DOI:
10.1039/C6RA05699G
(Paper)
RSC Adv., 2016,
6, 44599-44605
High contrast off–on fluorescence photo-switching via copper ion recognition, trans–cis isomerization and ring closure of a thiosemicarbazide Schiff base†
Received
3rd March 2016
, Accepted 27th April 2016
First published on 28th April 2016
Abstract
An easily available triphenylamine–isophorone-based Schiff base was synthesized and its specifically triple-addressable molecular photo-switching behaviour via copper ion recognition, trans–cis isomerization and ring closure were investigated in detail. Firstly, compound Lo was able to real-time monitor Cu2+ in an acetonitrile solution via displaying the “turn-on” emission in the near infrared region with high selectivity, and sensitivity. Furthermore, two independently photochromic phenomena based on the distinct mechanisms were found surprisingly, one through the ring closure of the thiosemicarbazide moiety based on a Michael addition reaction in tetrahydrofuran solution and another through the trans–cis isomerization of aldimine (–C
N–) in the solid state under the stimuli of UV/visible light. Finally, fluorescence imaging experiments in living cells demonstrated the potential practical applications in biological systems.
Introduction
As the third most abundant (after Fe3+ and Zn2+) essential metal ion in the human body, Cu2+ ion plays a pivotal role in various fundamental physiological processes.1 However, overloaded levels of Cu2+ can cause oxidative stress and disorders associated with neurodegenerative diseases, and the Cu2+ ion is also identified as an environmental pollutant, which is potentially very toxic to organisms.2 Consequently, the development of high-performance fluorescent probes for the determination and visualization of Cu2+ ion has received considerable attention because of high selectivity, sensitivity, specificity, a low detection limit and real-time monitoring with fast response.3
Photochromic molecules are better known by the possibility of achieving reversible changes in the color upon light absorption. Moreover, they may also find many other applications such as switches,4 optical memory devices,5 sensors,6 or holographic gratings.7 Two main mechanisms to achieve photochromism are the cis–trans isomerization and the opening-closure ring, azobenzenes8 or stilbenes9 and diarylethenes10 or spiropyrans,11 respectively, often reported in literature. However, instances of turn-on fluorescence of other photochromic systems upon irradiation with UV/visible light are rather rare. In a previous work,12 we reported that a triphenylamine Schiff base having the diaminomaleonitrile unit exhibited highly sensitivity and selectivity for Cu2+ ion by the ring-closing of diaminomaleonitrile moiety based on Michael addition reaction and coordination interaction. Malkondu reported13 a novel triphenylamine based semicarbazone Schiff base TOC, and exhibited fluorometric ‘turn-on’ response towards Cu2+ via a unique cyclization reaction. The preliminary work have contributed to expanding the kinds of photochromic molecular and further help us to understand their photochromic mechanisms.
In this communication, a new thiosemicarbazide based photochromic Schiff base Lo was successfully synthesized and its specifically dual-addressable molecular photo-switching behaviour of interacting with Cu2+ ion and light (UV/visible) were investigated in detail. The results will be helpful for synthesizing the efficient photoactive thiosemicarbazide derivatives with multi-addressable states and understanding the information processing on the unimolecular platform with potential applications in fluorescent chemosensors, data manipulation, as well as intelligent diagnostics.
Results and discussion
The Schiff base compound Lo was synthesized by aldol condensation and affinity addition–elimination reaction. The synthetic details are presented in Scheme 1. The intermediate 0 reacted with intermediate 1, and underwent a classical aldol condensation to form intermediate 2. Furthermore, a simple condensation of the intermediate 2 with compound b in anhydrous methanol gave a Schiff base Lo. They were characterized by 1H NMR, 13C NMR spectrum, mass spectrum, FT-IR and elemental analysis. For Lo, the presence of a singlet at 8.16 ppm corresponds to the aldimine proton (–HC
N–), which is further testified by 1687 cm−1 in IR spectrum. The singlet at 8.01 ppm corresponds to the amido (–NH2), which is tested by 3413 cm−1 in IR spectrum. The singlet at 7.62 ppm stands for –H2C
CH2– proton, which is obtained at 831 cm−1 in the IR spectrum.
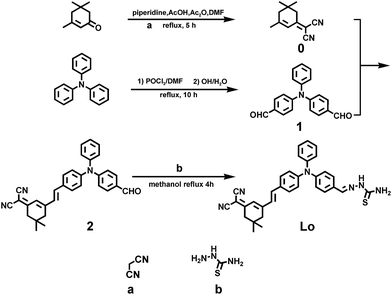 |
| Scheme 1 Synthesis route of compound Lo. | |
The compound Lo was soluble in acetone, acetonitrile, DMF and DMSO, tetrahydrofuran, dichloromethane and chloroform. It was partially soluble in water, methanol, ethanol. The photo/solution stability of compound Lo was thus monitored by UV-visible spectroscopy in acetonitrile (under visible light) and tetrahydrofuran (under dark light). And the absorption spectral of compound Lo shows no apparent spectral change even after 72 hours (Fig. S1, ESI†).
