DOI:
10.1039/C6RA04421B
(Review Article)
RSC Adv., 2016,
6, 45462-45474
Relaxivity and toxicological properties of manganese oxide nanoparticles for MRI applications
Received
18th February 2016
, Accepted 1st May 2016
First published on 10th May 2016
Abstract
Manganese oxide nanoparticles (MONs) have received growing attention as alternative T1 MRI contrast agents due to the association of commercial gadolinium-based contrast agents with nephrogenic systemic fibrosis. Since the seminal publication first describing the use of MONs as positive T1 contrast agents, there is an ongoing impetus to develop MONs of higher T1 signal intensity for better diagnostic efficacy. Indeed, various MON-based nanoprobe designs have been proposed, such as the employment of a mesoporous nanomaterial with MONs evenly dispersed within, or the traditional coating of a biocompatible layer onto the surface of MONs to form a core–shell configuration. Recent advances in this field also propose stimuli-responsive MONs that capitalize on an acidic dissolution or in situ reduction to release Mn2+ ions for a multi-fold increase in MRI contrast. However, the potential nanotoxicity of MONs remains a key obstacle to the clinical translation of MON-based T1 contrast agents. Due to the wide variety of functionalities and physicochemical properties of MONs, there is also a lack of consensus on the toxicological properties of MONs. In addition, the r1 relaxivity of MRI contrast agents typically decreases at higher field strength. Hence, it highlights the need to develop MON-based contrast agents with higher relaxivities. In this regard, this article aims to present a thorough review of MONs for MRI applications, with particular emphasis on their relaxivity and toxicological properties. In order to systematically review the current state-of-the-art for the development of MONs for MRI applications, the MON-based T1 contrast agents are categorized based on the structure of the nanomaterial system. Key parameters that influence the nanotoxicity of MONs are also examined while the absorption, distribution, metabolism and excretion of MON in vivo are evaluated to discern how long the nanoparticles will be present within the body, as well as to predict the organs or tissues in which they distribute.
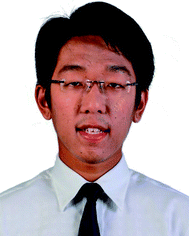 Benedict You Wei Hsu | Benedict You Wei Hsu pursued his PhD degree in the NUS Graduate School for Integrative Sciences & Engineering (NGS), National University of Singapore. He is currently working as a research scientist in the Institute of Materials Research and Engineering (IMRE), Agency for Science, Technology and Research (A*STAR). His research interests include the synthesis of inorganic metal oxide nanoparticles and the development of multifunctional nanomaterials for biomedical applications. |
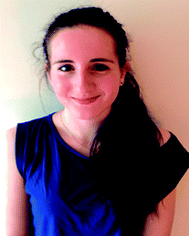 Georgia Kirby | Georgia Kirby read Medicine at King's College, University of Cambridge. She graduated with a BA in Medical Science from the University of Cambridge and is currently studying clinical medicine at University College London (UCL). Her research interests are in elucidating the physiological effects of nanoparticles in human biological systems. |
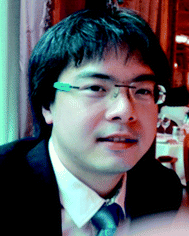 Aaron Tan | Aaron Tan is an MBBS/PhD medical student at University College London (UCL). He obtained both his BSc in Surgical Sciences and PhD in Surgery & Bioengineering from UCL. He is currently studying clinical medicine at UCL and is a visiting scholar at the Biomaterials & Advanced Drug Delivery Laboratory (BioADD) at Stanford University. His research interests are broadly in the areas of nanoscience and nanotechnology for surgical applications. |
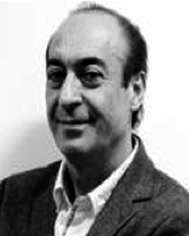 Alexander M. Seifalian | Alexander M. Seifalian is Professor of Nanotechnology & Regenerative Medicine at University College London (UCL), and co-director of NanoRegMed Ltd. He obtained his BSc from King's College London, and his MSc and PhD from UCL. He has received top prizes and accolades for his research in nanotechnology and artificial organs, and has published over 441 peer-reviewed papers, 41 book chapters, and holds 9 patents. |
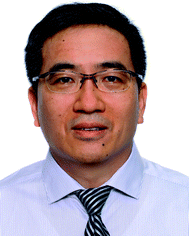 Xu Li | Xu Li is a Senior Scientist at Institute of Materials Research and Engineering, Agency for Science, Technology and Research. He finished his PhD study in chemistry in the Department of Chemistry, National University of Singapore (NUS) in 2001. He is an adjunct Associate Professor of the Department of Chemistry, NUS from 2012. He has published more than 80 research papers and filed 12 international patents. 5 of the patents were adopted by industry companies through technology licensing, including the one on fluorescent nanoparticles for bioimaging. |
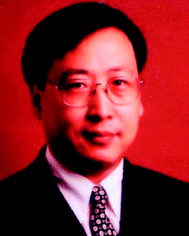 John Wang | John Wang is Professor and Head of Department of Materials Science and Engineering, and Senior Faculty Member, NUS Graduate School for Integrative Sciences & Engineering (NGS), National University of Singapore. He has more than 30 years of experience in teaching and research of functional materials and materials chemistry. His current research focuses include: materials chemistry, biomaterials and nanohybrids for bioimaging and healthcare, and nanostructured materials for sustainable energy. Professor John Wang has published >300 papers in prestigious, top international refereed journals. He has been invited, on a regular basis, to give keynote/invited lectures at major international conferences/symposia/workshops. |
1. Introduction
Apart from the non-invasive acquisition of anatomical images of high spatial resolution, magnetic resonance imaging (MRI) does not rely on the usage of radioisotopes or ionizing radiation. Hence, it has been the diagnostic tool of choice to image the central nervous system and neurological cancers.1 However, MRI contrast arising from the variations in relaxation characteristics of different tissues of interest may not be substantial enough to generate an obvious signal contrast in the MR images. Thus, in order to improve lesion delineation and image clarification, contrast agents are often administered intravenously in patients. In the current clinical setting, gadolinium-based contrast agents (GBCAs) are commonly used. Yet, due to its recent association with nephrogenic systemic fibrosis,2,3 there is an emerging research impetus to develop alternative T1 MRI contrast agents that can reduce the risk of toxicity while maintaining a comparable high imaging performance to the commercial GBCAs. As such, manganese oxide nanoparticles (MONs) have received great attention in recent years, because they are potentially less toxic than its lanthanide counterparts due to the physiological function of manganese as a regulatory cofactor for many enzymes and receptors.2 Besides, in contrast to the chelated complexes of GBCAs, the nanoparticulate characteristics of MONs can ensure a prolonged blood circulation time,4 thereby providing a clinically feasible scan time to achieve higher spatial MRI resolution.
