literature,3,55,56 the bands centered at around 220 nm were assigned to V4+ characteristic of tetrahedral coordination, those at around 250–260 nm to framework V5+ with tetrahedral coordination, while those at around 380 nm to V5+ with either pentagonal or pseudo-octahedral coordination, due to interaction with water molecules.57 As shown in Fig. S5,† all the catalyst samples give DR UV-vis reflection spectra over the range of 190–850 nm and exhibited strong low charge-transfer (CT) bands below 500 nm. To study them in detail, the spectra were fitted into various sub-bands, as shown in Fig. S6,† and the quantitative results obtained from the Peakfit v4 are shown in Table 4 and the peak area of the three types of peaks at around 220, 250 and 380 nm were denoted as S1, S2 and S3, respectively. The intensity of all the peaks increase consistently as the vanadium content increasing, but the relative intensity of different sub band varied in different ways. With the increase of vanadium loading, the peak area at around 220 nm increased monotonically, which might represent the increase of V4+ incorporated into the framework of the HZSM-22,55 that is, with low vanadium loading of 0.09–0.96 wt%, the V4+ with tetrahedral coordination increased monotonically. With further increase of vanadium loading from 1.81 to 2.86 wt%, the V4+ with tetrahedral coordination kept almost the same. A slight red shift from vanadium content of 0.09–2.86 wt% was also observed, which may be assigned to the longer bond length of V–O than that of Si–O.58 The bands at around 250 nm may represent the framework V5+.59 Over the vanadium content tested, the amount of framework V5+ increased monotonically. Moreover, the ratio of S1/S2 decreased monotonically, indicating that the V4+ may decrease while the framework V5+ may increase monotonically. With the vanadium content of 0.09–2.86 wt%, the bands at around 380 nm representing V5+ with either pentagonal or pseudo-octahedral coordination increased gradually. There was a further dramatic increase as the vanadium loading increased from 1.81 to 2.86 wt%,57 and the color of all the calcined VOx/HZSM-22 transformed from white to yellowish when they were exposed to the atmosphere at room temperature, intensifying with the increase of vanadium content.60 This could be resulted from the additional coordination of water molecules to form octahedral coordinated vanadium ions in the microporous defect sites.61 The bands at approximately from 600 to 800 nm were corresponded to the d–d transition of isolated vanadyl VO2+ ions with tetrahedral coordination, but their intensity was very low compared with the major bands.56,62–64
Table 4 Quantitative results of the DR UV-vis sub-bands
Catalyst (wt%) |
S1 |
S2 |
S3 |
S1/S2 |
0.09 |
4.6 |
4.5 |
0.0 |
1.0 |
0.36 |
7.7 |
9.9 |
8.3 |
0.8 |
0.71 |
17.9 |
29.7 |
39.1 |
0.6 |
0.96 |
25.5 |
39.3 |
40.6 |
0.6 |
1.81 |
28.1 |
67.5 |
84.8 |
0.4 |
2.86 |
28.4 |
84.3 |
126.4 |
0.3 |
3.1.7. H2-TPR. The TPR results are presented in Fig. 3. Obviously, we can find three peaks at approximately 658–677 K, 760–780 K and 890 K, which might be assigned to isolated vanadium species, polymeric vanadium species and bulk-like V2O5, respectively.65–67 As the content of vanadium increased from 0.09 to 2.86 wt%, the first peak shifted slightly to higher temperature with slight increase of intensity, because of the increase of isolated vanadium species. Additionally, the second peak changed in a similar way, but the intensity for the samples with vanadium content of 2.86 wt% increased sharply compared with the other catalysts with vanadium loading 0.09–1.81 wt%, meaning that a large quantity of polymerized vanadium species formed. The third peak only occurred on the sample containing 2.86 wt%, indicating that the bulk-like V2O5 was produced, which is in accordance with the XRD results.