Recognition ability toward metal ions
With that, we evaluated its spectral properties in the presence of some environmentally or physiologically important metal ions in the CH3CN solution. As shown in Fig. 1a, Cu2+ ion induced significant change in the emission spectra, whereas no distinct change was observed upon the addition of other ions. Consistent with the fluorescent enhancement, an apparent color change from dull-red to bright-red was observed by naked-eye under a UV lamp of 365 nm (Fig. 1a inset). Also, the fluorescence enhancement upon addition of Cu2+ ion almost remained unchanged even after adding large amounts of other competitive metal ions (Fig. S2, ESI†). This result indicated the high selectivity of probe Lo for Cu2+ over other competitive metal ions in acetonitrile solution. As evident from Fig. 1b, the fluorescence emission centred at 631 nm gradually increased along with the increasing amount of Cu2+ and when the concentration of Cu2+ ions reached 2.0 equivalent, a maximal fluorescence enhancement (31 fold) was observed. This fluorescence enhancement in the NIR region is superior to the known fluorescent Cu2+ probes with emission only in the visible region. Obviously, the stoichiometry showed 1
:
2 for the Lo–Cu2+ complexation (Fig. 1b, inset), which was further confirmed by Job plot analysis (Fig. S3, ESI†).14 Based on fluorescence titration data, the binding constant15 of Lo with Cu2+ in acetonitrile (Fig. S4a, ESI†) was found to be 2.076 × 104 M−1. Fluorometric titration data was also used to obtain the detection limits of Lo for Cu2+. The detection limits of Lo was found to be 4.275 × 10−7 M for Cu2+ (Fig. S4b, ESI†) based on the equation DL = K × SD/S, where K = 3, SD is the standard deviation of the blank solution, and S is the slope of the calibration curve,16,17 which is much lower than the limit of copper (∼20 μM) in drinking water by the U.S. Environmental Protection Agency,18 indicating that it could quantify Cu2+ in lower than micromolar level. Further, the pH titration (Fig. S5, ESI†), the reversibility (Fig. S6, ESI†) and the response time experiment (Fig. S7, ESI†) were also carried out. All results demonstrated that Lo was able to real-time monitor Cu2+ via displaying the “turn-on” emission in the NIR region with high selectivity, sensitivity.
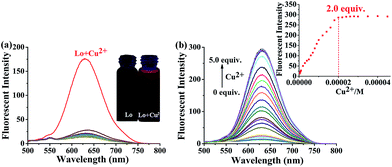 |
| Fig. 1 (a) Fluorescence spectra of Lo (10 μM) in CH3CN in the absence and presence of different metal ions (10 μM). Inset: color of Lo and Lo + Cu2+ system under UV lamp at 365 nm; (b) fluorescence spectra of Lo (10 μM) upon the addition of Cu2+ (0–5.0 equivalent). Inset: fluorescence intensity changes at 631 nm of Lo (10 μM) with the equiv. of Cu2+ ions. | |
The favorable features of the probe Lo include emission in the NIR region, a significant fluorescence turn-on signal, high sensitivity, high selectivity, and functioning well at physiological pH. These desirable characters prompted us to evaluate the ability of the probe to image Cu2+ in living cells. As shown in Fig. 2, HepG2 cells treated with 10 μM of Lo exhibited the ignorable emission, however, once incubated with the solution of Cu(NO3)2 (100 μM) for 0.5 h, the whole cell displayed remarkable fluorescence enhancement in the cell cytoplasm areas. At the same time, to evaluate cytotoxicity of the probe, Lo was taken as an example to perform a 3-(4,5-dimethylthiazol-2-yl)-2,5-diphenyltetrazolium bromide (MTT) assay on HepG2 cells with the concentration from 0 μM to 40 μM. The cellular viability estimated was ca. 91.2% in 24 h after treatment with 40 μM of Lo, exhibiting low toxicity to cultured cells (Fig. S8, ESI†). Furthermore, good photostability of Lo was also evaluated by photon-bleach experiment (Fig. S9, ESI†). These data indicate that the probe Lo can penetrate the cell membrane and sense exogenous Cu2+ in the living cells effectively in a non-invasiveness way, with the switching-on fluorescent signal.