Indeed, the in vivo applications of MONs as positive T1 MRI contrast agents have been demonstrated in preliminary animal studies. For example, formulations of PEG–phospholipid coated MONs were successfully employed for the anatomical imaging of various brain structures in mouse.5 Nonetheless, as MONs generally remained inferior in T1 contrast enhancement as compared to GBCAs, there is an ongoing emphasis to develop MONs of higher T1 relaxivity for better diagnostic efficacy. As a result, a diverse array of engineered MON-based T1 probes have emerged. Recent advances in this field also propose nanoprobe designs which capitalize on the acidic dissolution or the in situ reduction of MONs to release Mn2+ ions for a multi-fold increase in T1 MRI contrast.6,7 Yet, based on the current literature, there is a paucity of reviews that particularly devote to the progress made in the last decade in the design of MONs as T1 MRI contrast agents, as well as to assess the potential toxic effects of MONs on the human health.3 Therefore, in this review, we aim to accomplish the following: (i) to systematically review the current state-of-the-art for the development of MONs as MRI contrast agents; (ii) to propose a systematic classification of key parameters that influences the paramagnetic relaxation enhancement and cytotoxicity assessment of MONs; and (iii) to highlight representative case studies to elucidate the in vivo nanotoxicological profile of MONs. This will be followed by concluding remarks and a summary of future outlook.
2. Development of MON-based nanoplatforms as MRI contrast agents
For oxides of manganese to function as strong T1 MRI contrast agents, they must contain the divalent manganese (Mn2+) ions, which are characterized by the presence of 5 unpaired electrons in their 3d shell. This creates a large magnetic moment and is responsible for inducing magnetic relaxation in the nearby nuclei.1 Comparatively, the higher valence states of Mn tend to exhibit less effective T1 relaxation due to fewer unpaired electrons and a smaller electron spin relaxation time.8 As a result, the development of manganese-based T1-weighted MRI is primarily focused on the MnO or Mn3O4 phase, though there are some exceptions which would be highlighted later. In addition, a nanoparticulate system which contains the magnetically active MnO component is also preferred due to the high surface concentration of Mn2+ ions. Hence, the nanomaterial based on MON is able to carry a large paramagnetic payload and exhibits a strong T1 contrast enhancement even at a reduced dose. In this regard, there is a variety of MON-based nanoplatforms that have been developed for MRI as T1 contrast agents.
2.1 Uniformly dispersed MONs in mesoporous framework
Mesoporous silica nanoparticles (MSNs) are often used as the framework with MONs evenly dispersed within. This is because they allow the dispersed MONs to be easily accessible to water molecules, which facilitates their performance as contrast agents. For example, Chen et al. synthesized manganese oxide–mesoporous silica nanoparticles (Mn–MSNs) using a multi-step synthetic strategy.7,8 In their study, the MONs were first formed in situ inside the mesopores by the chemical reduction of KMnO4 aqueous solution, followed by heat treatment in a reducing atmosphere of H2 or Ar (Fig. 1). In a separate study, Wang et al. used a hydrothermal method to produce MONs-capped MSNs,9 wherein the pre-synthesized water dispersible MONs were conjugated to the mesoporous framework via EDC chemistry.
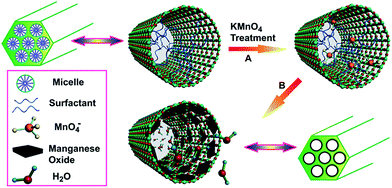 |
| Fig. 1 Schematic illustration for the preparation of Mn–MSNs. Reprinted from ref. 8. Copyright (2012), with permission from Elsevier. | |
Generally, these MONs-dispersed MSNs could be regarded as a new generation of stimuli-responsive MRI contrast agents because the integrated MONs would readily disintegrate in response to specific triggers to form free Mn2+ ions, which have a significantly higher r1 relativity as compared to its metal oxide counterparts.10 For example, Chen et al. demonstrated that the Mn–MSNs could be activated under mild acidic conditions to attain a high r1 relaxivity of 8.81 mM−1 s−1.7 On the other hand, Wang et al. showed that exposure to a highly reducing environment could similarly cause the dissolution of MONs to release free Mn2+ ions for a twofold increase in T1 signal.9 Such stimuli-responsive behaviour can be attributed to the absence of a protective coating layer for MONs, thereby rendering the exposed nanoparticles to be particularly susceptible to degradation in mild acidic and reduction environment.7–9 Besides MSNs, graphene oxide (GO) is another suitable candidate to construct the framework. By using a similar double redox strategy as mentioned above,7,8 Chen et al. successfully dispersed MONs onto exfoliated GO nanosheets.11 It was reported that the integrated MONs could also exhibit both pH-responsive and reduction-triggered T1-weighted MRI, thus are highly attractive for stimuli-responsive theranostics.
Nonetheless, there is a possible weakness that can arise from the poor physico-chemical stability of MONs in the mesoporous framework. Certainly, the integrated MONs would release Mn2+ ions upon acidic dissolution or in situ reduction and this property can be utilized for stimuli-responsive dynamic T1-weighted MRI. However, the chronic exposure to high levels of the Mn2+ ions can lead to an extrapyramidal disorder similar to Parkinson's disease,12 thereby fostering concern on their clinical use. This was also indicated in studies of BxPC-3 cells incubated with MONs-dispersed MSNs wherein it was also observed that the nanoparticles exhibited no cytotoxicity until upon dissolution in a reducing environment to release free Mn2+ ions.9 Nonetheless, to prevent excessive exposure to Mn2+ ions, a possible strategy is to confer gate-keeping properties to limit the rate of Mn2+ ion release. For example, light-induced switchable rotaxanes can be covalently conjugated to the surface to prepare functional MSNs that can be readily modulated by photothermal action.13
Another important factor of toxicity lies in the surface properties of the mesoporous framework. In this regard, unmodified MSNs, which tend to be negatively charged under physiological conditions, have been found to be more cytotoxic than their cationically modified counterparts.14 Pasqua et al. showed that the thiol- or amino-functionalization of MSNs is able to reduce the toxicity of MSNs under in vitro conditions.15 Similarly, Yildirim et al. also indicated that the hemolytic activity of MSNs could be significantly reduced by functionalization with organosilane monomers.16 Hence, this improves the blood compatibility of MSNs, which is an important aspect to be considered for the intravenous injection of nanoparticles as MRI contrast agents. In most of the studies reported so far, synthesis of MSNs also employs structure-directing agents to generate the mesopores. Herein, the biocompatibility of the structure-directing agents is important to minimize particle-induced toxicity. On this topic, the interested reader is recommended to consult some of the excellent reviews covering the different approaches to MSN syntheses.14
2.2 Core–shell structured MONs
Metabolomics analyses have indicated that in vivo exposure to uncoated MONs could lead to disturbances to a number of metabolic processes and induce hepatic necrosis to a certain extent.17 Hence, as indicated in Fig. 2, the MONs developed for MRI are traditionally coated by a biocompatible layer to form a core–shell configuration. For this class of nanosystem, newly-prepared MONs are typically used as a hard template for the subsequent coating of the shell material. This approach is advantageous because the synthesis of metal oxide nanoparticles with precisely controlled shape and size distribution is well-established.18 Thus, it ensures that the resultant MONs are monodispersed and offers an avenue to tailor the magnetic properties of MONs for higher r1 relaxivity. However, due to the inherent hydrophobicity of the resultant MONs, it also implies that the coating materials must to be able to render the MONs water-dispersible and colloidally stable in the physiological environment. In this regard, the covalent attachment of PEG chains to the coating layer is a popular strategy to improve the aqueous solubility of MONs. In particular, Gallo et al. reported that longer PEG molecules (∼5000 Da) were preferred for nanoparticle functionalization because they prolonged the blood circulation time of MONs and afforded a greater accumulation in tumours.19
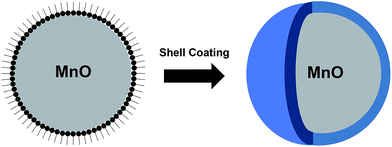 |
| Fig. 2 Schematic representation for the biocompatible shell coating of MONs to form a core–shell configuration. | |
Ideally, the coating material should also be sufficiently thin so that the overall diameter remains less than 100 nm; such dimension would favour the reduced opsonisation of MONs so that the signal duration is sufficiently long for in vivo MR contrast enhancement. More importantly, the coating material should also allow the encapsulated manganese paramagnetic core to interact readily with the surrounding water molecules for higher r1 relaxivity. For example, despite the excellent biocompatibility of silica, it has been argued that the r1 relaxivity of silica-coated MONs is inevitably affected by the dense silica coating layer, which reduces the accessibility of water molecules to the MnO core.