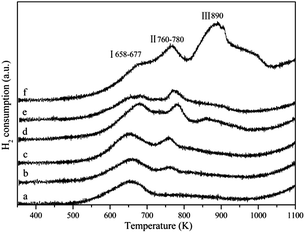 |
| Fig. 3 H2-TPR of all the catalysts: (a) 0.09 wt% VOx/HZSM-22, (b) 0.36 wt% VOx/HZSM-22, (c) 0.71 wt% VOx/HZSM-22, (d) 0.96 wt% VOx/HZSM-22, (e) 1.81 wt% VOx/HZSM-22, (f) 2.86 wt% VOx/HZSM-22. | |
3.2. Catalytic oxidation of ethylbenzene
The results of activity test in terms of ethylbenzene conversion, acetophenone yield, and selectivity to different products are shown in Tables 5–9.
Table 5 Effect of V loading on the oxidation of EBa
Catalyst (wt%) |
Conv. (%) |
SAP (%) |
Sside-chain (%) |
Sring (%) |
YAP (%) |
YBD (%) |
Y1-PE (%) |
YEQ (%) |
Yethylphenols (%) |
Reaction condition: 200 mg of VOx/HZSM-22, 70 °C, 4 h, 49.9 mmol 30% H2O2, 8.2 mmol EB, 10 mL acetonitrile. |
HZSM-22 |
0.8 |
63.3 |
100.0 |
0.0 |
0.5 |
0.2 |
0.1 |
0.0 |
0.0 |
0.09 |
0.6 |
50.0 |
83.3 |
16.7 |
0.3 |
0.1 |
0.1 |
— |
0.1 |
0.36 |
5.5 |
52.7 |
83.6 |
16.4 |
2.9 |
0.6 |
1.1 |
0.1 |
0.8 |
0.71 |
15.0 |
64.7 |
90.0 |
10.0 |
9.7 |
1.8 |
1.9 |
0.6 |
0.9 |
0.96 |
19.3 |
72.5 |
94.3 |
5.7 |
14.0 |
2.1 |
2.0 |
0.3 |
0.8 |
1.81 |
24.4 |
71.7 |
92.6 |
7.4 |
17.5 |
2.3 |
2.7 |
0.1 |
1.7 |
2.86 |
17.8 |
71.3 |
94.4 |
5.6 |
12.7 |
2.0 |
2.0 |
0.1 |
0.9 |
Table 6 Effect of amount of catalysts on the oxidation of EBa
Catalyst (mg) |
Conv. (%) |
SAP (%) |
Sside-chain (%) |
Sring (%) |
YAP (%) |
YBD (%) |
Y1-PE (%) |
YEQ (%) |
Yethylphenols (%) |
Reaction condition: 1.81 wt% VOx/HZSM-22, 70 °C, 4 h, 49.9 mmol 30% H2O2, 8.2 mmol EB, 10 mL acetonitrile. |
25 |
4.0 |
57.5 |
85.0 |
15.0 |
2.3 |
0.4 |
0.7 |
0.1 |
0.5 |
50 |
12.0 |
63.3 |
91.7 |
8.3 |
7.6 |
1.5 |
1.8 |
0.3 |
0.7 |
100 |
18.1 |
68.5 |
91.7 |
8.3 |
12.4 |
2.0 |
2.2 |
0.2 |
1.3 |
200 |
24.4 |
71.7 |
92.6 |
7.4 |
17.5 |
2.3 |
2.7 |
0.1 |
1.7 |
300 |
18.6 |
72.0 |
93.5 |
6.5 |
13.4 |
1.9 |
2.1 |
0.1 |
1.1 |
400 |
16.0 |
67.5 |
90.6 |
9.4 |
10.8 |
1.8 |
1.8 |
0.4 |
1.1 |
Table 7 Effect of reaction time on the oxidation of EBa
Reaction time (h) |
Conv. (%) |
SAP (%) |
Sside-chain (%) |
Sring (%) |
YAP (%) |
YBD (%) |
Y1-PE (%) |
YEQ (%) |
Yethylphenols (%) |
Reaction condition: 1.81 wt% VOx/HZSM-22, 200 mg catalyst, 70 °C, 49.9 mmol 30% H2O2, 8.2 mmol EB, 10 mL acetonitrile. |
1 |
16.9 |
72.2 |
93.5 |
6.5 |
12.2 |
1.8 |
1.8 |
0.8 |
0.3 |
2 |
18.6 |
72.6 |
94.1 |
5.9 |
13.5 |
1.9 |
2.1 |
0.2 |
0.9 |
3 |
21.0 |
74.3 |
94.8 |
5.2 |
15.6 |
2.0 |
2.2 |
0.1 |
1.0 |
4 |
24.4 |
71.7 |
92.6 |
7.4 |
17.5 |
2.3 |
2.7 |
0.1 |
1.7 |
5 |
19.8 |
71.7 |
93.9 |
6.1 |
14.2 |
2.0 |
2.2 |
0.0 |
1.2 |
6 |
20.4 |
71.1 |
92.6 |
7.4 |
14.5 |
2.1 |
2.2 |
0.1 |
1.4 |
Table 8 Effect of reaction temperature on the oxidation of EBa
Reaction temperature (°C) |
Conv. (%) |
SAP (%) |
Sside-chain (%) |
Sring (%) |
YAP (%) |
YBD (%) |
Y1-PE (%) |
YEQ (%) |
Yethylphenols (%) |
Reaction condition: 1.81 wt% VOx/HZSM-22, 200 mg catalyst, 4 h, 49.9 mmol 30% H2O2, 8.2 mmol EB, 10 mL acetonitrile. |
30 |
2.3 |
43.5 |
60.9 |
39.1 |
1.0 |
0.1 |
0.3 |
0.3 |
0.5 |
40 |
6.0 |
51.7 |
73.3 |
