DOI:
10.1039/C6RA05886H
(Paper)
RSC Adv., 2016,
6, 47043-47054
In vitro interaction investigation between three Ru(II) arene complexes and human serum albumin: structural influences†
Received
5th March 2016
, Accepted 20th April 2016
First published on 25th April 2016
Abstract
Ru(II) arene complex-based anticancer drugs have attracted great attention in biomedical and pharmacological areas. The investigation of structural influences helps in understanding biological effects and in contributing to the development of new drugs. The study of protein–drug interactions is very important for understanding the mechanism behind the bioactivities of drugs. To examine the potential of Ru(II) arene complexes as therapeutics, we systematically investigated the structural influences of three Ru(II) arene complexes (Ru-1, Ru-2, and Ru-3) based on their in vitro interaction with human serum albumin (HSA) by multi-spectroscopic methods. The binding interactions of three drugs with HSA results in the formation of three HSA–drug complexes, and van der Waals forces and hydrogen bonding played major roles in stabilizing these complexes. The changes of micro-environments and conformations of HSA were significant and the biological activity of HSA was weakened when present in the three drugs. We found that a number of Ru(II) arene groups and space steric hindrance were responsible for the differences in the in vitro interactions between different Ru(II) arene complexes and HSA. Our results together provide further molecular level understanding of binding interactions of protein with Ru(II) arene complexes and a strategy for the research of structural influences.
1. Introduction
Ru(II) arene complexes have been widely investigated by biochemical scientists due to their superior antiviral, antibacterial, antitumor, and anticancer activities. Since Ru(II) arene complexes exhibit a higher anticancer activity and lower toxicity than traditional platinum drugs, they are considered as promising lead Ru(II)-based anticancer drugs of the near future.1,2 Some researchers have revealed that the anticancer activities of Ru(II) arene complexes are affected largely by the property of their chelating ligands to various extents. Beckford and co-workers exhibited the good anticancer activity of Ru(II) arene complexes with thiosemicarbazones against different human cancer cell lines.3 Smith’s group demonstrated the antiparasitic activities of mono- and dinuclear Ru(II) arene complexes.4 Pettinari and co-workers displayed the potent and selective anticancer activity of Ru(II) arene complexes with curcumin analogs.5 Su et al. validated the high antitumor activities of numerous Ru(II) arene complexes.6,7 Since variations in molecular structure may be responsible for the pharmacological properties of drugs,8 the investigation of structural influences is quite significant for designing and understanding the biological activities of Ru(II) arene complex-based anticancer drugs.
In order to obtain detailed information regarding the absorption, distribution, metabolism, and excretion of pharmaceutical agents in the circulatory system, it is of much importance to investigate interactions between exogenous drugs and serum proteins.9,10 As the most abundant protein among serum proteins, human serum albumin (HSA) participates in a cyclic process of numerous therapeutic and diagnostic drugs within the human body. Due to the medicinal importance and the distinctive ligand-binding properties of HSA, HSA is the fully-deserving model protein of interaction investigations between different exogenous drugs and protein. In the context of interaction between Ru(II) arene complexes and HSA, Sánchez-Delgado’s group illustrated that arene–Ru(II)–chloroquine complexes bound to HSA in a covalent manner and reduced the α-helical content of the structure in HSA.11 Our group indicated that the micro-environments and conformations of HSA changed obviously in the presence of Ru(II) arene complexes.12,13 Such investigations are highly significant for thoroughly understanding the pharmacokinetic and pharmacological behaviors of Ru(II) arene complex-based anticancer drugs.
Research about selected Ru(II) arene complexes and HSA has been reported, but most of them just stay at an investigation about molecular interaction,14 with the help of interaction to study the structural influences systematically being rarely involved. It is quite necessary and important to compare the interactions between different Ru(II) arene complexes and HSA for further evaluating their biological actions on organisms from a molecular biology level.
Various methods such as UV-vis,15 fluorescence16,17 and circular dichroism (CD) spectroscopy,18,19 equilibrium dialysis,20 and potentiometry21 have been used to investigate the binding of ligands to proteins. Among these methods, equilibrium dialysis is used widely, but it requires the analysis of the free and total drug concentration and takes a long time.22 In the potentiometric method, ion-selective electrodes are used. These electrodes have a lack of selectivity for many ligands such as drug molecules.21 Fluorescence techniques are very helpful in protein–drug interactions due to their high sensitivity, rapidity, and ease of implementation. The fluorescence measurements can provide some useful information about the binding of small molecules to protein such as the binding mechanism, binding mode, binding constants, and binding numbers.23
In this study, three Ru(II) arene complexes (Ru-1, Ru-2, and Ru-3), which exhibit good anticancer activities against several human cancer cells,6,7 are purposely chosen to compare their in vitro interactions with HSA. The structures and relationships of three Ru(II) arene complexes are shown in Fig. 1. The structural influences for HSA interactions of three drugs were characterized systematically by multi-spectroscopic methods, including UV-vis absorption, fluorescence, FT-IR, CD, and mass spectroscopy. Particularly, micro-environmental and conformational changes of HSA induced by the three drugs were studied by three-dimensional fluorescence spectrophotometry, FT-IR, and the CD technique. Comparisons about the differences in the quenching mechanism, thermodynamic parameters, binding constants, and binding sites have been associated with their structure for the discussion of structural influences. This study may provide insights into the structural influences at the molecular level which is valuable in providing a theoretical basis for the subsequent development of Ru(II) arene complexes as potential therapeutics.
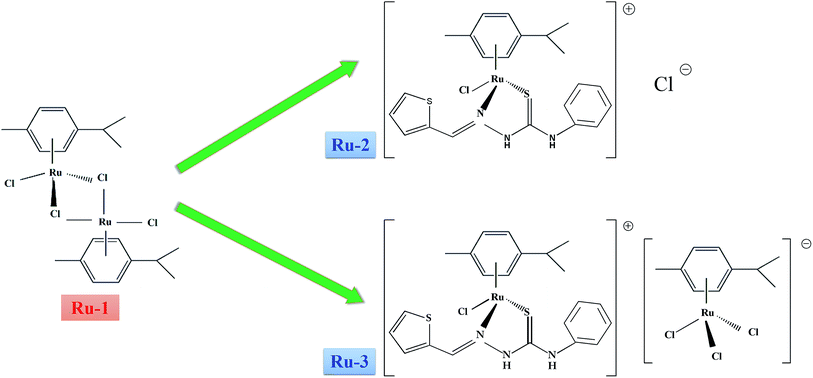 |
| Fig. 1 Structures and relationships of the three Ru(II) arene complexes. | |
2. Materials and methods
2.1. Materials
Three Ru(II) arene complexes were synthesized and characterized according to the reported literature.6,7 The stock solutions of the three drugs were prepared by dissolving their crystals in dimethyl sulphoxide (DMSO). HSA, warfarin, and ibuprofen were purchased from Sigma (Sigma-Aldrich, USA). HSA was dissolved in phosphate buffer saline (PBS, 0.1 mol L−1, pH 7.4) and stored in a refrigerator. The concentration of HSA was determined spectrophotometrically using an extinction coefficient at 280 nm of 36
600 L mol−1 cm−1.24 All other chemical reagents were of analytical reagent grade and used as received without further purification. Ultrapure water with a resistivity of 18.2 MΩ cm was produced by passing through a RiOs 8 unit followed by a Millipore-Q Academic purification set (Millipore, Bedford, MA, USA) and used throughout the whole experiments.