From a toxicological standpoint, the coating material should be stable, both during storage and under in vivo conditions. This is because compromise of the coating layer for core–shell structured MONs can reveal the metal oxide core to release metal ions (Mn2+) upon dissolution, which is toxic. This would preclude the use of numerous amphiphilic polymers as the coating material due to the inherent instability of the polymeric micelles at concentrations below the critical micelle concentration of the polymer. Nonetheless, such limitations can be overcome by crosslinking the micelle shell so that it does not readily disintegrate into its different constituents in the physiological environment. Finally, cytotoxicity of the coating material itself is a major determining factor in the overall toxicity of MONs. It has to be considered alongside with improving the r1 relaxivity of MONs so as to develop safe nanoprobes of excellent contrast ability for practical clinical MRI applications. To this extent, we propose to categorize the core–shell structured MONs based on coating materials, which will be elaborated in a later section.
2.3 Rattle-type structured MONs
In contrast to the core–shell structured MONs, rattle-type structured MONs refers to the formation of hollow shell with a solid MON core and interstitial hollow/soft-material filled space in between. Due to the excellent loading capacity and large interior surface area of rattle-type nanostructures, they have attracted much attention in many biomedical applications such as drug storage and controlled release. To prepare rattle-type structures, most of the synthetic strategies involve the formation of core–shell nanostructures, followed by a partial removal of the shell material from inside.20 This removal process typically entails calcination or etching in appropriate solvents. However, given that MONs are readily oxidised at high temperatures and susceptible to acidic/redox conditions, it remains a challenge to employ this strategy for the fabrication of rattle-type structured MONs. Nonetheless, Hsu et al. have developed an interfacial templating technique to synthesize rattle-type structured MONs with a nanohybrid shell layer of silica and F127,21 as illustrated in Fig. 3. In this non-conventional approach, hydrolysis and condensation of the silica precursors is confined to the interfacial region between the PPO core and the PEO corona of F127 micelles. Hence, it prevents the MON core from being in direct contact with the peripheral silica nanoshell and facilitates the passage of water molecules to the encapsulated MON since the hydroxyl groups on the MON surface are not involved in the sol–gel process.21 Interestingly, the synthesis protocol was also facilely extended to yield hollow MONs when immersed in an acetate buffer solution, thus yielding MONs of higher surface-to-volume ratio.
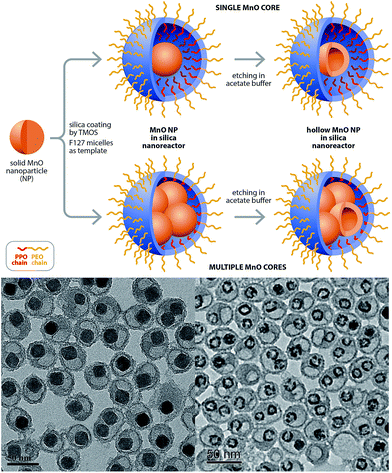 |
| Fig. 3 Schematic representation for the preparation of rattle-typed structured MONs (solid/hollow) and their corresponding TEM images. Reproduced from ref. 21 with permission from the Royal Society of Chemistry. Reprinted with permission from ref. 38. Copyright 2015 Wiley-VCH. | |
In general, the rattle-type structured MONs were observed to exhibit a higher r1 relaxivity as compared to that of other core–shell structured MONs of similar shell material. For instance, the r1 value of silica-F127 nanohybrid encapsulated MONs was reported to be 1.17 mM−1 s−1 while the r1 values of core–shell structured MONs were 0.08 and 0.65 mM−1 s−1, when coated with dense silica and mesoporous silica respectively.22 Besides the excellent imaging ability of rattle-type structured MONs, Hsu et al. also reported that for the formation of silica-F127 nanohybrid encapsulated MONs,21 the silica plays an important role in reinforcing the F127 micellar structure. Thus, the colloidal stability of MONs is improved and there is minimal leaching of the Mn2+ ions. Moreover, a high cell viability of 83% was achieved when MDA-MB-231 cells were incubated at a high nanoparticle concentration of 224.9 μM Mn for 24 hours. This low toxicity profile is attributed to the proven biocompatibility of the two constituent materials of silica and F127 that form the shell layer.
2.4 Redoxable MnO2 nanosheets
Recently, MnO2 nanosheets have been proposed for use as T1 MRI contrast agents. However, unlike the previous class of MON-based nanoplatforms, MnO2 nanosheets per se tend to exhibit very low r1 relaxivity. This is due to fewer unpaired electrons in the d3 configuration of Mn4+ ions. As a result, the electron spin magnetic moment of Mn4+ ions is smaller and unable to accelerate the longitudinal relaxation of water protons effectively.23 Instead, the MnO2 nanosheets are uniquely designed to be intracellular glutathione (GSH)-activated MRI contrast agents.24,25 Herein, GSH is a cytosolic biomolecule which is often present in elevated levels in cancerous tissues. As a reducing agent, GSH could also induce the dissolution of MnO2 nanosheets and release Mn2+ ions for MRI contrast enhancement. It is noteworthy that these MnO2 nanosheets could also be coupled to lanthanide-doped upconversion nanoparticles24 or cyanine dyes25 to incorporate fluorescence-activatable properties for cancer diagnostics applications. As shown in Fig. 4, for such hybrid probe design, the MnO2 nanosheets would serve to quench the fluorophores effectively. However, upon cellular uptake by the targeted cancer cells, the MnO2 nanosheets would be reduced by the intracellular GSH, thus leading to fluorescence restoration of the nanoprobe.