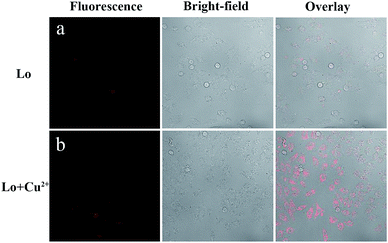 |
| Fig. 2 Fluorescence microscopy of HepG2 cells for (a) cells treated with 10 μM of Lo; (b) treated with 100 μM of Cu2+ to (a). | |
Photochromic reaction
Photochromic reaction under solution
Unexpectedly, the photochromic behavior of compound Lo in THF and the others solvents (Fig. S10, Table S1, ESI†) at room temperature was discovered. Immediately following this find, the ring closure photochromic process from Lo (open-ring isomer) to Lc (closed-ring isomer) after irradiation with visible light was demonstrated by 1H NMR.19 As illustrated in Fig. 3, as the growth of the irradiation time, the signals of aldimine protons (Hb) and amidogen protons (Ha) gradually disappeared. Meanwhile, the new signal assignable to para amide (Hc) appeared at 2.280 ppm became more and more obvious. Moreover, by comparing the MALDI-TOF mass spectrometry (Fig. S11, ESI†), we found that the original peak at m/z 542.227 for free Lo disappeared and a new peak at m/z 540.202 for the corresponding closed-ring compound Lc emerged after irradiation with visible light. Overall results indicated that Lo underwent a closure-ring process after irradiation with visible light. Furthermore, we began to research their ultraviolet and fluorescence spectral properties. In Fig. 4, UV-visible spectrum of Lo (10 μM) displayed absorption bands at 484, 368 nm, under visible light irradiation (10 min, 100 μM), this orange solution turned to colorless and the absorption of the open-ring isomer at a shorter wavelength (368 nm) decreased gradually while a longer wavelength (484 nm) vanished, eventually formed the closed-ring isomer. Meanwhile, the original fluorescent band at 620 nm (red color) disappeared and a new band centred on 431 nm (blue color) was observed and its intensity increased with the increasing of irradiation time (Fig. S12, ESI†). Furthermore, compared with Lo, Lc displayed 36 fold higher quantum yields and fluorescence lifetimes (Fig. S13, Table S2, ESI†).
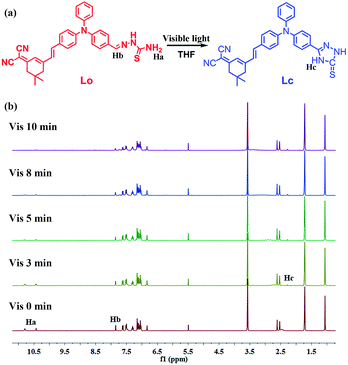 |
| Fig. 3 (a) Changes in chemical structure of Lo under the alternative irradiation with visible light in THF; (b) changes in 1H NMR spectrum of Lo under the alternative irradiation with visible light in THF. | |
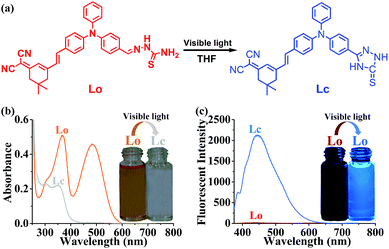 |
| Fig. 4 (a) Changes in chemical structure of Lo under the alternative irradiation with visible light in THF; absorption (b) and fluorescent (c) spectra of Lo in THF solution before and after visible irradiation. | |
The above described properties inspired us to investigate photochromism in living cells. Herein, live cells treated with the opening form of compound Lo initially showed a red fluorescence signal, but after 30 min of visible light irradiation, a substantial cytoplasmic blue fluorescence signal appeared, suggesting a conversion of Lo into the closed-form isomer Lc in vitro, as shown in Fig. 5.
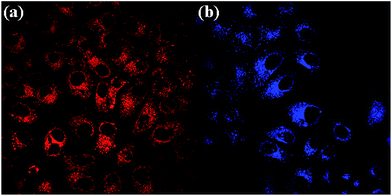 |
| Fig. 5 (a) The confocal laser scanning microscope image of HepG2 cells incubated with Lo (100 μM) for 20 min at 25 °C (a) in original state, (b) irradiated by 405 nm light for 30 min. | |
In order to explored the cell viability after long photo-exposure time (Fig. S14, ESI†). One reliable way to assess the in vitro anticancer activity of a compound is to evaluate its cytotoxicity in terms of its half maximal inhibitory concentration (IC50) value in cancer cells by using the MTT (3-(4,5-dimethylthiazol-2-yl)-2,5-diphenyltetrazolium bromide) assay.20 Therefore, the anticancer activity of the compound Lo was evaluated by the MTT assay in HepG2 cells in the presence of visible light (400–700 nm, 10 J cm−2) for 0.5 h, giving IC50 values of 70 μM, and >105 μM in the absence of light, which indicated the compound Lo had not significant toxicity in HepG2 cells under visible light.
The dual-colour photochromic property of Lo make it an ideal tool for bioimaging applications with either red or blue fluorescence.