2.2. Apparatus
Fluorescence measurements were performed on a Perkin-Elmer LS-55 luminescence spectrometer (PerkinElmer, USA). Time-resolved fluorescence decay traces were recorded with a Fluorolog-3 system (Horiba Jobin Yvon, France). UV-vis absorption spectra were measured on a Cary 100 UV-vis spectrophotometer (Agilent Technologies, Inc., Australia). FT-IR spectra were recorded on a Nicolet iS10 spectrometer (Thermo, USA). CD spectra were recorded on a Chirascan CD spectrometer (Applied Photophysics, England). Ultrahigh performance liquid chromatography-mass spectrometry (UPLC-MS) was recorded on a ACQUITY UPLC/XEVO G2 Q TOF mass spectrometer (Waters Corporation, Milford, USA) equipped with an electrospray ion source operating in either positive or negative ESI mode. Chromatographic separation was achieved on a ACQUITY UPLC BEH300 C4 column (1.7 μm, 2.1 × 100 mm). Quartz cells (1.0 cm path length) were used for all measurements. All pH measurements were made with a basic pH meter PB-10 (Sartorius Scientific Instruments Co., Ltd., China).
2.3. UV-vis absorption spectrometry
UV-vis absorption spectra of HSA, and difference absorption spectra between the three HSA–drug systems and the corresponding drug were measured from 200 nm to 350 nm. The solutions of a blank buffer and the sample were placed in reference and sample cuvettes, respectively. The concentrations of HSA and the three drugs were all 2.0 × 10−6 mol L−1.
2.4. Fluorescence spectrometry
Steady-state fluorescence spectra of HSA with different concentrations of the three drugs were recorded at 298 K, 304 K, and 310 K in the wavelength range of 300–450 nm. The widths of the excitation and emission slits were set to 10.0 nm and 8.0 nm, and the excitation wavelength of 295 nm was chosen. Each spectrum was the average of three scans. The concentration of HSA was 2.0 × 10−6 mol L−1. The concentration of Ru-1 increased from 0 to 1.25 × 10−6 mol L−1 with an interval of 1.25 × 10−7 mol L−1. The concentration of Ru-2 increased from 0 to 2.5 × 10−6 mol L−1 with an interval of 2.5 × 10−7 mol L−1. The concentration of Ru-3 increased from 0 to 1.0 × 10−6 mol L−1 with an interval of 1.0 × 10−7 mol L−1.
Binding site investigation experiments were performed using two site marker probes through keeping the concentrations of HSA and the probes constant but varying the concentrations of the three drugs only. Other scanning parameters were the same as that of the steady-state fluorescence spectra. The concentrations of the probes and HSA were all 2.0 × 10−6 mol L−1.
Time-resolved fluorescence decay traces were recorded at room temperature using an excitation wavelength of 278 nm and emission wavelength of 350 nm. The concentrations of HSA and the three drugs were all 2.0 × 10−6 mol L−1.
Three-dimensional fluorescence spectra of HSA and three HSA–drug systems were recorded with the excitation wavelength in the range of 200–350 nm and the emission wavelength in the range of 200–500 nm with increments of 5 nm. Other scanning parameters were the same as that of the steady-state fluorescence spectra. The concentrations of HSA and the three drugs were all 1.0 × 10−6 mol L−1.
2.5. FT-IR spectrometry
FT-IR spectra of HSA and the three HSA–drug systems were recorded in the range of 1500–1800 cm−1 with 128 interferograms and a resolution of 4 cm−1. The corresponding absorbance contributions of the buffer and three drugs were recorded and digitally subtracted with the same instrumental parameters. The subtraction of the reference spectrum from the spectrum of the protein solution was carried out in accordance with the criteria that the straight baseline was obtained between 2000 and 1750 cm−1.25 The concentrations of HSA and the three drugs were all 1.0 × 10−3 mol L−1.
2.6. CD spectrometry
CD spectra were recorded from 190 to 320 nm at 298 K under constant nitrogen flush. CD profiles were obtained employing a scan speed of 500 nm min−1 and a response time of 0.5 s. Thermal unfolding scans were measured from 20 °C to 90 °C at 208 nm with a 0.5 °C step size at 1 °C min−1 ramp rate with ±0.1 °C tolerance. Each spectrum was the average of three successive scans and was corrected by buffer solution. The concentration of HSA was 2.0 × 10−6 mol L−1, and the concentrations of the three drugs were all 2.0 × 10−5 mol L−1.
2.7. UPLC-MS
HSA and the three HSA–drug systems (molar ratio of 1
:
1 and 1
:
2) were prepared in 0.1% formic acid in acetonitrile and introduced into UPLC-MS with a syringe pump. The mobile phase consisted of a constant flow rate gradient of methanol, 0.1% formic acid in water and 0.1% formic acid in acetonitrile under an elution program at a flow rate of 0.2 mL min−1. The injection volume was 10 μL and the analysis run time was 12 min for each injection. The optimal conditions of analysis were set as follows: capillary voltage of 2.5 kV, sampling cone voltage of 30.0 V, and extraction cone voltage of 4.0 V. The temperature was set at 100 °C, the desolvation gas temperature was 350 °C and the desolvation gas flow was 800 L h−1. The mass spectrometer was calibrated using a solution of sodium formate before the experiment. Full-scan mass spectral data were produced across the deconvolution m/z range of 1190–1480 Da and the protein molecular mass range of 62
000–75
000 Da. Scans were of 12 min duration. Data were collected in centroid mode and the mass was corrected during acquisition using an external reference (Lock-Spray) comprising 200 pg mL−1 solution of leucine-enkephalin via a lockspray interface, generating a reference ion at 556.277 Da ([M + H]+) for positive ESI mode.