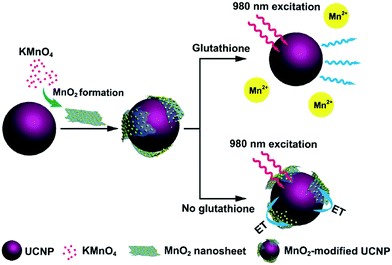 |
| Fig. 4 Schematic illustration of MnO2-nanosheet-modified upconversion nanoparticles for intracellular glutathione detection. Reprinted with permission from ref. 24. Copyright 2011 American Chemical Society. | |
Nonetheless, there are concerns about the biocompatibility of MnO2 nanosheets despite their novel stimuli-responsive MRI behaviour. Studies have suggested that the MnO2 nanomaterial could induce significant accumulation of reactive oxygen species and result in the formation of oxidative stress in cells.26 Hence, this would lead to DNA oxidative damage-induced necrosis and cell apoptosis. Yet, in the designs of MnO2 nanosheets for T1-weighted MRI,24,25 the MONs are typically not assigned with biocompatible secondary coatings to mitigate their risk of nanotoxicity, which is exacerbated by a lack of toxicology-based studies for this newly-developed nanosystem. Therefore, special attention should be given to determine the absorption, distribution, metabolism and excretion (ADME) characteristics of the released free Mn2+ ions from the nanosheets.
2.5 MON-based heterodimer nanocomposites
The feasibility of developing MON-based hetero-nanoparticles has been demonstrated by various research groups. Touted as the next generation of Janus-type nanoparticles, it is envisioned that such nanocomposites would be highly versatile for applications in biomedical imaging due to their multi-functional capabilities. For instance, Im et al. have reported the synthesis of a T1–T2 dual modal MRI contrast agent based on Fe3O4/MnO hybrid nanocrystals.27 On the other hand, Schladt et al. successfully constructed Au@MnO nanoflowers for concurrent magnetic and optical detection (Fig. 5).28
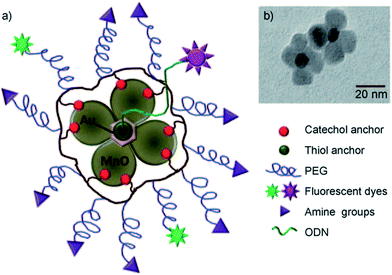 |
| Fig. 5 (A) Schematic representation and (B) the corresponding TEM image of a polymer-coated Au@MnO nanoflower. Reprinted with permission from ref. 28. Copyright 2010 Wiley-VCH. | |
Hetero-nanoparticles are synthesized by heterogeneous nucleation of MONs onto the surface of the other inorganic counterpart (e.g. Fe3O4 or Au nanocrystals). As a result, the two inorganic domains are joined together through a small interfacial area to form an asymmetric structure with different regions of surface-active properties. Interestingly, it has been reported that a synergistic enhancement in the nanoscale properties could also be achieved in anisotropic gold and metal oxide nanoparticles (e.g. Au@MnO heterodimer). Unlike the isotropic analogues with a uniform wettability, these Janus-type nanoparticles would exhibit an amphiphilic character, thereby favouring their self-assembly at the oil-water interface due to the drastic decrease in interfacial tension.29 Moreover, selective surface functionalization could also be achieved. For example, Schick et al. successfully encapsulated the metal oxide domain with a silica coating while leaving the gold domain untouched.30
In general, cell viability assays have suggested the non-cytotoxic nature of MON-based heterodimers.28,30 However, comparison between these studies can be somewhat difficult due to the lack of uniformity in the methods used to assess toxicity and the vastly different physicochemical properties of individual compounds. Besides, the combination of multiple inorganic nanoparticles in the hetero-structure would also increase the complexity of cytotoxicity assessment since the dissolution of either inorganic core to their constituent metal ions could increase the potential toxicity risks. As has been established in core-structured MONs, the toxicity risks of these nanocomposites are also strongly influenced by the coating material used. This is because the synthesis of hetero structured MONs are typically carried out through non-hydrolytic routes, thus a suitable ligand or shell coating is required to improve their aqueous dispersibility.
3. Paramagnetic relaxation enhancement
It is noteworthy that MRI is increasingly shifting to higher fields (1.5 T and above) for greater signal-to-noise ratio and higher spatial resolution and/or reduced acquisition time. Yet, the r1 relaxivity of MRI contrast agents typically decreases at higher field strength. For example, Kim and co-workers reported that the r1 value of mesoporous silica-coated hollow MONs decreases from 1.72 mM−1 s−1 at 1.5 T to 0.99 mM−1 s−1 at 11.7 T.22 This can be attributed to the increased magnetization of paramagnetic MONs in higher magnetic fields, which favors the domination of T2 effects and a reduced effectiveness of MONs to shorten the T1 relaxation time of water.31 Therefore, it highlights the need to develop MON-based contrast agents of higher relaxivities. Moreover, in lieu of the direct dose-dependent relationship on the contrast enhancement effect, a higher r1 relaxivity is also favourable to its toxicity profile since a lower dose of the contrast agent can be administered.
Although the fundamental theories underlying the paramagnetic relaxation enhancement of T1 contrast agents fall beyond the scope of the current review, there are several excellent sources available on this subject.32–34 For a simplified treatment of the phenomenon, the T1 contrast agent can be envisioned to have different solvation spheres.31 Since the major relaxation mechanism arises from the dipole–dipole coupling between water protons and the paramagnetic ion, the inner-sphere water molecules can form stronger dipole–dipole interactions and are more susceptible to spin-lattice relaxation. Thus, in order to increase the r1 relaxivity of MONs, current strategies are largely targeted towards increasing the availability of water molecules in closer proximity to the magnetic core. In the case of core–shell structured MONs, this can be achieved by increasing the surface-to-volume ratio of MONs, as well as structural modifications of the coating layer to enhance its water permeability.
In addition, spin-lattice relaxation is also more efficient when the tumbling rate of T1 contrast agents (as characterized by the correlation time) corresponds closely to the proton Larmor precession frequency.34 Yet for nanoprobe designs (e.g. redoxable MnO2 nanosheets) which rely on the release of Mn2+ ions for T1 MRI contrast enhancement, it is likely that the small aqueous contrast agents have a high tumbling rate.31 As such, the correlation time should be modulated so that it corresponds more closely to the proton Larmor precession frequency, thereby attaining a higher r1 relaxivity.
3.1 Surface-to-volume ratio of MONs
Given that only the surface Mn2+ ions of the nanoparticle are mainly responsible for longitudinal water proton relaxation, a larger surface-to-volume ratio of MONs is expected to favour a more efficient rate of water exchange. For example, amongst the different nanostructured morphologies of MONs, nanoplates were observed to exhibit the highest r1 relaxivity due to their largest surface area.35 Na et al. also demonstrated that the T1-weighted MR images of MONs were considerably brighter as the nanoparticle size decreased.5 On the other hand, the formation of hollow MONs is another popular approach to improve the particle surface-to-volume ratio. This is typically achieved by employing a suitable etchant (e.g. phthalate buffer,36 mild hydrochloric acid22 or hydroxylamine solution37) to selectively remove the MnO core from a sacrificial template of MnO–Mn3O4 core–shell nanoparticles. Alternatively, as shown in Fig. 6, Hsu et al. have developed a hybrid silica nanoreactor to form well-defined hollow MONs of superior r1 relativity of up to 2.58 mM−1 s−1 even in a high magnetic field of 7 T.38 Herein, the hollow formation was attributed to the surface stabilization of MONs by acetate ions, followed by subsequent acidic etching of the MnO core interior. Moreover, the versatility of the silica nanoreactors to yield hollow MONs with various encapsulation morphologies was also demonstrated.