Photochromic reaction under solid
In the past decade, a number of photochromic molecules show photoisomerization under the solid state have been proposed. However, the most of those molecules in solid state showed the same photochromic mechanism as solution state. Based on the abnormal cyclization in aqueous solution and the characteristics of Schiff bases tend to occur trans–cis isomerization in solid state, the design and development of photochromic molecules with distinct photochromic mechanisms in solid and solution state is a wide topic.
Excitingly, the distinct color change of the Lo under solid state was observed when it was exposed to appropriate light (Fig. 6a). Using the selective 365 nm light irradiation through an aluminum mask, the exposed regions turned gradually to red with 22 fold fluorescent enhancement at 690 nm, whereas the masked areas maintained the initial color, at the same time, it displayed 28 fold enhancement in fluorescence lifetimes and quantum yields (Fig. S15, Table S3, ESI†). On visible light illumination, the above process underwent a reversible reaction to the initial color. The 13C NMR result displayed that the chemical shift (–C
N–) happened to small change from 149.50 to 150.81 ppm and no changes for others (Fig. S16, ESI†), which exhibited this photoluminescence derived from the trans–cis isomerization.21 To gain more insight into the photophysical properties of trans–cis isomerization, the time-dependent density functional theory (TD-DFT) (B3LYP/6-31G(d)) calculations was also studied in Fig. 7. The energy gaps between HOMO and LUMO are 2.88 eV (Lo-trans) and 2.93 eV (Lo-cis). The Lo-trans is associated with lower energy than Lo-cis by 0.05 eV. Furthermore, experimentally Lo-trans and Lo-cis absorb at 569 and 551 nm while the theoretical calculation showed absorption maxima at λmax 564 nm (f = 0.8633) and λmax 543 nm (f = 0.7531), the calculated absorption peaks correlate very well with the experimental observed values, which also confirmed that the photochromic properties should originate from the Lo-trans to Lo-cis isomeric reaction from experimental one. At the same time, the corresponding DFT coordinates for the optimized structures of Lo-cis and Lo-trans were also put in Tables S4 and 5 (ESI†). The delightful photoluminescence properties of the target compound might be utilized for practical development of solid-state photonic materials and photo-switchable accessories.
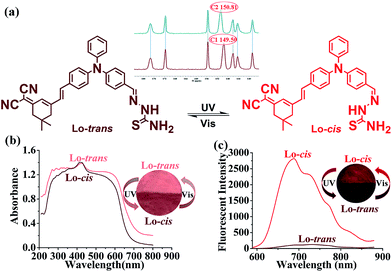 |
| Fig. 6 (a) Changes in chemical structure under the alternative irradiation with UV and visible light in solid state; absorption (b) and fluorescent spectra (c) of Lo-trans in solid state before and after UV irradiation. | |
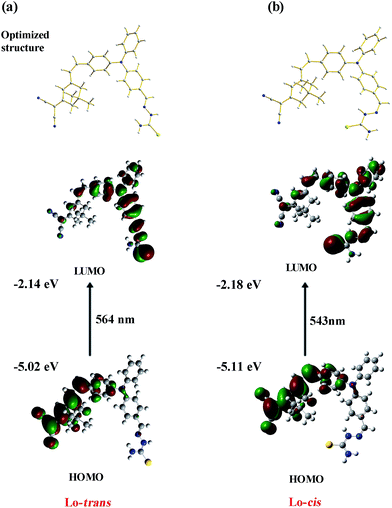 |
| Fig. 7 Optimized geometries, HOMO and LUMO distributions of Lo-trans (a) and Lo-cis (b). | |
Conclusion
In conclusion, as far as we know this is the first clear demonstration of thiosemicarbazide based Schiff base about photochromic behaviours with distinctive color changes in response to distinguish mechanisms, which happened to the ring closure in THF solution and trans–cis isomerization in solid state under the stimuli of UV/visible light. The dual photochromic compound is also showed excellent recognition ability toward Cu2+ in MeCN with high potential applications in sensing and labelling, as a consequence the system is able to respond to three stimuli. This example compared with many others reported literatures during the last few years shows the potentialities of this family of compounds, as versatile photochromic systems.
Experimental section
Materials and measurements
Unless otherwise mentioned, chemicals and solvents were purchased from commercially available resources and were used without further purification. 1H NMR and 13C NMR spectra were recorded on a Bruker AVANCE-400 MHz and 100 MHz, respectively and chemical shifts are expressed in ppm using TMS as an internal standard. The UV-vis absorption spectra were performed on a Shimadzu UV-265 spectrophotometer, and the fluorescence spectra were done using a Hitachi F-7000 fluorescence spectrophotometer with quartz cuvette (path length = 1 cm). IR spectra were recorded with a Nicolet FT-IR NEXUS 870 spectrometer (KBr discs) in the 4000–400 cm−1 region. The mass spectrum were recorded by AXIMA-CFR plus MALDI-TOF MS. The elemental analysis was performed by Perkin Elmer 240B analyzer. The fluorescent life and quantum yield were performed on a HORIBA FluoroMax-4P full-function steady transient fluorescence spectrometer. The pH titration was carried out by using a METTLER TOLEDO FE20 pH meter.