3. Results and discussion
3.1. Effect of Ru(II) arene complex on HSA fluorescence spectra
Intrinsic fluorescence quenching of protein is widely used to elucidate the binding mechanism of its interaction with different endogenous and exogenous ligands.26,27 In order to investigate the distinctions of in vitro interactions between the three Ru(II) arene complexes and HSA, steady-state fluorescence spectra of HSA with different concentrations of the three drugs were recorded under the same conditions (298 K, pH 7.4 of 0.1 mol L−1 PBS). As illustrated in Fig. 2, the three drugs exhibit almost no fluorescence but HSA shows strong fluorescence at 350 nm under the same conditions. Moreover, a remarkable decrease of the fluorescence intensity of HSA is observed upon the addition of the three drugs, and the fluorescence intensity of HSA is quenched regularly with the increase of the concentration of the three drugs, which implies that the three drugs quench the intrinsic fluorescence of HSA mainly through a concentration-dependent manner. These concentration-dependent fluorescence changes indicate the stronger binding ability to HSA at higher concentrations of the three drugs. The insets of Fig. 2 show that the results agree well with the fluorescence quenching equation excellently at lower concentrations of the three drugs but depart from the initial linearity at higher concentrations of the three drugs. In order to avoid the inner filter effects, all experiments are carried out within the lower concentrations of the three drugs. Interestingly, the quenching efficiencies for the three drugs are different, and 8.75 × 10−7 mol L−1 of Ru-1, 1.75 × 10−6 mol L−1 of Ru-2, and 6.0 × 10−7 mol L−1 of Ru-3 present an equal quenching efficiency of 15.6%. Since the three Ru(II) arene complexes show almost no absorbance in the same wavelength range of 300–450 nm (Fig. S1†), these Ru(II) arene complexes cannot quench the fluorescence of HSA by the inner filter effect or resonance energy transfer.28 On the other hand, the reported possible quenching mechanism of a Ru(II) arene complex to fluorescent substances is mainly photoinduced electron transfer (PET).29,30 Due to the stable combination of the Ru(II) arene complex with HSA, PET can easily occur between HSA and the Ru(II) arene complex. Meanwhile, the central Ru(II) ion of the Ru(II) arene complex has partially filled d-orbitals and can be used as an electron acceptor. The ultrafast PET from HSA to the Ru(II) arene complex prevents the normal recombination of electrons and the highest occupied molecular orbital in the tryptophan residue. Therefore, the electron returns to the ground state with a non-radiative transition, resulting in the fluorescence quenching of HSA. Since the Ru(II) arene group plays a major role in the fluorescence quenching process, Ru-3 exhibits the strongest quenching ability while Ru-2 shows the weakest quenching ability on the intrinsic fluorescence of HSA.
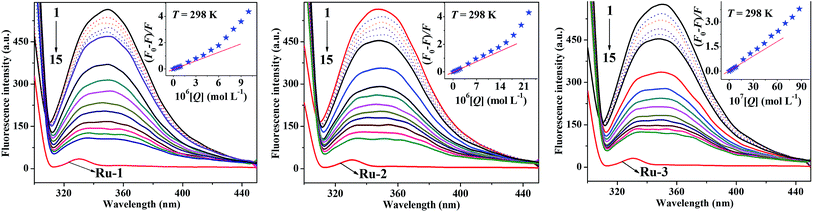 |
| Fig. 2 Fluorescence spectra of HSA with three Ru(II) arene complexes. Insets correspond to the Stern–Volmer plots of three HSA–drug systems at 298 K. c (HSA) = 2.0 × 10−6 mol L−1; c (Ru-1)/(10−6 mol L−1), 1–15: 0; 0.2; 0.4; 0.6; 0.8; 1; 2; 3; 4; 5; 6; 7; 8; 9; 10; c (Ru-1) = 1.0 × 10−5 mol L−1; c (Ru-2)/(10−6 mol L−1), 1–15: 0; 0.45; 0.9; 1.35; 1.8; 2.25; 4.5; 6.75; 9; 11.25; 13.5; 15.75; 18; 20.25; 22.5; c (Ru-2) = 2.25 × 10−5 mol L−1; c (Ru-3)/(10−7 mol L−1), 1–15: 0; 1.75; 3.5; 5.25; 7; 8.75; 17.5; 26.25; 35; 43.75; 52.5; 61.25; 70; 78.75; 87.5; c (Ru-3) = 8.75 × 10−6 mol L−1. | |
3.2. Fluorescence quenching mechanism
Static and dynamic quenching, which are a principal fluorescence quenching mechanism, can be distinguished by the difference in the temperature dependence through steady-state fluorescence spectra. Fluorescence quenching constants usually increase with the increment of temperature for dynamic quenching, while the reverse is true for static quenching. In order to understand whether fluorescence quenching is in a dynamic or static mode, steady-state fluorescence quenching experiments at three different temperatures (298 K, 304 K, and 310 K) were performed to explore the quenching mechanism and thermodynamics of drug binding. All fluorescence data can be analyzed by the well-known Stern–Volmer equation:28 |
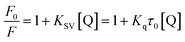 | (1) |
where F0 and F are the steady-state fluorescence intensities of HSA in the absence and presence of the drug, respectively. KSV denotes the Stern–Volmer quenching constant, [Q] is the concentration of drug, Kq is the bimolecular quenching constant (maximum value of 2.0 × 1010 L mol−1 s−1 for dynamic quenching),28 and τ0 represents the fluorescent lifetime of HSA (τ0 = 1.0 × 10−8 s), respectively.13 Stern–Volmer plots of the three HSA–drug systems are shown in Fig. S2,† and the calculated KSV values and Kq values are listed in Table 1. Both KSV values and Kq values decrease with the temperature rising in the three HSA–drug systems, indicating that the quenching mechanisms of the three HSA–drug systems are all static quenching rather than dynamic quenching. All Kq values are larger than 2.0 × 1010 L mol−1 s−1, reconfirming the static quenching mechanism. Furthermore, the KSV values of the HSA–Ru-3 system are much higher than those of the other two HSA–drug systems at the same temperature, suggesting that Ru-3 exhibits a stronger quenching ability than Ru-1 and Ru-2.