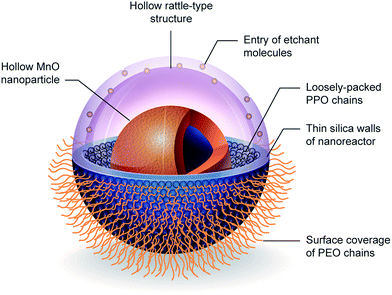 |
| Fig. 6 3D-illustration of silica nanoreactor framework to fabricate hollow MONs. Reprinted with permission from ref. 38. Copyright 2015 Wiley-VCH. | |
3.2 Water permeability of the coating layer
For the traditional core–shell structured MONs, the interaction of MONs with water molecules is strongly dependent on the porosity of the coating material. For instance, Peng et al. verified that a higher silica shell porosity would yield a larger r1 relaxivity due to the improved accessibility of water molecules to the encapsulated MONs.39 To generate mesopores in the silica shell, an organic structure-directing template such as cetyltrimethylammonium bromide (CTAB) is typically used.22,39 However, it should be noted that CTAB is toxic and any incomplete removal of this surfactant can impede their use for biomedical applications. In this regard, Hsu et al. have proposed the design of a silica nanoshell perforated with PEO chains to act as hydrophilic nanochannels for the rapid penetration of water molecules to the MnO core.21 Due to the highly permeable nature of the porosified silica nanoshell, the as-encapsulated MONs were shown to exhibit excellent T1 contrast enhancement even upon cellular uptake by MDA-MB-231 cells. Hydrophilicity of the polymer ligands is an important prerequisite too, owning to a more efficient water exchange exerted by the MONs. Xing et al. synthesized highly water-soluble polyaspartic acid (PASP)-coated MONs which exhibited an r1 relaxivity of 1.29 mM−1 s−1.40 In contrast, using the comparatively more hydrophobic poly(lactic-co-glycolic acid) (PLGA) as a coating material resulted in a lower r1 relaxivity of 0.21 mM−1 s−1 of the intact MONs.10
3.3 Effect of rotational dynamics
As has been illustrated by Caravan and co-workers, the paramagnetic relaxation could be optimized by a protein binding strategy to reduce the rotational correlation time of T1 contrast agents.31 For instance, the r1 value of MnL1 complex was shown to increase from 4.46 mM−1 s−1 (unbound form) to 5.78 mM−1 s−1 (protein-bound form) at 4.7 T. Similar studies have also suggested that dendrimer conjugation was another viable approach to favour a slower rotational correlation time for higher r1 relaxivity.41 Meanwhile, Hsu et al. proposed that for the encapsulation of a cluster of MONs in silica nanocarrier, the rotational diffusion of the paramagnetic payload is likely to be more restricted by the closer proximity and stronger polar interactions with the hydroxyl groups of the silica nanoshell.38 As a result, it would be able to induce the longitudinal water proton relaxation efficiently. Indeed, the effect of rotational dynamics is a useful parameter to optimize the r1 relaxivity, especially at low fields. Nonetheless, it is not without accompanying drawbacks. Herein, there are concerns that the binding of proteins (e.g. human serum albumin) could reduce the colloidal stability, thereby increasing their tendency to precipitate upon prolonged storage.42
4. Toxicological characteristics
Due to the wide variety of functionalities and physicochemical properties of MONs, there is a lack of consensus on the toxicological properties of MONs. It is in this context that an understanding of the toxicological characteristics of MONs is essential prior to their clinical translation. The toxicity of MONs can be induced by several mechanisms including the production of reactive oxygen species and an excessive accumulation of Mn2+ ions due to nanoparticle degradation. Table 1 is a summary of the in vitro studies performed to determine the potential nanotoxicity of MONs or lack thereof. Indeed, these in vitro assays can be used to screen hazardous nanomaterials and provide useful mechanistic insights about the nano-bio interactions. For example, MONs-dispersed MSNs were shown to exhibit a half-maximal inhibitory concentration (IC50) of 400 μg ml−1 with breast cancer MCF-7 cells after 36 hours incubation.8 Meanwhile, it was revealed that the high intracellular GSH concentration of cancer cells was sufficient to trigger the steady dissolution of Mn3O4 nanocrystals via a thiol-disulfide transformation to produce Mn2+ ions.9 Nonetheless, given the variability in cell lines and a wide range of nanoparticle concentration used to evaluate the nanotoxicity of MONs, comparability of the in vitro studies may be open to doubt due to the lack of test procedure standardization. Often, the problem is complicated by insufficient characterization of the investigated nanomaterial.43 Therefore, in the following section, we would discuss the key parameters that influence the cytotoxicity of MONs, which are essentially dependent on the nanocarrier material and the individual physicochemical properties of MONs.