26.7 |
3.1 |
0.5 |
0.8 |
0.8 |
0.8 |
50 |
11.5 |
62.6 |
83.5 |
16.5 |
7.2 |
1.0 |
1.4 |
1.0 |
0.9 |
60 |
15.7 |
70.7 |
89.8 |
10.2 |
11.1 |
1.3 |
1.7 |
0.4 |
1.2 |
70 |
24.4 |
71.7 |
92.6 |
7.4 |
17.5 |
2.3 |
2.7 |
0.1 |
1.7 |
80 |
19.7 |
73.6 |
97.0 |
3.0 |
14.5 |
2.3 |
2.2 |
0.2 |
0.4 |
Table 9 Testifying whether the catalyst is heterogeneousa
Catalyst |
Conv. (%) |
SAP (%) |
Sside-chain (%) |
Sring (%) |
YAP (%) |
YBD (%) |
Y1-PE (%) |
YEQ (%) |
Yethylphenols (%) |
Reaction condition: 1.81 wt% VOx/HZSM-22, 200 mg catalyst, 70 °C, 4 h, 49.9 mmol 30% H2O2, 8.2 mmol EB, 10 mL acetonitrile. |
Used solvent |
1.0 |
20.0 |
40.0 |
60.0 |
0.2 |
0.1 |
0.1 |
— |
0.6 |
Used catalyst |
17.0 |
74.1 |
93.5 |
6.5 |
12.6 |
1.6 |
1.6 |
0.2 |
0.9 |
Fresh |
24.4 |
71.7 |
92.6 |
7.4 |
17.5 |
2.3 |
2.7 |
0.1 |
1.7 |
3.2.1. The influence of vanadium loading on the activity. The catalytic performance of the samples and the support for the partial oxidation of ethylbenzene is shown in Table 5. A 0.8% conversion of ethylbenzene with 63.3% selectivity was obtained on HZSM-22. This is negligible compared to the results obtained on the catalysts. Thus, the support might just have effect on the vanadium species to form active sites, and then affect the reaction. The partial oxidation of EB in the present system could be classified into two categories, the side-chain oxidation producing BD, 1-PE, etc.; and benzene ring oxidation producing phenols. It was obvious that over the vanadium loading range tested, the selectivity of side-chain C–H bond oxidation was higher than that of the ring C–H bond oxidation. As the vanadium loading increased, the selectivity of side-chain increased but that of the ring decreased. The conversion of EB increased with vanadium content firstly, and reached the maximum value of 24.4% at 1.81 wt% vanadium content, whereas further increase of vanadium content to 2.86 wt% decreased it to 17.8%. This variation trend is in accordance with that of the amount of extra-framework vanadium species from 0.07 to 1.59 wt%. It implied that highly dispersed extra-framework vanadium species might be responsible for the partial oxidation of EB as well as the yield of AP, BD and 1-PE. With further increase of the vanadium loading to 2.86 wt%, although the extra-framework vanadium species increased dramatically, the formation of crystalline V2O5 might not be beneficial for the conversion of EB. XPS results showed that with the vanadium loading increasing, the surface V4+ increased monotonically. It might imply that the surface V4+ extra-framework vanadium species was beneficial for the conversion of EB and other side-chain products. TPR results also confirmed that those species were isolated vanadium species. With the vanadium content increasing from 0.09 to 0.96 wt%, the selectivity of AP increased from 50.0 to 72.5%, and with further increase of vanadium content to 1.81 wt%, it decreased slightly to 71.7% while on 2.86 wt% catalyst, the selectivity decreased further to 71.3%. These data proved further that highly dispersed extra-framework vanadium species were beneficial for the selectivity of AP while oligomerized vanadium species might result in deeper oxidation. However, the presence of oligomer of aggregated V species and crystalline V2O5 would decrease the selectivity of AP and side-chain of C–H bond oxidation.