Table 1 Stern–Volmer quenching constants KSV, bimolecular quenching constants Kq, associative binding constants Ka, and relative thermodynamic parameters for the three HSA–drug systems
System |
T (K) |
KSV (105 L mol−1) |
R2a |
Kq (1013 L mol−1 s−1) |
Ka (105 L mol−1) |
R2a |
ΔH (kJ mol−1) |
ΔG (kJ mol−1) |
ΔS (J mol−1 K−1) |
R2a |
R2 is the correlation coefficient. |
HSA–Ru-1 |
298 |
2.01 |
0.999 |
2.01 |
5.55 |
0.996 |
−44.94 |
−32.77 |
−40.84 |
0.999 |
304 |
1.46 |
0.998 |
1.46 |
3.88 |
0.999 |
−32.53 |
310 |
1.29 |
0.998 |
1.29 |
2.75 |
0.998 |
−32.28 |
HSA–Ru-2 |
298 |
1.06 |
0.998 |
1.06 |
0.415 |
0.999 |
−42.42 |
−26.34 |
−53.91 |
0.999 |
304 |
1.01 |
0.999 |
1.01 |
0.302 |
0.999 |
−26.07 |
310 |
0.97 |
0.998 |
0.97 |
0.214 |
0.999 |
−25.70 |
HSA–Ru-3 |
298 |
3.13 |
0.999 |
3.13 |
6.90 |
0.997 |
−90.19 |
−33.35 |
−190.73 |
0.993 |
304 |
2.89 |
0.998 |
2.89 |
3.61 |
0.998 |
−32.21 |
310 |
2.81 |
0.999 |
2.81 |
1.69 |
0.999 |
−31.07 |
For all static quenching processes, the fluorescence data can be analyzed by a modified Stern–Volmer equation:28
|
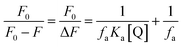 | (2) |
Herein, ΔF is the difference of the fluorescence intensities of the fluorophore in the absence and presence of a quencher, fa is the fraction of the initial fluorescence that is accessible to the quencher, and Ka is the associative binding constant, respectively.31 Modified Stern–Volmer plots of the three HSA–drug systems are shown in Fig. S3,† and the calculated Ka values of the three HSA–drug systems are also listed in Table 1. The decreasing trend of Ka values with the increase of temperature, similar to KSV discussed above, suggests the static fluorescence quenching of the three HSA–drug systems. High associative binding constants for interactions between protein and ligands are in the range of 106 to 108 L mol−1.32 As obtained in the present study, the range of associative binding constants of the three HSA–drug systems is 2.14 × 104 L mol−1 to 6.90 × 105 L mol−1 during three different temperatures, indicating a relatively weak interaction between the three drugs and HSA. Lower binding constants observed at a high temperature suggest that HSA may bind weakly fewer drugs at body temperature than at room temperature, thus making the release of drugs easier from plasma protein to target organs. Moreover, an interesting phenomenon regarding the effect of temperature on different drugs was observed as is evident from the results presented in Fig. S3† and Table 1. The effect of temperature on the three drugs is related to the number of Ru(II) arene groups and space steric hindrance, and the order is as follows: Ru-3 > Ru-1 > Ru-2. There is one Ru(II) arene group in Ru-2 but two Ru(II) arene groups in Ru-3 and Ru-1, and two Ru(II) arene groups in Ru-1 exert bigger space hindrance than in Ru-3, and thus, Ru-3 is most easily affected by temperature and Ru-2 has the weakest influence of temperature.
UV-vis absorption spectroscopy can be used to illustrate the fluorescence quenching mechanism.33 The spectrum of the fluorophore usually changes for static quenching but remains constant for dynamic quenching. Fig. 3A shows the UV-vis absorption spectrum of HSA, and the difference absorption spectra between the three HSA–drug systems and their corresponding drug at the same concentrations. Since the UV-vis absorption spectrum of HSA does not overlap perfectly with the difference absorption spectra between the three HSA–drug systems and their corresponding drug within experimental errors, the fluorescence quenching mechanisms among the three HSA–drug systems are all static quenching mechanisms. In addition, Ru-3 exhibits the greatest difference compared to Ru-1 and Ru-2, suggesting the strongest complex formation of Ru-3 with HSA than of the other two drugs.
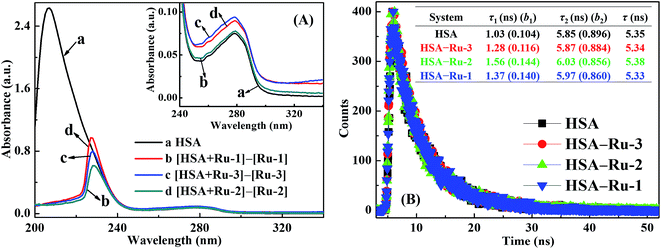 |
| Fig. 3 (A) UV-vis absorption spectrum of HSA, and difference absorption spectra between the three HSA–drug systems and their corresponding drug. c (HSA) = c (Ru-1) = c (Ru-2) = c (Ru-3) = 2.0 × 10−6 mol L−1. (B) Fluorescence decay traces of HSA and the three HSA–drug systems. τ is the fluorescence lifetime of HSA and b is the normalized pre-exponential factor, respectively. c (HSA) = c (Ru-1) = c (Ru-2) = c (Ru-3) = 2.0 × 10−6 mol L−1. | |
A detailed fluorescence quenching mechanism can be directly distinguished by investigating the variation of fluorescence lifetime through time-resolved fluorescence spectrometry.28 The fluorescence lifetime of a fluorophore usually changes in a dynamic quenching process but remains constant during a static quenching process. Fluorescence decay traces of HSA and the three HSA–drug systems are shown in Fig. 3B. As shown in Fig. 3B, HSA exhibits two fluorescence decay components no matter whether the three drugs are present or not. Therefore, all fluorescence decay curves are fitted with a bi-exponential equation, and the average fluorescence lifetime is given by the sum of ∑τibi products.34 The average fluorescence lifetime of HSA changes slightly from 5.35 ns to 5.34 ns, 5.38 ns, and 5.33 ns after the addition of Ru-3, Ru-2, and Ru-1, respectively, and such tiny variations in the fluorescence lifetime of HSA can be omitted within experimental errors. The almost constant fluorescence lifetime of HSA further confirms the static quenching mechanism of the three HSA–drug systems.