The solutions of metal ions were prepared from Pb(NO3)2, Co(NO3)2·6H2O, Zn(NO3)2·6H2O, Ca(NO3)2·4H2O, NaNO3, LiNO3, Cu(NO3)2·3H2O, Ni(NO3)2·6H2O, Bi(NO3)3·5H2O, KNO3, Ba(NO3)2, Al(NO3)3·9H2O, Cd(NO3)2·2H2O, AgNO3, Hg(NO3)2·0.5H2O, Cr(NO3)3·9H2O, Mg(NO3)2·6H2O, Fe(NO3)3·9H2O and Mn(NO3)2·4H2O.
Synthesis
Synthesis of compounds 0 and 1. Compounds 0 and 1 were prepared according to the literature.21
Synthesis of compound 2. Compound 0 (0.186 g, 1.0 mmol) and compound 1 (0.273 g, 1.0 mmol) were dissolved in acetonitrile (20 mL) under nitrogen. Piperidine (0.1 mL) was added as a catalyst and the mixture was reacted for 10 h at room temperature. The crude product was collected by filtration and purified by column chromatography (silica gel, petroleum ether/ethyl acetate = 25/1) to obtain red pure product. Yield: 60%. 1H NMR (400 MHz, DMSO-d6) δ (ppm) (Fig. S17, ESI†): 9.82 (s, 1H), 7.79–7.77 (d, J = 8.2 Hz, 2H), 7.71–7.69 (d, J = 8.1 Hz, 2H), 7.73–7.46 (t, J = 7.4 Hz, 2H), 7.38–7.34 (d, J = 16.2 Hz, 1H), 7.30–7.25 (m, 2H), 7.20–7.19 (d, J = 7.8 Hz, 2H), 7.13–7.11 (d, J = 7.8 Hz, 2H), 7.03–7.01 (d, J = 8.2 Hz, 2H), 6.87 (s, 1H), 2.62 (s, 2H), 2.55 (s, 2H), 1.08–0.97 (s, 6H). 13C NMR (100 MHz, CDCl3) δ (ppm) (Fig. S18, ESI†): 190.46, 169.13, 153.86, 152.57, 147.74, 145.78, 136.13, 131.42, 131.32, 130.26, 129.97, 128.86, 128.31, 126.64, 125.72, 124.90, 123.33, 122.14, 121.17, 78.31, 43.00, 39.24, 32.05, 28.04. MS (MALDI-TOF) (Fig. S19, ESI†): m/z 468.223 [(M − 1)−, calcd 468.215].
Synthesis of compound Lo. Thiosemicarbazide (0.0971 g, 1.066 mmol) and compound 2 (0.5 g, 1.066 mmol) were dissolved in anhydrous methanol (20 mL). Aceticacid (0.1 mL) was added as a catalyst and the mixture was refluxed for 2 h. Then the solution was concentrated to 10 mL and stood at room temperature for 3 h. The precipitate was filtered and washed 4 times with cold ethanol (20 mL). After drying under reduced pressure, dark red pure product was obtained (0.451 g). Yield: 73%. 1H NMR (400 MHz, DMSO-d6) δ (ppm) (Fig. S20, ESI†): 11.39 (s, 1H), 8.17 (s, 1H), 7.99 (s, 1H), 7.92 (s, 1H), 7.74–7.72 (d, J = 8 Hz, 2H), 7.64–7.62 (d, J = 8 Hz, 2H), 7.41–7.37 (t, J = 8 Hz, 2H), 7.32–7.23 (m, J = 16 Hz, 2H), 7.20–7.16 (d, J = 8 Hz, 2H), 7.13–7.11 (d, J = 8 Hz, 2H), 7.01–6.97 (t, J = 8 Hz, 2H), 6.83 (s, 1H), 2.61 (s, 2H), 2.54 (s, 2H), 1.02 (s, 6H). 13C NMR (100 MHz, CDCl3) δ (ppm) (Fig. S21, ESI†): 177.64, 170.20, 156.01, 148.06, 147.82, 145.13, 141.74, 137.41, 130.28, 130.23, 129.89, 129.32, 128.61, 127.79, 125.63, 124.77, 123.25, 122.54, 121.99, 114.03, 113.22, 75.29, 42.928, 38.22, 31.63, 27.41. FT-IR (KBr, cm−1) (Fig. S22, ESI†): 3413 (m), 3330 (m), 3276 (w), 3025 (w), 2951 (w), 2925 (w), 2869 (w), 2216 (s), 1594 (vs), 1559 (vs), 1522 (vs), 1496 (s), 1323 (m), 1278 (s), 1176 (m), 1154 (vs), 963 (m), 821 (w), 694 (w), 510 (w). MS (APCI) (Fig. S23, ESI†): m/z 542.227 [M, calcd 542.225]. EA: calcd: C 73.03, N 15.49, H 5.57; found: C 73.06, N 15.48, H 5.58.