3.2.2. The influence of reaction parameters.
The influence of the amount of catalysts. The effect of the amount of catalysts on the activity is demonstrated in Table 6. With the amount of catalysts increasing from 25 mg to 200 mg, the conversion of EB increased from 0.6 to 24.4%, and then decreased to 16.0% with the amount of catalyst of 400 mg. Besides, the selectivity of AP increased from 50.0 to 71.7%, and kept almost the same with 300 mg catalyst (72.0%) then decreased to 67.5%. Obviously, this reaction relies heavily on the amount of catalyst. However, more amounts of catalysts may result in the decomposition of a large volume of hydrogen peroxide, being disadvantageous for the selective reaction of EB to AP, to 200 mg of catalyst is suitable for the present system.
The influence of reaction time. The results obtained with different reaction time on the catalytic oxidation of EB over VOx/HZSM-22 are listed in Table 7. The yield of AP increased gradually from 16.9 to 24.4% with the reaction time of 1–4 h. The yield of by-products of BD, 1-PE and ethylphenols changed in the same way, but the selectivity of AP did not vary significantly. Nevertheless, when the reaction time exceeded 4 h, the yield of all products decreased continuously. The apparent decrease of the conversion of EB might be caused by the fact that the sum of the yield of the products decreased while the formation of other products that could not be detected by our present techniques did not contributed to the conversion calculation.
The influence of temperature. As shown in Table 8, as the temperature rose from 30 to 70 °C, the yield of AP increased monotonically, and then decreased, the optimal reaction temperature was 70 °C under the reaction conditions of hydrogen peroxide/EB molar ratio of 6.1
:
1, 10 mL acetonitrile as solvent, and 4 h of reaction time, where the highest yield of AP was 17.5%. By contrast, the selectivity of AP increased monotonically until the reaction temperature of 80 °C, reaching 73.6%. Moreover, with the temperature increasing to above 60 °C, the self-decomposition of hydrogen peroxide was extremely serious, resulting in the decrease of the conversion of EB.68The effect of several solvents (ethanol, acetone, isopropyl, ethyl acetate, n-butanol, glycol, methanol, acetic acid and acetonitrile), the amount of H2O2 used and the amount of CH3CN was investigated. However, the best yield of ethylbenzene and selectivity remained 17.5% and 72.5%, respectively. Further work is needed to get better results.