3.3. Binding interaction between Ru(II) arene complex and HSA
3.3.1. Binding forces. Binding forces between proteins and ligands, which contain hydrogen bonding, van der Waals forces, electrostatic interactions, and hydrophobic binding interactions, can be elucidated through the variation of thermodynamic parameters.35 When the enthalpy change (ΔH) keeps constant in a narrow temperature range, the values of ΔH, entropy change (ΔS) and free energy change (ΔG) can be calculated from the following equations:28 |
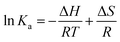 | (3) |
Herein, Ka is the associative binding constant at the corresponding temperature T and R is the gas constant. Plots between ln
Ka and 1/T of the three HSA–drug systems at three different temperatures are shown in Fig. S4,† and the calculated values of the three thermodynamic parameters are listed in Table 1. As indicated in Table 1, negative values of ΔG suggest that the binding processes between the three drugs and HSA are spontaneous. Moreover, negative values of ΔH indicate that the binding interactions of the three HSA–drug systems are exothermic.36 The values of both ΔH and ΔS are negative for these drugs, heralding that the binding interactions between the three drugs and HSA are mainly driven by van der Waals forces and hydrogen bonding.36
3.3.3. Binding numbers. UPLC-MS can be utilized to certify the binding interaction of ligands with HSA.39 In order to illustrate the detailed binding interaction between the three Ru(II) arene complexes and HSA, mass spectra of HSA and three drugs were taken and are depicted in Fig. S6.† The average molecular weight of HSA is found to be around 66
557 Da, which is in accordance with the reported value.13,39 It is easy to know that the precise molecular weights of Ru-1 and Ru-2 were 612.16 g mol−1 and 567.56 g mol−1, respectively, according to the molecular formula. However, in the positive ion spectrum, the main fragment ions of two complexes are observed at m/z 576.90 and m/z 496.04; these corresponded with the molecular weights of the structures of [M − Cl]+ and [M − Cl − HCl]+.7,13 In addition, two main fragment ions of Ru-3 are observed at m/z 496.04 (positive ion spectrum) and m/z 340.92 (negative ion spectrum); these corresponded with the molecular weights of the structures of [M − Cl − HCl]+ and [M]−, respectively.6,7Fig. 5 shows the mass spectra of the three HSA–drug systems with molar ratios of HSA to the three drugs being 1
:
1 and 1
:
2, respectively. It is very obvious that the same molecular weights of around 66
557 Da appear after the binding interactions of HSA with the three drugs. New absorption peaks corresponding with different molecular weights occur at about 67
134 Da, 67
053 Da, and 67
394 Da, and the molecular weights increase by around 576 Da, 496 Da and 837 Da compared with the molecular mass of HSA, indicating the ground state complex formation of the three drugs with HSA. As illustrated continuously in Fig. 5A and B, compared with the mass spectrum of HSA, almost the same average molecular weights of around 67
134 Da are present in the HSA–Ru-1 system with two different molar ratios of Ru-1 versus HSA, indicating that the additional molecular weight on HSA originates from one Ru-1 molecule and the binding number of Ru-1 with HSA is 1
:
1. The same changing trends exist in the HSA–Ru-2 system and HSA–Ru-3 system with two different molar ratios (Fig. 5C–F), revealing that the binding numbers of the three drugs to HSA are all near 1
:
1, which is highly consistent with the reported results previously.12,13
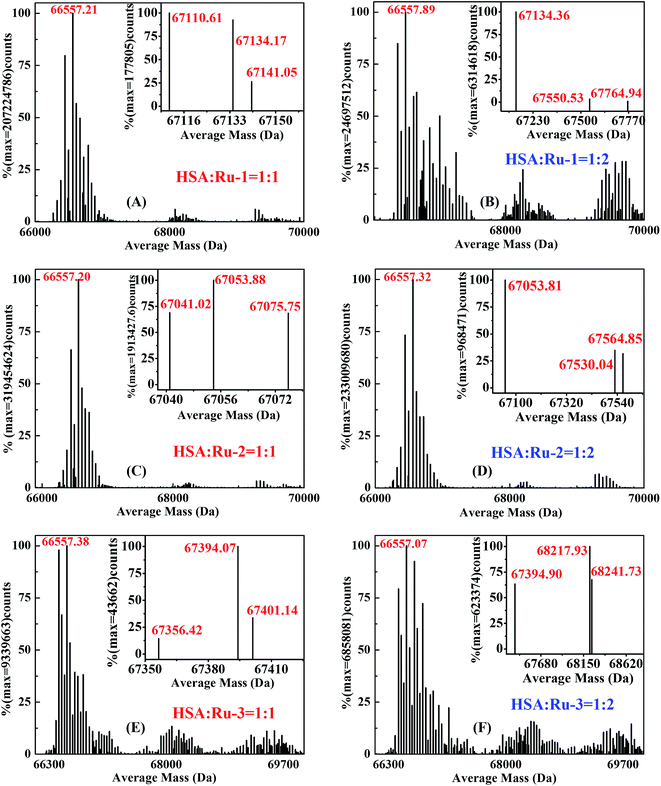 |
| Fig. 5 Mass spectra of the three HSA–drug systems with the molar ratio of HSA to the three drugs being 1 : 1 and 1 : 2, respectively. | |
3.4. Micro-environmental and conformational change investigation
3.4.1. Three-dimensional fluorescence spectrometry. The changes of micro-environments and conformations of protein influenced by ligands can be revealed by three-dimensional fluorescence spectrometry.40 Three-dimensional fluorescence spectra of HSA and the three HSA–drug systems are illustrated in Fig. S7† and fluorescence peak information is summarized in Table 3. The strong fluorescence peak 1 reveals the fluorescent characteristic of a single tryptophan residue of HSA, while the fluorescence peak 2 corresponds to the polypeptide backbone structure of HSA, respectively.41 When the three drugs are present, the fluorescence intensity decrements of both peak 1 and peak 2 at different levels suggest that the binding interactions of the three drugs with HSA lead to the variations of both the polar environment of a single tryptophan residue and the polypeptide backbone structure of HSA.39 The order of the decrease in the two fluorescence intensities among the studied drugs is as follows: Ru-3 > Ru-1 > Ru-2, indicating that the addition of Ru-3 can induce most the hydrophobic tryptophan residue to be buried in the hydrophobic pocket of HSA.41
Table 3 Three-dimensional fluorescence spectral characteristics of HSA and the three HSA–drug systems
Fluorescence peaks |
HSA |
HSA–Ru-1 system |
HSA–Ru-2 system |
HSA–Ru-3 system |
Peak position λex/em (nm nm−1) |
Intensity F (a.u.) |
Peak position λex/em (nm nm−1) |
Intensity F (a.u.) |
Peak position λex/em (nm nm−1) |
Intensity F (a.u.) |
Peak position λex/em (nm nm−1) |
Intensity F (a.u.) |
Peak 1 |
290.0/347.3 |
805 |
290.0/345.1 |
559 |
290.0/348.7 |
615 |
290.0/343.3 |
534 |
Peak 2 |
235.0/345.2 |
498 |
235.0/345.5 |
350 |
235.0/345.5 |
401 |
235.0/346.3 |
340 |
3.4.2. FT-IR spectrometry. FT-IR spectrometry is widely used to analyze the micro-environmental and conformational variation of protein under the influence of ligands. The FT-IR spectrum of HSA, and the difference absorption spectra between the three HSA–drug systems and their corresponding drug are shown in Fig. 6A. As previously reported, HSA exhibits two amide bands, an amide I band mainly at 1653 cm−1 ascribed to the C
O stretch vibration, and an amide II band mainly at 1548 cm−1 due to C–N stretch and N–H bending vibrations.40 Furthermore, after the addition of the three drugs, the peak positions of the amide I band red-shift from 1653.20 cm−1 to 1658.48 cm−1 (Ru-1), 1657.80 cm−1 (Ru-2), and 1658.55 cm−1 (Ru-3), respectively, which suggests that the C
O stretch vibration of HSA is affected by the three drugs to varying degrees. However, when the three drugs are present, the peak positions of the amide II band blue-shift from 1547.96 cm−1 to 1544.38 cm−1 (Ru-1), 1545.14 cm−1 (Ru-2), and 1544.55 cm−1 (Ru-3), respectively. The amide II band is less sensitive to the structure variation of HSA than the amide I band, so the C–N stretch and N–H bending vibrations are less influenced by the three drugs. On the whole, the order of these variations among the three drugs is as follows: Ru-3 ≥ Ru-1 > Ru-2, implying that Ru-3 and Ru-1 exhibit greater impacts on the micro-environmental and conformational change of HSA than Ru-2 does, which agrees well with the results exhibited in the above experiments.