Job plot
To determine the binding stoichiometry of the Lo–Cu2+ complex, Job plot is investigated by the fluorescence intensity of probe Lo at 637 nm were plotted as a function of their molar fraction under a constant total concentration (1 × 10−5 M), with a continuous variable molar fraction of Cu2+.
Detection limits
The detection limit was determined by using fluorescence titrations. The fluorescence spectrum of Lo was measured 10 times and a standard deviation of a blank solution was achieved. To obtain the slope, the ratio of the fluorescence emission at 637 nm was plotted vs. the concentration of Cu2+ ions. The detection limit was calculated based on the equation DL = K × SD/S, where K = 3, SD is the standard deviation of the blank solution, and S is the slope of the calibration curve.22
Effect of pH
Probe Lo (50 μL) was added in 4.95 mL acetonitrile–buffer solution (v/v = 9/1). The configuration of the buffer solution: pH 1–2, with HCl modulation directly; pH 3–4, with KHP/HCl modulation directly; pH 5–6, with KHP/NaOH modulation directly; pH 7–10, with HEPES/NaOH modulation directly; pH 11–13, with NaOH modulation directly. Here, the KHP is potassium hydrogen phthalate; 4-hydroxyethyl HEPES is piperazine ethyl sulfonic acid. All of the solution we had prepared act as solvent dilution.
Cell culture and imaging studies23
For HepG2 cells (liver hepatocellular carcinoma), the medium used was Dulbecco's Modified Eagle's Medium (DMEM) supplemented with 10% fetal calf serum (FCS, GIBCO), penicillin and streptomycin, L-glutamine and fungizone. For live cell confocal laser scanning microscopy experiment, HepG2 cells were seeded in 24 well glass bottom plate at density of 10
000, and incubated for 72–96 hours at 37 °C in 95% air 5% CO2 in order to allow the cells to reach ∼80% confluence, the medium changed every two days. Some of adherent cells were incubated with 10.0 μM probe Lo for 0.5 h at 37 °C under 5% CO2 and then washed with PBS three times before incubating with 100.0 μM Cu(NO3)2 for another 0.5 h, cells were rinsed with PBS three times again, then the fluorescence imaging of intracellular Cu2+ was observed under a LSM710 confocal laser scanning microscope (Zeiss) with an objective lens (×60). The raw HepG2 cells only incubated with 10.0 μM Lo for 0.5 hour at 37 °C under 5% CO2 was as a control.
Cytotoxicity assays in cells24
To ascertain the cytotoxic effect of the three compounds, the 3-(4,5-dimethylthiazol-2-yl)-2,5-diphenyltetrazolium bromide (MTT) assay was performed. HepG2 cells were trypsinized and plated to ∼80% confluence in 96 well plates (∼1 × 104 cells per well) 48 h before treatment. Prior to the compounds' treatment, the Dulbecco's Modified Eagle's Medium (DMEM) was removed and replaced with fresh DMEM, and aliquots of the compound stock solutions (1 mM DMSO) were added to obtain final concentrations of 5, 10, 20 and 40 μM. The treated cells were incubated for 24 h at 37 °C and under 5% CO2. Subsequently, the cells were treated with 5 mg mL−1 MTT (40 μL per well) and incubated for an additional 4 h (37 °C, 5% CO2). Then, DMEM was removed, the formazan crystals were dissolved in DMSO (150 μL per well), and the absorbance at 570 nm was recorded. The cell viability (%) was calculated according to following equation:
where OD570 (sample) represents the optical density of the wells treated with various concentration of the compounds and OD570 (control) represents that of the wells treated with DMEM + 10% fetal calf serum (FCS). Three independent trials were conducted, and the averages and standard deviations are reported. The reported percent cell survival values are relative to untreated control cells.
Computational studies
All geometries were optimized at B3LYP level of theory and with 6-31G+(d) basis set as implemented in the Gaussian 09 programs.25 The absorption spectra were calculated using time-dependent density functional theory (TD-DFT).26
Acknowledgements
This work was supported by the National Natural Science Foundation of China (21271003, 21271004, 51432001 and 51472002), Science and Technology Plan of Anhui Province (1604b0602016), the Ministry of Education of the People's Republic of China, Higher Education Revitalization Plan Talent Project of Anhui Province (2013).