Table 1 Summary of toxicological studies performed to determine the potential toxicity of MONs
Type of MONs |
Cell assay |
Conditions |
Dosage |
Relaxivity |
Cytotoxicity |
Ref. |
PEG–phospholipid |
PC-3/U-87 MG/MCF7/Huh7/MRC5/NCI H460/HEK 293/HL-60 cell lines (SRB or WST-8 assays) |
1 × 103 to 25 × 103 cells per well 72 h |
0.045–450 μg ml−1 |
r
1 = 0.37 mM−1 s−1 field: 3 T |
IC50 range from 0.36 mM of Mn in NCI–H460 cells to 4.73 mM of Mn in MRC-5 cells |
5
|
Manganese oxide dispersed MSNs |
MCF-7 cells (MTT assay) |
1 × 104 cells per well 36 h |
0–400 μg ml−1 |
r
1 = 2.28 mM−1 s−1 field: 3 T |
Very low cytotoxic effect, IC50 > 400 μg ml−1 |
8
|
Mn3O4 capped MSNs |
BxPC-3 cells (MTT assay) |
8 × 103 cells per well 72 h |
0–0.1 μg ml−1 |
r
1 = 13.39 mM−1 s−1, r1 = 25.17 mM−1 s−1 (reduced) field: 3 T |
Mn3O4 fairly low toxicity, but released Mn2+ ions highly toxic |
9
|
Poly(lactic-co-glycolic acid) |
RG2 cells (MTS assay) |
1 × 104 cells per well 48 h |
50 μM [Mn2+] |
r
1 = 0.21 mM−1 s−1, r1 = 7 mM−1 s−1 (dissolved) field: 4 T |
Slight toxic effect |
10
|
PEG-1-amino undecanoic acid |
M21 cells (SRB assay) |
1.5 × 103 cells per well 72 h |
0.005–3 mM |
r
1 = 1.44 mM−1 s−1 field: 9.4 T |
IC50 = 0.14 mM |
19
|
Mesoporous SiO2 |
Adipose-derived MSCs (MTS assay) |
5 × 103 cells per well 24 h |
7.6–68.6 μg ml−1 of Mn |
r
1 = 1.72 mM−1 s−1 field: 1.5 T |
>75% cell viability at 68.6 μg ml−1 of Mn |
22
|
Polystyrene sulfonate |
A549 cells (MTT assay) |
4 × 103 cells per well 24 h |
0–100 μg ml−1 |
r
1 = 2.06 mM−1 s−1 field: 3 T |
>90% cell viability |
35
|
Mesoporous SiO2–PEG |
HeLa cells (MTT assay) |
5 × 104 cells per well 24 h |
5–100 μg ml−1 |
r
1 = 0.17 mM−1 s−1 field: 3 T |
Almost 100% cell viability at 100 μg ml−1 |
39
|
DC-cholesterol and DOPE |
HEK293/LLC1/A549 cells (Presto Blue assay) |
72 h |
0–14 μg ml−1 |
r
1 = 1.17 mM−1 s−1 field: 7 T |
No apparent toxicity up to 14 μg ml−1 |
44
|
SiO2–PEG–aptamer |
HeLa/L02 cells (MTT assay) |
5 × 104 cells per well 12 and 24 h |
0–1000 μg ml−1 |
r
1 = 0.53 mM−1 s−1 field: 0.5 T |
>90% cell viability after 24 h at 1000 μg ml−1 |
45
|
SiO2–PEG |
HeLa/MCF-7 cells (MTT assay) |
24 h |
0–100 μg ml−1 |
r
1 = 0.49 mM−1 s−1 field: 0.5 T |
>80% cell viability at 100 μg ml−1 |
46
|
Dopamine–PEG–NH2 |
Caki-1 cells (WST-8 assay) |
1 × 105 cells per well 24 and 48 h |
25–100 μg ml−1 |
— |
Low toxicity; >90% cell viability at 100 μg ml−1 |
47
|
Dopamine–ssDNA |
Caki-1 cells (MTT assay) |
3 × 104 cells per well 12 h |
6–100 μg ml−1 |
— |
Increase in cell viability at 50 μg ml−1 15% cell death at 100 μg ml−1 |
48
|
SiO2–PEG/NH2 |
Bone marrow-derived DCs and PMNs (Annexin-V/PI or Nicoletti staining) |
2 × 105 cells per well 24 and 72 h |
0.1–30 μg ml−1 |
r
1 = 0.47 mM−1 s−1 field: 3 T |
Negligible cytotoxicity up to 30 μg ml−1 for 72 h |
49
|
4.1 Influence of nanocarrier composition
4.1.1 PEG–phospholipid micelles.
In the seminal publication first describing the use of MONs as T1 contrast agents for MRI (Fig. 7), Na et al. employed PEG–phospholipid micelles (DSPE–mPEG) to encapsulate MONs.5 The potential toxicity of these PEG–phospholipid coated MONs was subsequently investigated with eight different cell lines. It was observed that the IC50 value ranged from 0.36 mM of Mn with NCI-H460 cells to 4.73 mM of Mn with MRC-5 cells. ICP-AES suggested no appreciable Mn-ion leaching from the MONs when kept for 7 days at room temperature while the in vivo biocompatibility was demonstrated by an absence of abnormal behavioural changes or signs of weight loss in mice that were injected with the PEG–phospholipid coated MONs. Shin et al. have extended the system of PEG–phospholipid micelles to encapsulate hollow MONs, which were reported to be relatively non-cytotoxic to MCF-7 cell lines after 72 hours.36 Similarly, Howell et al. coated MONs with a phospholipid shell (DC-cholesterol and DOPE) surface-decorated with an outer corona of PEG polymers.44In vitro cytotoxicity was evaluated with HEK293 cells using the Presto Blue assay and the experimental results showed no apparent toxicity of the nanoparticles after 72 hours at a concentration of 14 μg ml−1.
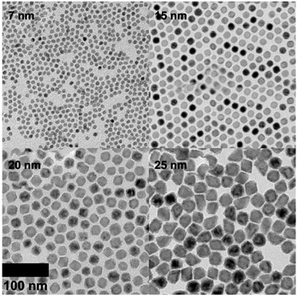 |
| Fig. 7 TEM images of PEG–phospholipid coated MONs with different particle sizes. Reprinted with permission from ref. 5. Copyright 2007 Wiley-VCH. | |
In general, these in vitro studies suggest that the PEG–phospholipid is useful to render MONs biologically compatible, as shown by the lack of observable cytotoxicity of MONs to the various human cell lines (normal and cancer). There was also no apparent MON-induced toxicity in mice in vivo. These findings could be attributed to the tight hydrophobic inner layer of the phospholipid micelles, which formed a strong protective barrier for the as-encapsulated MONs. Herein lies both the benefit and drawback: although the PEG–phospholipid coating enhances the physicochemical stability of MONs and significantly reduces the likelihood of Mn-ion leaching under oxidative conditions,5 it also prevents direct water interaction with the encapsulated MON which is otherwise required for efficient water exchange.40 As a result, the r1 relaxivity of PEG–phospholipid coated MONs tend to be inherently low.
4.1.2 Silica shell.
The second class of coating material involves a nanostructured silica shell (Fig. 8). While it may be difficult to alter the phospholipid structure without interfering with the micellar stability, the silica coating is advantageous in that it can be facilely modified by structural engineering techniques (e.g. generation of mesopores) to improve the r1 relaxivity.22 Yet, studies have indicated that such alterations to the physicochemical properties of nanostructured silica can dramatically impact its attendent toxicology.50,51 Therefore, besides overcoming the inherent toxicity and poor water solubility of MONs under physiological conditions, it is also imperative for the nanostructured silica shell alone to exhibit excellent biocompatibility.
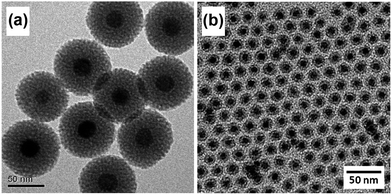 |
| Fig. 8 TEM images of MONs coated with a uniform shell of: (a) mesoporous silica; and (b) dense silica prepared by a w/o reverse microemulsion technique. Reprinted with permission from ref. 39. Copyright 2011 American Chemical Society. Reproduced from ref. 49 with permission from the Royal Society of Chemistry. | |
In this regard, toxicology of the silica shell is affected by a multitude of factors including the degree of condensation in silica, porosity and the type of ligand functionalization.51 For instance, it has been determined that the presence of surface silanol groups in amorphous silica can potentially compromise the cell membrane integrity by inducing the denaturation of membrane proteins.52 Consequently, the potential for cell lysis is highly correlated to the degree of condensation in silica which determines the ratio of Si–OH to Si–O–Si groups on the silica surface. Lin et al. also investigated multifunctional nanoparticles of different silica shell nanoarchitecture; it was indicated that a non-porous silica shell would cause greater hemolytic activity than the mesoporous counterparts.53 Interestingly, PEGylation has always been utilized for current designs of silica-coated MONs. Given that the resulting nanoparticles were generally reported to be well-tolerated in cell viability assays, it is likely that the effectiveness of PEGylation masks the toxic effects of silanol groups.