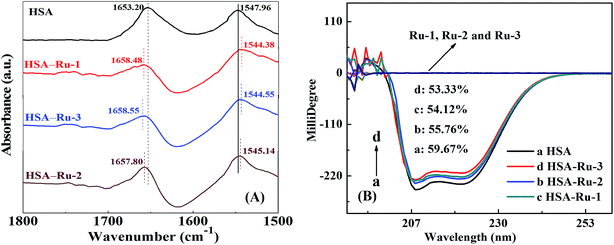 |
| Fig. 6 (A) FT-IR spectrum of HSA, and difference absorption spectra between the three HSA–drug systems and their corresponding drug. c (HSA) = c (Ru-1) = c (Ru-2) = c (Ru-3) = 1.0 × 10−3 mol L−1. (B) CD spectra of HSA (a) and the three Ru(II) arene complexes. Difference spectrum between the HSA–Ru-2 system and Ru-2 (b), difference spectrum between the HSA–Ru-1 system and Ru-1 (c), and difference spectrum between the HSA–Ru-3 system and Ru-3 (d). c (HSA) = 2.0 × 10−6 mol L−1; c (Ru-1) = c (Ru-2) = c (Ru-3) = 2.0 × 10−5 mol L−1. | |
3.4.3. CD spectrometry. The changes of micro-environments and conformations of protein affected by ligands can be illustrated through CD spectrometry by comparing the contents of its secondary structures. CD spectra of HSA and the three drugs are shown in Fig. 6B. As is consistent with the reported results, HSA exhibits two negative absorption bands at 208 nm and 222 nm which are characteristic absorption peaks of its α-helical structure.38 However, the three drugs have almost no absorption during the same wavelength range. When the three drugs are added into HSA solution, the absorbance at two bands all decreases, suggesting that the binding interactions of the three drugs with HSA result in the reduction of the typical α-helical content of HSA. A detailed value of the typical α-helical content of HSA can be calculated according to the following equations:42 |
 | (5) |
|
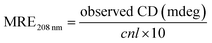 | (6) |
Herein, MRE208 is the mean residue ellipticity at 208 nm, c is the concentration of HSA, n is the number of amino acid residues for HSA (n = 585), and l is the path length (1.0 cm), respectively. As shown in Fig. 6B, the α-helical content of HSA decreases from 59.67% to 54.12% (Ru-1), 55.76% (Ru-2), and 53.33% (Ru-3), respectively, suggesting remarkable structural variations of those related to lower degrees of surface coverage after interactions with the three drugs.43 Thermal unfolding analysis demonstrates that the melting temperature (Tm) of HSA decreases from 68.9 ± 0.3 °C to 59.3 ± 0.1 °C (Ru-1), 66.3 ± 0.1 °C (Ru-2), and 58.9 ± 0.1 °C (Ru-3), respectively, further proving the decreased stability of HSA after binding with the three drugs.44,45 The α-helical content and the stability of the protein are correlated closely with its biological functions,46 and the largest degradation of the α-helical content and lowest Tm value of HSA are present in the HSA–Ru-3 system, indicating the biggest reduction in biological activity of HSA by Ru-3 among the investigated three drugs. These results agree nicely with the results obtained from the above experiments.
4. Conclusions
In this work, we systematically investigated the in vitro interactions between three Ru(II) arene complexes and HSA. Three drugs can statically quench the intrinsic fluorescence of HSA effectively through a concentration-dependent relationship. Among the three drugs, Ru-3 quenches the intrinsic fluorescence of HSA more efficiently and binds with HSA more strongly. Van der Waals forces and hydrogen bonding play major roles during the three HSA–drug complex formations. The changes of micro-environments and conformations of HSA are more significant in the presence of a drug with more Ru(II) arene groups and less space steric hindrance, indicating that the biological activity of HSA is weakened in the presence of this Ru(II) arene complex. These results give a better understanding of the structural influences on the in vitro interactions of HSA with the Ru(II) arene complex from a molecular biology level, which is important for the further applications of Ru(II) arene complex-based drugs in biomedical fields.
Acknowledgements
This work was financially supported by the National Natural Science Foundation of China (21563006, 21403039, 21261005), the Guangxi Natural Science Foundation (2013GXNSFCA019005, 2015GXNSFAA139033), and Guangxi Colleges and Universities Key Laboratory of Synthetic and Natural Functional Molecular Chemistry, Guangxi Teachers Education University.
References
- T. Sriskandakumar, S. Behyan, A. Habtemariam, P. J. Sadler and P. Kennepohl, Electrophilic activation of oxidized sulfur ligands and implications for the biological activity of ruthenium(II) arene anticancer complexes, Inorg. Chem., 2015, 54, 11574–11580 CrossRef CAS PubMed.
- M. Martínez-Alonso, N. Busto, F. A. Jalón, B. R. Manzano, J. M. Leal, A. M. Rodríguez, B. García and G. Espino, Derivation of structure–activity relationships from the anticancer properties of ruthenium(II) arene complexes with 2-aryldiazole ligands, Inorg. Chem., 2014, 53, 11274–11288 CrossRef PubMed.