Notes and references
-
(a) M. C. Lindeer and M. H. Azam, Am. J. Clin. Nutr., 1996, 63, 797–811 Search PubMed;
(b) G. Peers and N. M. Price, Nature, 2006, 441, 341–344 CrossRef CAS PubMed;
(c) K. Rurack, M. Kollmannsberger and J. Daub, J. Am. Chem. Soc., 2000, 122, 968–969 CrossRef CAS;
(d) S. Y. Moon, N. R. Cha and Y. H. Kim, J. Org. Chem., 2004, 69, 181–183 CrossRef CAS PubMed;
(e) A. Mathie, G. L. Sutton and C. E. Clarke, Pharmacol. Ther., 2006, 111, 567–583 CrossRef CAS PubMed.
-
(a) Y. F. Tan, N. L. Taylor and A. H. Millar, Plant Physiol., 2010, 152, 747–761 CrossRef CAS PubMed;
(b) V. Desai and S. G. Kaler, Am. J. Clin. Nutr., 2008, 88, 855–858 Search PubMed;
(c) C. N. Hancock, L. H. Stockwin and B. Han, Free Radical Biol. Med., 2011, 50, 110–121 CrossRef CAS PubMed.
-
(a) H. I. Un, S. Wu and C. B. Huang, Chem. Commun., 2015, 51, 3143–3146 RSC;
(b) Z. Yu, R. M. Schmaltz and T. C. Bozeman, J. Am. Chem. Soc., 2013, 135, 2883–2886 CrossRef CAS PubMed;
(c) Q. H. You, W. H. Chan and X. M. Zhu, Chem. Commun., 2014, 50, 6207–6210 RSC;
(d) Y. H. Lee, Y. B. Park and Y. J. Hwang, Chem. Commun., 2014, 50, 3197–3200 RSC;
(e) W. Li, X. H. Tian, B. Huang, H. J. Li, X. Y. Zhao, S. Gao, J. Zheng, X. Z. Zhang, H. P. Zhou, Y. P. Tian and J. Y. Wu, Biosens. Bioelectron., 2016, 77, 530–536 CrossRef CAS PubMed;
(f) Z. Li, E. W. Miller, A. Pralle, E. Y. Isacoff and C. J. Chang, J. Am. Chem. Soc., 2006, 128, 10–11 CrossRef PubMed;
(g) R. Krämer, Angew. Chem., Int. Ed., 1998, 37, 772–773 CrossRef.
-
(a) O. S. Bushuyev, T. A. Singleton and C. J. Barrett, Adv. Mater., 2013, 25, 1796–1800 CrossRef CAS PubMed;
(b) D. Kitagawa, H. Nishi and S. Kobatake, Angew. Chem., Int. Ed., 2013, 52, 9320–9322 CrossRef CAS PubMed;
(c) F. Terao, M. Morimoto and M. Irie, Angew. Chem., Int. Ed., 2012, 51, 925–928 CrossRef.
-
(a) J. Kärnbratt, M. Hammarson and S. Li, Angew. Chem., Int. Ed., 2010, 49, 1898–1901 CrossRef;
(b) R. C. Shallcross, P. Zacharias and A. Köhnen, Adv. Mater., 2013, 25, 469–476 CrossRef CAS PubMed;
(c) S. Kobatake, H. Imagawa and H. Nakatania, New J. Chem., 2009, 33, 1362–1367 RSC.
-
(a) K. Kreger, P. Wolfer and H. Audorff, J. Am. Chem. Soc., 2010, 132, 509–516 CrossRef CAS PubMed;
(b) A. Ryabchun, A. Bobrovsky and A. Sobolewska, J. Mater. Chem., 2012, 22, 6245–6250 RSC.
-
(a) M. Han, R. Michel and B. He, Angew. Chem., Int. Ed., 2013, 52, 1319–1323 CrossRef CAS PubMed;
(b) R. Tong, H. D. Hemmati and R. Langer, J. Am. Chem. Soc., 2012, 134, 8848–8855 CrossRef CAS PubMed.
-
(a) S. C. Sebai, D. Milioni and A. Walrant, Angew.
Chem., Int. Ed., 2012, 51, 2132–2135 CrossRef CAS PubMed;
(b) M. Lohse, K. Nowosinski and N. L. Traulsen, Chem. Commun., 2015, 51, 9777–9780 RSC;
(c) D. Kim, S. A. Lee and H. Kim, Chem. Commun., 2015, 51, 11080–11083 RSC;
(d) R. S. Stoll, M. V. Peters and A. Kuhn, J. Am. Chem. Soc., 2009, 131, 357–367 CrossRef CAS PubMed;
(e) W. Fuß, C. Kosmidis and W. E. Schmid, Angew. Chem., Int. Ed., 2004, 43, 4178–4182 CrossRef PubMed.