Heterogeneous nature of the catalytic reaction. To study whether the selective oxidation of EB is heterogeneous, the VOx/HZSM-22 was added to a 50 mL two-necked round-bottom flask, and then adding 10 mL acetonitrile, and let them keep 4 h in a water bath with a reflux condenser at 70 °C. After four hours, the mixture was filtered to obtain the used solvent and used catalyst, 8.2 mmol EB, 10 mL acetonitrile and 49.9 mmol 30% H2O2 were added to the round-bottom flask with used catalyst and kept for 4 h at 70 °C. The same procedure was employed to the used solvent. All the results combing with the results of fresh VOx/HZSM-22 are shown in Table 9. The used solvent showed a slight conversion of EB but the used catalyst showed 17.0% conversion of EB. Both the conversion of EB and the selectivity of AP were lower than that of the fresh VOx/HZSM-22. It demonstrated that the reaction is heterogeneous and a small amount of vanadium species was leached in the solvent. The stability of the catalyst deserved further study.
The recyclability of the catalyst. The 1.81 wt% VOx/HZSM-22 was reused for two times by conducting the same experiments with the used catalyst. The results are shown in the Table S1 (ESI†). After second times' usage, the catalyst almost lost the catalytic activity because the catalyst had a 55.6% leaching rate from the results of the ICP-AES. At first, to explore if carbon deposition deactivated the catalyst, the used catalyst was calcined at 550 °C for 6 h and then reused to test the catalytic activity. The results are shown in Table S1 (ESI†). The conversion of ethylbenzene using the regenerated catalyst was a little higher than that of the second circle (without calcination). The above data indicated that the origin of the deactivation of the catalyst was not carbon deposition. In order to figure out further the reason of activity decrease, we performed another contrast experiment. The designed catalyst, acetonitrile and hydrogen peroxide were added into the round-bottom flask under reaction conditions and kept for 4 h. Then filtrating the mixture to obtain solution and used solid catalyst, the solution and used solid catalyst were used to do the same catalytic experiment, respectively. The results are shown in Table S1.† The conversion of ethylbenzene was only around 2.1% by using the solution obtained. When the treated solid catalyst was used, the conversion of ethylbenzene decreased significantly, only 2.2% ethylbenzene conversion was obtained. Although the catalytic reaction is heterogeneous, the hydrogen peroxide can dissolve a large amount of extra-framework vanadium species. Thus, enhancing the stability of the catalyst remained one challenge.
4. Conclusions
The results showed that the vanadium species could be incorporated into the framework and/or existed extra-framework of VOx/HZSM-22 depending on the loading. The highly dispersed extra-framework vanadium species was advantageous for the selective oxidation of ethylbenzene with the EB conversion of 24.4% and good AP selectivity of 72.5%. Specifically, the surface extra-framework V4+ was responsible for the conversion of EB as well as yield of AP, BD and 1-PE. Furthermore, the formation of crystalline V2O5 was disadvantageous for both the conversion of ethylbenzene and side-chain oxidation.
Acknowledgements
This work is financially supported by National Natural Science Foundation of China (No. 21372167), and the technical support from the Analytical and Testing Centre of Sichuan University are cordially acknowledged.
Notes and references
- H. J. Sanders, H. F. Keag and H. S. Mccullough, Ind. Eng. Chem., 1953, 45, 2–14 CrossRef CAS.
- G. A. Olah, Friedel-Crafts and Related Reactions: Miscellaneous reactions, Interscience Publishers, NYC, 1965 Search PubMed.