- F. A. Beckford, D. Dourth, M. Shaloski Jr, J. Didion, J. Thessing, J. Woods, V. Crowell, N. Gerasimchuk, A. Gonzalez-Sarrías and N. P. Seeram, Half-sandwich ruthenium–arene complexes with thiosemicarbazones: synthesis and biological evaluation of [(η6-p-cymene)Ru(piperonal thiosemicarbazones)Cl]Cl complexes, J. Inorg. Biochem., 2011, 105, 1019–1029 CrossRef CAS PubMed.
- T. Stringer, B. Therrien, D. T. Hendricks, H. Guzgay and G. S. Smith, Mono- and dinuclear (η6-arene) ruthenium(II) benzaldehyde thiosemicarbazone complexes: synthesis, characterization and cytotoxicity, Inorg. Chem. Commun., 2011, 14, 956–960 CrossRef CAS.
- R. Pettinari, F. Marchetti, F. Condello, C. Pettinari, G. Lupidi, R. Scopelliti, S. Mukhopadhyay, T. Riedel and P. J. Dyson, Ruthenium(II)–arene RAPTA type complexes containing curcumin and bisdemethoxycurcumin display potent and selective anticancer activity, Organometallics, 2014, 33, 3709–3715 CrossRef CAS.
- W. Su, Q. Q. Qian, P. Y. Li, X. L. Lei, Q. Xiao, S. Huang, C. S. Huang and J. G. Cui, Synthesis, characterization, and anticancer activity of a series of ketone-N4-substituted thiosemicarbazones and their ruthenium(II) arene complexes, Inorg. Chem., 2013, 52, 12440–12449 CrossRef CAS PubMed.
- W. Su, Z. F. Tang, Q. Xiao, P. Y. Li, Q. Q. Qian, X. L. Lei, S. Huang, B. H. Peng, J. G. Cui and C. S. Huang, Synthesis, structures, antiproliferative activity of a series of ruthenium(II) arene derivatives of thiosemicarbazones ligands, J. Organomet. Chem., 2015, 783, 10–16 CrossRef CAS.
- B. Tu, Z. J. Liu, Z. F. Chen, Y. Ouyang and Y. J. Hu, Understanding the structure–activity relationship between quercetin and naringenin: in vitro, RSC Adv., 2015, 5, 106171–106181 RSC.
- Y. R. Zheng, K. Suntharalingam, T. C. Johnstone, H. Yoo, W. Lin, J. G. Brooks and S. J. Lippard, Pt(IV) prodrugs designed to bind non-covalently to human serum albumin for drug delivery, J. Am. Chem. Soc., 2014, 136, 8790–8798 CrossRef CAS PubMed.
- B. Tu, Z. F. Chen, Z. J. Liu, R. R. Li, Y. Ouyang and Y. J. Hu, Study of the structure–activity relationship of flavonoids based on their interaction with human serum albumin, RSC Adv., 2015, 5, 73290–73300 RSC.
- A. Martínez, J. Suárez, T. Shand, R. S. Magliozzo and R. A. Sánchez-Delgado, Interactions of arene–Ru(II)–chloroquine complexes of known antimalarial and antitumor activity with human serum albumin (HSA) and transferrin, J. Inorg. Biochem., 2011, 105, 39–45 CrossRef PubMed.
- S. Huang, S. S. Peng, F. W. Zhu, X. L. Lei, Q. Xiao, W. Su, Y. Liu, C. S. Huang and L. X. Zhang, Multispectroscopic investigation of the interaction between two ruthenium(II) arene complexes of curcumin analogs and human serum albumin, Biol. Trace Elem. Res., 2016, 169, 189–203 CrossRef CAS PubMed.
- S. Huang, F. W. Zhu, Q. Xiao, Q. Zhou, W. Su, H. N. Qiu, B. Q. Hu, J. R. Sheng and C. S. Huang, Combined spectroscopy and cyclic voltammetry investigates the interaction between [(η6-p-cymene)Ru(benzaldehyde-N(4)-phenylthiosemicarbazone)Cl]Cl anticancer drug and human serum albumin, RSC Adv., 2014, 4, 36286–36300 RSC.
- S. Huang, F. W. Zhu, Q. Xiao, Q. Zhou, W. Su, H. N. Qiu, B. Q. Hu, J. R. Sheng and C. S. Huang, Thermodynamic investigation of interaction between [(η6-p-cymene)RuII(acetone-N4-phenylthiosemicarbazone)Cl]Cl anticancer drug and human serum albumin: spectroscopic and electrochemical studies, Biol. Trace Elem. Res., 2015, 164, 150–161 CrossRef CAS PubMed.
- S. Tunç, O. Duman, İ. Soylu and B. K. Bozoğlan, Spectroscopic investigation of the interactions of carbofuran and amitrol herbicides with human serum albumin, J. Lumin., 2014, 151, 22–28 CrossRef.
- S. Tunç, A. Çetinkaya and O. Duman, Spectroscopic investigations of the interactions of tramadol hydrochloride and 5-azacytidine drugs with human serum albumin and human hemoglobin proteins, J. Photochem. Photobiol., B, 2013, 120, 59–65 CrossRef PubMed.
- S. Tunç, O. Duman and B. K. Bozoğlan, Studies on the interactions of chloroquine diphosphate and phenelzine sulfate drugs with human serum albumin and human hemoglobin proteins by spectroscopic techniques, J. Lumin., 2013, 140, 87–94 CrossRef.
- J. Zhang, H. H. Sun, Y. Z. Zhang, L. Y. Yang, J. Dai and Y. Liu, Interaction of human serum albumin with indomethacin: spectroscopic and molecular modeling studies, J. Solution Chem., 2012, 41, 422–435 CrossRef CAS.
- S. Tunç, O. Duman, İ. Soylu and B. K. Bozoğlan, Study on the bindings of dichlorprop and diquat dibromide herbicides to human serum albumin by spectroscopic methods, J. Hazard. Mater., 2014, 273, 36–43 CrossRef PubMed.
- M. Tanaka, Y. Asahi and S. Masuda, Interaction between drugs and water-soluble polymers. VII. Binding of berberine with bovine serum albumin, J. Macromol. Sci., Part A: Pure Appl. Chem., 1995, 32, 339–347 CrossRef.
- E. Ayranci and O. Duman, Binding of fluoride, bromide and iodide to bovine serum albumin, studied with ion-selective electrodes, Food Chem., 2004, 84, 539–543 CrossRef CAS.
- A. D. Bani-Yaseen, Spectrofluorimetric study on the interaction between antimicrobial drug sulfamethazine and bovine serum albumin, J. Lumin., 2011, 131, 1042–1047 CrossRef.