-
(a) J. C. Chan, W. H. Lam and H. L. Wong, J. Am. Chem. Soc., 2011, 133, 12690–12705 CrossRef CAS PubMed;
(b) K. Liu, Y. Wen and T. Shi, Chem. Commun., 2014, 50, 9141–9144 RSC;
(c) T. Sumi, T. Kaburagi and M. Morimoto, Org. Lett., 2015, 17, 4802–4805 CrossRef CAS PubMed;
(d) L. L. Hou, X. Y. Zhang and T. C. Pijper, J. Am. Chem. Soc., 2014, 136, 910–913 CrossRef CAS PubMed.
-
(a) G. Tomasello, M. J. Bearpark and M. A. Robb, Angew. Chem., Int. Ed., 2010, 49, 2913–2917 CrossRef CAS PubMed;
(b) V. I. Minkin, Chem. Rev., 2004, 104, 2751–2776 CrossRef CAS PubMed;
(c) R. Klajn, Chem. Soc. Rev., 2013, 43, 148–184 RSC;
(d) Y. S. Nam, I. Yoo and O. Yarimaga, Chem. Commun., 2014, 50, 4251–4254 RSC.
- M. D. Yang, H. Z. Wang, J. Huang, M. Fang, B. Mie, H. P. Zhou, Y. P. Tian and J. Y. Wu, Sens. Actuators, B, 2014, 204, 710–715 CrossRef CAS.
- D. Maity and T. Govindaraju, Chem.–Eur. J., 2011, 17, 1410–1414 CrossRef CAS PubMed.
- S. Malkondu and S. Erdemir, Tetrahedron, 2014, 70, 5494–5498 CrossRef CAS.
- M. Q. Wang, K. Li and J. T. Hou, J. Org. Chem., 2012, 77, 8350–8354 CrossRef CAS PubMed.
- M. Shortreed, R. Kopelman and M. Kuhn, Anal. Chem., 1996, 68, 1414–1418 CrossRef CAS PubMed.
- W. Lin, L. Yuan and Z. Cao, Eur. J. Chem., 2009, 15, 5096–5103 CrossRef CAS PubMed.
-
(a) H. N. Kim, Z. Guo and W. Zhu, Chem. Soc. Rev., 2011, 40, 79–93 RSC;
(b) N. Boens, V. Leen and W. Dehaen, Chem. Soc. Rev., 2012, 41, 1130–1172 RSC.
-
(a) H. N. Kim, Z. Guo, W. Zhu, J. Yoon and H. Tian, Chem. Soc. Rev., 2011, 40, 79–93 RSC;
(b) N. Boens, V. Leen and W. Dehaen, Chem. Soc. Rev., 2012, 41, 1130–1172 RSC.
-
(a) R. Pandey, R. K. Gupta and P. Z. Li, Org. Lett., 2012, 14, 593–595 CrossRef PubMed;
(b) N. J. A. Coughlan, B. D. Adamson and L. Gamon, Phys. Chem. Chem. Phys., 2015, 17, 22623–22631 RSC;
(c) X. Li, L. W. Chung and K. J. Morokuma, J. Chem. Theory Comput., 2011, 7, 2694–2698 CrossRef CAS PubMed.
- T. Sarkar, S. Banerjee and A. Hussain, RSC Adv., 2015, 5, 16641 RSC.
- W. Li, Y. Zhang, X. P. Gan, M. D. Yang, B. Mie, M. Fang, Q. Y. Zhang, J. H. Yu, J. Y. Wu, Y. P. Tian and H. P. Zhou, Sens. Actuators, B, 2015, 206, 640–646 CrossRef CAS.
- W. Lin, L. Yuan, Z. Cao, Y. Feng and L. Long, Eur. J. Chem., 2009, 15, 5096–5103 CrossRef CAS PubMed.
- A. Rai, N. Kumari, R. Nair, K. Singh and L. Mishra, RSC Adv., 2015, 5, 14382 RSC.
- T. Mosmann, J. Immunol. Methods, 1983, 65, 55–63 CrossRef CAS PubMed.
-
(a) M. J. Frisch, G. W. Trucks, H. B. Schlegel, G. E. Scuseria, M. A. Robb, J. R. Cheeseman, G. Scalmani, V. Barone, B. Mennucci and G. A. Petersson, Gaussian 09, Wallingford, CT, 2009 Search PubMed;
(b) A. D. Becke, J. Chem. Phys., 1993, 98, 5648–5652 CrossRef CAS.
-
(a) R. Bauernschmitt and R. Ahlrichs, Chem. Phys. Lett., 1996, 256, 454–464 CrossRef CAS;
(b) R. E. Stratmann, G. E. Scuseria and M. J. Frisch, J. Chem. Phys., 1998, 109, 8218–8224 CrossRef CAS.
Footnote |
† Electronic supplementary information (ESI) available: Synthesis, characterization spectra and theoretical calculations. See DOI: 10.1039/c6ra05699g |
|
This journal is © The Royal Society of Chemistry 2016 |