- Z. H. Luan, J. Xu, H. Y. He, J. Klinowski and L. Kevan, J. Phys. Chem., 1996, 100, 19595–19602 CrossRef CAS.
- T. Matsue, M. Fujihira and T. Osa, J. Electrochem. Soc., 1981, 128, 2565–2569 CrossRef CAS.
- S. Velusamy and T. Punniyamurthy, Tetrahedron Lett., 2003, 44, 8955–8957 CrossRef CAS.
- V. Choudhary, J. Indurkar, V. Narkhede and R. Jha, J. Catal., 2004, 227, 257–261 CrossRef CAS.
- M. Zhu, X. Wei, B. Li and Y. Yuan, Tetrahedron Lett., 2007, 48, 9108–9111 CrossRef CAS.
- M. Arshadi, M. Ghiaci, A. Rahmanian, H. Ghaziaskar and A. Gil, Appl. Catal., B, 2012, 119, 81–90 CrossRef.
- S. Vetrivel and A. Pandurangan, Ind. Eng. Chem. Res., 2005, 44, 692–701 CrossRef CAS.
- M. Hudlicky, Oxidations in organic chemistry, American Chemical Society, Washington, DC, 1990 Search PubMed.
- R. Wang, B. Gao and W. Jiao, Appl. Surf. Sci., 2009, 255, 4109–4113 CrossRef CAS.
- C. Lu, Z. Fu, Y. Liu, F. Liu, Y. Wu, J. Qin, X. He and D. Yin, J. Mol. Catal. A: Chem., 2010, 331, 106–111 CrossRef CAS.
- J. Kochi and R. Sheldon, Metal Catalyzed Oxidation of Organic Compounds, Academic, New York, NY, 1981 Search PubMed.
- R. Alcántara, L. Canoira, P. G. Joao, J.-M. A. Santos and I. Vázquez, Appl. Catal., A, 2000, 203, 259–268 CrossRef.
- V. Raji, M. Chakraborty and P. A. Parikh, Ind. Eng. Chem. Res., 2012, 51, 5691–5698 CrossRef CAS.
- H. Y. Li, H. Ma, X. H. Wang, J. Gao, C. Chen, S. Shi, M. J. Qu, N. Feng and J. Xu, J. Energy Chem., 2014, 23, 742–746 CrossRef.
- M. Ghorbanloo, S. Ghamari, N. Shahbakhsh and S. W. Ng, J. Braz. Chem. Soc., 2014, 25, 2073–2079 CAS.
- S. S. Acharyya, S. Ghosh and R. Bal, Ind. Eng. Chem. Res., 2014, 53, 20056–20063 CrossRef CAS.
- P. Visuvamithiran, K. Shanthi, M. Palanichamy and V. Murugesan, Catal. Sci. Technol., 2013, 3, 2340–2348 CAS.
- B. Gutmann, P. Elsner, D. Roberge and C. O. Kappe, ACS Catal., 2013, 3, 2669–2676 CrossRef CAS.
- X. L. Yang, M. H. Xie, C. Zou, Y. He, B. Chen, M. O'Keeffe and C. D. Wu, J. Am. Chem. Soc., 2012, 134, 10638–10645 CrossRef CAS PubMed.
- M. Arshadi, M. Ghiaci, A. Rahmanian, H. Ghaziaskar and A. Gil, Appl. Catal., B, 2012, 119, 81–90 CrossRef.
- P. Visuvamithiran, K. Shanthi, M. Palanichamy and V. Murugesan, Catal. Sci. Technol., 2013, 3, 2340–2348 CAS.
- M. Hosseini-Sarvari, T. Ataee-Kachouei and F. Moeini, RSC Adv., 2015, 5, 9050–9056 RSC.
- S. S. Acharyya, S. Ghosh and R. Bal, Ind. Eng. Chem. Res., 2014, 53, 20056–20063 CrossRef CAS.