In addition, cytotoxicity studies have generally suggested that the silica shell coating is highly effective as a “capping” material to suppress the adverse effects of MONs on the cell viability. For example, Hu et al. encapsulated MONs within an amino-functionalized silica shell surface-conjugated with PEG chains.45 Based on a MTT assay with HeLa and L02 cells, it was shown that the nanoparticles had minimal cytotoxicity since a high cell viability of over 90% was maintained for 24 hours even at an elevated nanoparticle concentration of 1000 μg ml−1. Similar findings were reported by Yang et al. who synthesized PEGylated silica coated MONs with folate receptors.46
Besides, the silica coating also increases the resistance of MONs to degradation, thus it is effective to prevent Mn-ion leaching. In fact, Lee and co-workers reported that amongst the various coatings tested for core–shell structured MONs, the silica coating layer is most effective to reduce the likelihood of Mn-ion leaching.54 Mice toxicity studies were also carried out by Hu et al., where samples of major organs were taken at 10 days post-injection for histological examination.45 There were no indications that the nanoparticles caused any adverse effects in the animals as no apparent cellular or tissue damage was observed in any of the organs examined. There was also no inflammatory response observed in blood smears while kidney and hepatic function indicators were at the same levels as controls, thereby affirming the non-cytotoxic nature of the silica coated MONs. It is noteworthy that the unique properties of silica-coated MONs could also render them useful for many other applications. For example, due to the catalytically active nature of the Mn3O4 phase, silica-coated hollow MONs have been employed for the catalysis of cyanosilylation reactions with size and shape selectivity.37
4.1.3 Dopamine-based ligands.
Biocompatible ligands conjugated with dopamine moieties are an alternative class of coating material to functionalize MONs for applications as T1 MRI contrast agents. This strategy has received surging interest among the scientific community because dopamine has proven to be a unique catechol anchor group that has a strong binding affinity to metal oxide nanoparticles.55 Schladt and co-workers synthesized highly water-dispersible MONs through surface functionalization with a dopamine–PEG–protoporphyrin (DA–PEG–PP) ligand.47 MTT assay of the DA–PEG–PP functionalized MONs confirmed a low level of cytotoxicity as a high cell viability of over 90% was observed after 48 hour incubation at a concentration of 100 μg ml−1. Shukoor et al. also immobilized ssDNA (CpG ODN 2006) onto MONs successfully by using a dopamine-based polymeric ligand with a reactive amine group for the coupling of ssDNA.48 The resultant nanoparticles were relatively non-toxic with concentrations up to 50 μg ml−1.
Indeed, dopamine has been commonly conjugated to biocompatible polymers or proteins so as to immobilize these functional molecules onto MONs.42,47,48 However, it has also been reported the catechol unit in dopamine tends to oxidize under aerobic conditions to form dark insoluble polymers,55 thereby affecting the binding stability between dopamine and the metal oxide surface. Consequently, the dopamine-based ligand coating may be labile and degrading in water and biological fluids, thereby exposing the MON core complex to dissolution. This was verified by Schladt et al., who showed the susceptibility of DA-PEG coated MONs to Mn-ion leaching since TEM images of the MONs had appeared rougher and less homogeneous after the leaching experiments.49
4.1.4 Polymer ligands.
A number of in vitro and in vivo studies have been conducted to assess the toxicity of MONs stabilized by polymeric ligands. Gallo et al. displaced the oleic acid surface ligands of MONs with 1-aminoundecanoic acid, followed by PEG coupling for better biocompatibility.19In vitro toxicity assays indicated an IC50 value of 0.14 mM of Mn for the nanoparticles, which is higher than the level required for imaging in vivo. On the other hand, Huang et al. employed polystyrene sulfonate polymer to replace the hydrophobic surfactants of the MONs.35 MTT assay revealed that the cell viability remained high (>90%) even at a high Mn concentration of 1.3 mM for 1 day. The negatively charged polymeric coating was also effective in preventing Mn-ion leaching, as ICP-AES analysis indicates that only a very small amount of free Mn2+ ions (∼1.3%) was produced upon incubation in PBS solution at 37 °C for 48 hours. From these studies, it can be seen that engineered MONs with polymeric ligand can have vastly different outing coating bioactivity, depending on the type of polymers used. Hence, each type of MONs in this classification should be characterized individually for their potential toxicity and ADME properties. Nonetheless, based on the Pearson acid base (HSAB) concept, oxygen containing polymers would be particularly favoured because they could exhibit a high binding affinity to MONs.55 As a result, a compact coating layer is achieved for more effective water–manganese interactions.
4.2 Influence of MON core morphology
The effect on r1 relaxivity by changing the particle size and shape of MONs has been well-established. However, the interplay between MON morphology and toxicity is less commonly discussed. Firstly, given that smaller nanoparticles have a larger surface-to-volume ratio and higher surface reactivity, it is likely that smaller sized MONs are potentially more cytotoxic due to their greater susceptibility to Mn-ion leaching. Moreover, they would be more prone to aggregation, especially in the presence of serum proteins in the cell culture medium. Such instability in the cell medium would affect the cellular uptake of MONs during in vitro assays and alter the toxicological profile of MONs accordingly. Secondly, based on studies on other inorganic nanoparticles, the toxicity of MONs is also affected by their shape and morphology. For example, it was observed that the transferrin-coated rod-shaped gold nanoparticles were less readily internalized by HeLa cells as compared to their spherical counterparts.56 As a result, conclusions about the toxic potential of the nanoparticles would be misleading since the high cell viability arose from a decreased cellular uptake of nanoparticles instead of an improved biocompatibility of the nanoparticles. On the other hand, it has been shown that for TiO2 nanoparticles with high aspect ratios, they may cause shape-induced toxicity by lysosomal disruption.57 Certainly, the nano-bio interactions of MONs with varying shapes may be different, nonetheless it highlights the importance of elucidating the potential impact of particle shape on the cytotoxicity assessment of MONs.
5. Pharmacokinetics of MONs
Examining the cytotoxicity of MONs alone is inadequate for the risk assessment of MONs as T1 MRI contrast agents for clinical use. It is also of paramount importance to understand the ADME characteristics of MONs in the living system. This will enable us to discern how long the nanoparticles will be present within the body, as well as to predict the organs or tissues in which they distribute. In this regard, the following section seeks to consider several key parameters to assess the in vivo nanotoxicological profile of MONs.
5.1 Opsonisation and blood circulation time
While there has been one instance of the intranasal administration of MONs in the form of nasal drops,44 most in vivo studies involve an intravenous injection of MONs. Hence, the absorption is fairly straightforward and bioavailability is often near 100% since the administered dose of MONs is directly introduced into the systemic circulation. However, one of the most critical issues in association with the intravenous route of administration is the possible binding of opsonins to the nanoparticles which would facilitate their capture by macrophages in the bloodstream.55 This is a major obstacle because it reduces the blood circulation time of MONs and prevents a clinically feasible scan time for high spatial MRI resolution.