- B. K. Bozoğlan, S. Tunç and O. Duman, Investigation of neohesperidin dihydrochalcone binding to human serum albumin by spectroscopic methods, J. Lumin., 2014, 155, 198–204 CrossRef.
- G. J. Zhang, B. Keita, C. T. Craescu, S. Miron, P. D. Oliveira and L. Nadjo, Polyoxometalate binding to human serum albumin: a thermodynamic and spectroscopic approach, J. Phys. Chem. B, 2007, 111, 11253–11259 CrossRef CAS PubMed.
- A. C. Dong, P. Huang and W. S. Caughey, Protein secondary structures in water from second-derivative amide I infrared spectra, Biochemistry, 1990, 29, 3303–3308 CrossRef CAS PubMed.
- Y. Zhang and Q. X. Zhong, Encapsulation of bixin in sodium caseinate to deliver the colorant in transparent dispersions, Food Hydrocolloids, 2013, 33, 1–9 CrossRef CAS.
- B. Tu, Z. F. Chen, Z. J. Liu, L. Y. Cheng and Y. J. Hu, Interaction of flavones with DNA in vitro: structure–activity relationships, RSC Adv., 2015, 5, 33058–33066 RSC.
- J. R. Lakowicz, Principles of fluorescence spectroscopy, Springer, New York, 3rd edn, 2006 Search PubMed.
- D. Y. Gao, Z. H. Sheng and H. Y. Han, A novel method for the analysis of calf thymus DNA based on CdTe quantum dots–Ru(bpy)32+ photoinduced electron transfer system, Microchim. Acta, 2010, 168, 341–345 CrossRef CAS.
- S. Huang, F. W. Zhu, H. N. Qiu, Q. Xiao, Q. Zhou, W. Su and B. Q. Hu, A sensitive quantum dots-based “OFF–ON” fluorescent sensor for ruthenium anticancer drugs and ctDNA, Colloids Surf., B, 2014, 117, 240–247 CrossRef CAS PubMed.
- R. M. Watt and E. W. Voss, Solvent perturbation of the fluorescence of fluorescein bound to specific antibody, J. Biol. Chem., 1979, 254, 1684–1690 CAS.
- F. Sheng, Y. N. Wang, X. C. Zhao, N. Tian, H. L. Hu and P. X. Li, Separation and identification of anthocyanin extracted from mulberry fruit and the pigment binding properties toward human serum albumin, J. Agric. Food Chem., 2014, 62, 6813–6819 CrossRef CAS PubMed.
- M. A. Jhonsi, A. Kathiravan and R. Renganathan, Spectroscopic studies on the interaction of colloidal capped CdS nanoparticles with bovine serum albumin, Colloids Surf., B, 2009, 72, 167–172 CrossRef PubMed.
- A. Orte, J. M. Alvarez-Pez and M. J. Ruedas-Rama, Fluorescence lifetime imaging microscopy for the detection of intracellular pH with quantum dot nanosensors, ACS Nano, 2013, 7, 6387–6395 CrossRef CAS PubMed.
- D. Leckband, Measuring the forces that control protein interactions, Annu. Rev. Biophys. Biomol. Struct., 2000, 29, 1–26 CrossRef CAS PubMed.
- D. P. Ross and S. Subramanian, Thermodynamics of protein association reactions: forces contributing to stability, Biochemistry, 1981, 20, 3096–3102 CrossRef PubMed.
- D. E. Epps, T. J. Raub and F. J. Kézdy, A general wide-range spectrofluorometric method for measuring the site-specific affinities of drugs toward human serum albumin, Anal. Biochem., 1995, 227, 342–350 CrossRef CAS PubMed.
- Y. S. Ge, C. Jin, Z. Song, J. Q. Zhang, F. L. Jiang and Y. Liu, Multi-spectroscopic analysis and molecular modeling on the interaction of curcumin and its derivatives with human serum albumin: a comparative study, Spectrochim. Acta, Part A, 2014, 124, 265–276 CrossRef CAS PubMed.
- Z. Y. Chen, H. Y. Xu, Y. L. Zhu, J. Y. Liu, K. Y. Wang, P. X. Wang, S. J. Shang, X. N. Li, Z. L. Wang, W. Shao and S. D. Zhang, Understanding the fate of an anesthetic, nalorphine upon interaction with human serum albumin: a photophysical and mass-spectroscopy approach, RSC Adv., 2014, 4, 25410–25419 RSC.
- S. Huang, H. N. Qiu, Y. Liu, C. S. Huang, J. R. Sheng, W. Su and Q. Xiao, Molecular interaction investigation between three CdTe:Zn2+ quantum dots and human serum albumin: a comparative study, Colloids Surf., B, 2015, 136, 955–962 CrossRef CAS PubMed.
- S. Huang, H. N. Qiu, S. Y. Lu, F. W. Zhu and Q. Xiao, Study on the molecular interaction of graphene quantum dots with human serum albumin: combined spectroscopic and electrochemical approaches, J. Hazard. Mater., 2015, 285, 18–26 CrossRef CAS PubMed.
- Z. X. Lu, T. Cui and Q. L. Shi, Application of circular dichroism and optical rotatory dispersion in molecular biology, Science Press, Beijing, 1st edn, 1987, p. 79 Search PubMed.
- S. Li, Y. Z. Wang, J. G. Jiang and S. J. Dong, pH-Dependent protein conformational changes in albumin:gold nanoparticle bioconjugates: a spectroscopic study, Langmuir, 2007, 23, 2714–2721 CrossRef PubMed.
- K. Chattopadhyay and S. Mazumdar, Structural and conformational stability of horseradish peroxidase: effect of temperature and pH, Biochemistry, 2000, 39, 263–270 CrossRef CAS PubMed.
- W. M. Rabeh, F. Bossard, H. J. Xu, T. Okiyoneda, M. Bagdany, C. M. Mulvihill, K. Du, K. di Bernardo, Y. H. Liu, L. Konermann, A. Roldan and G. L. Lukacs, Correction of both NBD1 energetics and domain interface is required to restore DF508 CFTR folding and function, Cell, 2012, 148, 150–163 CrossRef CAS PubMed.
- P. Koegler, A. Clayton, H. Thissen, G. N. C. Santos and P. Kingshott, The influence of nanostructured materials on biointerfacial interactions, Adv. Drug Delivery Rev., 2012, 64, 1820–1839 CrossRef CAS PubMed.
Footnote |
† Electronic supplementary information (ESI) available. See DOI: 10.1039/c6ra05886h |
|
This journal is © The Royal Society of Chemistry 2016 |