- M. Salavati-Niasari and S. Abdolmohammadi, J. Porous Mater., 2009, 16, 19–26 CrossRef CAS.
- E. P. Reddy and R. S. Varma, J. Catal., 2004, 221, 93–101 CrossRef CAS.
- K. V. R. Chary, G. Kishan, C. P. Kumar and G. V. Sagar, Appl. Catal., A, 2003, 246, 335–350 CrossRef CAS.
- V. P. Vislovskiy, J.-S. Chang, M.-S. Park and S.-E. Park, Catal. Commun., 2002, 3, 227–231 CrossRef CAS.
- X. Gao and I. E. Wachs, J. Catal., 2000, 192, 18–28 CrossRef CAS.
- P. Concepción, M. Navarro, T. Blasco, J. L. Nieto, B. Panzacchi and F. Rey, Catal. Today, 2004, 96, 179–186 Search PubMed.
- B. R. Jermy, S. Y. Kim, D. K. Kim and D. W. Park, J. Ind. Eng. Chem., 2011, 17, 130–137 CrossRef CAS.
- T. Radhika and S. Sugunan, Catal. Commun., 2007, 8, 150–156 CrossRef CAS.
- G. Centi, S. Perathoner, F. Trifiro, A. Aboukais, C. F. Aissi and M. Guelton, J. Phys. Chem., 1992, 96, 2617–2629 CrossRef CAS.
- D. Verboekend, A. M. Chabaneix, K. Thomas, J.-P. Gilson and J. Pérez-Ramírez, CrystEngComm, 2011, 13, 3408–3416 RSC.
- N. Kumar, L. Lindfors and R. Byggningsbacka, Appl. Catal., A, 1996, 139, 189–199 CrossRef CAS.
- A. K. Jamil, O. Muraza, M. Yoshioka, A. M. Al-Amer, Z. H. Yamani and T. Yokoi, Ind. Eng. Chem. Res., 2014, 53, 19498–19505 CrossRef CAS.
- S. Shylesh and A. Singh, J. Catal., 2004, 228, 333–346 CrossRef CAS.
- Q. Liu, L. Zhu, L. Li, B. Guo, X. Hu and C. Hu, J. Mol. Catal. A: Chem., 2010, 331, 71–77 CrossRef CAS.
- B. Guo, L. Zhu, X. Hu, Q. Zhang, D. Tong, G. Li and C. Hu, Catal. Sci. Technol., 2011, 1, 1060–1067 CAS.
- T. Sen, V. Ramaswamy, S. Ganapathy, P. Rajamohanan and S. Sivasanker, J. Phys. Chem., 1996, 100, 3809–3817 CrossRef CAS.
- K. Wu, B. Li, C. Han and J. Liu, Appl. Catal., A, 2014, 479, 70–75 CrossRef CAS.
- R. Baran, T. Onfroy, T. Grzybek and S. Dzwigaj, Appl. Catal., B, 2013, 136, 186–192 CrossRef.
- G. Du, S. Lim, M. Pinault, C. Wang, F. Fang, L. Pfefferle and G. L. Haller, J. Catal., 2008, 253, 74–90 CrossRef CAS.
- Y.-M. Liu, Y. Cao, N. Yi, W.-L. Feng, W.-L. Dai, S.-R. Yan, H.-Y. He and K.-N. Fan, J. Catal., 2004, 224, 417–428 CrossRef CAS.
- G. Coudurier, C. Naccache and J. C. Vedrine, J. Chem. Soc., Chem. Commun., 1982, 1413–1415 RSC.
- J. R. Sohn, S. J. DeCanio, J. H. Lunsford and D. J. O'Donnell, Zeolites, 1986, 6, 225–227 CrossRef CAS.
- U. J. Etim, B. Xu, R. Ullah and Z. Yan, J. Colloid Interface Sci., 2016, 463, 188–198 CrossRef CAS PubMed.
- A. Aronne, S. Esposito, C. Ferone, M. Pansini and P. Pernice, J. Mater. Chem., 2002, 12, 3039–3045 RSC.
- Y.-M. Liu, Y. Cao, N. Yi, W.-L. Feng, W.-L. Dai, S.-R. Yan, H.-Y. He and K.-N. Fan, J. Catal., 2004, 224, 417–428 CrossRef CAS.
- M. Soriano, J. Cecilia, A. Natoli, J. Jiménez-Jiménez, J. L. Nieto and E. Rodríguez-Castellón, Catal. Today, 2015, 254, 36–42 CrossRef CAS.
- B. R. Jermy, S. Y. Kim, D. K. Kim and D. W. Park, J. Ind. Eng. Chem., 2011, 17, 130–137 CrossRef CAS.
- M. D. Soriano, J. A. Cecilia, A. Natoli and J. Jiménez-Jiménez, Catal. Today, 2015, 254, 36–42 CrossRef CAS.
- M. D. Soriano, J. M. López Nieto, F. Ivars, P. Concepción and E. Rodríguez-Castellón, Catal. Today, 2012, 192, 28–35 CrossRef CAS.
- L.-X. Dai, K. Tabata, E. Suzuki and T. Tatsumi, Chem. Mater., 2001, 13, 208–212 CrossRef CAS.
- K. Chao, C. Wu, H. Chang, L. Lee and S.-F. Hu, J. Phys. Chem. B, 1997, 101, 6341–6349 CrossRef.
- M. Piumetti, B. Bonelli, M. Armandi, L. Gaberova, S. Casale, P. Massiani and E. Garrone, Microporous Mesoporous Mater., 2010, 133, 36–44 CrossRef CAS.
- K. Wu, B. Li, C. Han and J. Liu, Appl. Catal., A, 2014, 479, 70–75 CrossRef CAS.
- C. W. Lee, W. J. Lee, Y. K. Park and S.-E. Park, Catal. Today, 2000, 61, 137–141 CrossRef CAS.
- S. Dzwigaj, Curr. Opin. Solid State Mater. Sci., 2003, 7, 461–470 CrossRef CAS.
- G. Centi, S. Perathoner, F. Trifiro, A. Aboukais, C. Aissi and M. Guelton, J. Phys. Chem., 1992, 96, 2617–2629 CrossRef CAS.
- W. J. L. Chul Wee Lee, Y. K. Park and S.-E. Park, Catal. Today, 2000, 61, 137–141 CrossRef.
- S. Dzwigaj, E. Ivanova, R. Kefirov, K. Hadjiivanov, F. Averseng, J. M. Krafft and M. Che, Catal. Today, 2009, 142, 185–191 CrossRef CAS.
- B. W. Jan Kornatowski, J. Jirkovský, E. Löffler and W. Pilz, J. Chem. Soc., Faraday Trans., 1996, 92, 1067–1078 RSC.
- M. Banares, J. Cardoso, F. Agulló-Rueda, J. Correa-Bueno and J. Fierro, Catal. Lett., 2000, 64, 191–196 CrossRef CAS.
- A. Held, J. Kowalska-Kuś and K. Nowińska, Catal. Commun., 2012, 17, 108–113 CrossRef CAS.
- B. Solsona, T. Blasco, J. L. Nieto, M. Pena, F. Rey and A. Vidal-Moya, J. Catal., 2001, 203, 443–452 CrossRef CAS.
- R. Yu, F.-S. Xiao, D. Wang, J. Sun, Y. Liu, G. Pang, S. Feng, S. Qiu, R. Xu and C. Fang, Catal. Today, 1999, 51, 39–46 CrossRef CAS.
Footnote |
† Electronic supplementary information (ESI) available. See DOI: 10.1039/c6ra05906f |
|
This journal is © The Royal Society of Chemistry 2016 |