The degree of opsonisation is affected by the overall size of nanoparticles. Larger nanoparticles (>200 nm) tend to be quickly recognized and eliminated by the reticuloendothelial system (RES). In this regard, one significant challenge for using MSNs as a platform to disperse MONs lies in the large size of the mesoporous framework, which typically ranges in the order of several hundreds of nm.14 Chen et al. investigated the biodistribution of Mn–MSNs injected into mice,8 which was observed to rapidly accumulate in the liver and spleen; such enhanced uptake suggests that the nanoparticles were highly susceptible to splenic filtration and be removed from circulation in the blood. Comparatively, the hydrodynamic size of core–shell structured MONs is usually smaller to avoid fast RES uptake, yet large enough to be above the renal clearance threshold of 5.5 nm. Hence, they are more suited for long circulation nanoparticle formulation and can be used to achieve site-specific accumulation in designated areas such as tumors. For example, Hu et al. have demonstrated the ability of silica coated MONs to function as tumour-specific MRI contrast agents by determining their biodistribution in tumour-bearing mice.45 Majority of the nanoparticles were seen to accumulate in the tumour, with smaller amount taken up in the liver and spleen.
The likelihood of opsonisation is also strongly correlated to the length of PEG molecules conjugated to MONs. Gallo et al. investigated the bioaccumulation of PEGylated MONs in the liver, spleen and gall bladder by analysing the Mn content of the various tissues harvested from mice.19 It was revealed that nanoparticles which were functionalised with longer PEG chains (5000 Da) exhibited a greater degree of accumulation at longer time duration than that of their shorter PEG counterparts (600 Da). This indicates that having a longer PEG length is effective to prevent adsorption of serum proteins, hence prolonging the blood circulation of MONs before their eventual uptake into the liver and spleen.
5.2 Biodistribution and clearance
Several in vivo studies have suggested that the biodistribution of core–shell structured MONs are fairly similar despite the variation in coating material used. Howell et al. showed that the highest accumulation of PEG–phospholipid coated MONs was detected in the liver after 24 hours post-injection.44 Yang et al., who traced the fate of silica coated MONs in mice 4 hours post-injection, also reported similar observations.46 Such findings could be attributed to the coating material thickness, which necessarily increased the hydrodynamic diameter. Dense PEGylation might increase blood half-life, but it also increases the hydrodynamic diameter beyond the renal filtration threshold and precludes renal excretion from the body. Therefore, despite the systemic circulation of the nanoparticles to all bodily organs, the liver is observed to be the target organ of higher nanoparticle accumulation. Nonetheless, discrepancies in the literature exist. Chevallier et al. reported the presence of low Mn content in the liver and spleen by neutron activation analysis.58 Instead, the PEGylated MONs were found to accumulate primarily in the faeces, intestine, gall bladder, kidneys and stomach, in decreasing order of magnitude. This suggests that the uptake of nanoparticles by the mononuclear phagocyte system is relatively negligible. Such atypical biodistribution data was attributed to the ultra small dimensions of MONs (6–8 nm diameter), which were also grafted with highly hydrophilic PEGylated bis-phosphonate dendrons (PDn) for the smallest possible hydrodynamic diameter. Moreover, it was indicated that over 70% of the injected dose was eliminated from the body within 48 hours as PDn grafting could be applied advantageously to confer fast renal clearance of the MONs.
As has been identified in several in vivo studies, the liver is likely the main organ for the clearance of nanoparticles from the blood circulation. For example, the concentration of silica coated MONs in the liver of mice was observed to peak at 12 hour post-injection before decreasing considerably by the 24 hour time point.45 Howell et al. also reported that the highest accumulation of PEG–phospholipid coated MONs was detected in the liver after 24 hours, before declining significantly to the control levels by the 96 hour time point.44 To account for a possible route of excretion in the liver, a distinction should first be made between the two major cell types of the liver: the Kupffer cells and the hepatocytes. Due to the particulate nature of MONs, they are likely to be engulfed by the Kupffer cells of the liver, which forms part of the mononuclear phagocyte system. This is usually undesirable as the phagocytized material is expected to remain in the body for a long period of time and increases the likelihood of toxicity. However, once in the Kupffer cells, the nanoparticles would be shuttled to the low pH compartments (acidic endosomes) within the cells. This results in the dissolution of MONs to release Mn2+ ions, which could subsequently be taken up by the adjacent hepatocytes and be excreted through the biliary pathway. Hence, the MONs are unlikely to present problems with body burdens, as verified by the absence of MON accumulation in the liver at longer times.
5.3 Interaction with blood components
Upon entry into the body, the intravenously injected MONs would interact with the various blood components including the red blood cells and platelets. Hence, from a toxicological perspective, the MONs should not compromise the red blood cell membrane and induce the rupture of red blood cells. Otherwise, the leakage of haemoglobin could potentially lead to adverse health effects such as anaemia or hypohepatia. In vitro hemolysis assay indicates that the hemolysis potential of MONs was 2.85%.59 This is less than the 5% limit criterion in ASTM E2524-08, which is the standard test method for analysis of hemolytic properties of nanoparticles. Thus, it indicates that the water-dispersible MONs exhibit good hemocompatibility. Another important consideration for the toxicity profile of MONs is the particle susceptibility to trigger the inflammatory response in the body. Herein, by measuring the secretion levels of inflammatory cytokines such as interleukin 12 (IL-12) or 6 (IL-6), Schladt et al. quantified that silica coated MONs were not able to activate the inflammatory bone marrow-derived dendritic cells.49 This indicated that the injected MONs would not exert any toxic effect in cells.
6. Conclusions
The development of MONs as T1 MRI contrast agents is a burgeoning field of research with huge prospects for medical imaging applications. To achieve higher r1 relaxivities of MONs, current design tenets include increasing the surface-to-volume ratio of MONs and water permeability of the nanocarriers, as well as optimizing the rotational correlation time of the paramagnetic payload. Moreover, the recent and present trends in this field are to further investigate in vivo biocompatibility, biodistribution, targeting efficiency, toxicity, biodegradability and clearance of MONs in animal models before their translation into clinical trials. Herein, the studies reviewed here suggest several key points, in particular, that the toxicity and ADME characteristics of MONs are dependent on the structure of the nanomaterial system and the type of coating materials used. To optimize the nanotoxicity evaluation of MONs, it is also necessary to thoroughly characterize the nanomaterial properties. In addition, besides an in vitro assessment of the MONs for their short-term cell viability, emphasis should also be placed on in vivo outcomes such as the opsonisation and biodistribution of the nanoparticles upon intravenous administration.
References
- H. B. Na, I. C. Song and T. Hyeon, Adv. Mater., 2009, 21, 2133 CrossRef CAS.
- D. Pan, S. D. Caruthers, A. Senpan, A. H. Schmieder, S. A. Wickline and G. M. Lanza, Wiley Interdiscip. Rev.: Nanomed. Nanobiotechnol., 2011, 3, 162 CAS.
- Z. Zhen and J. Xie, Theranostics, 2012, 2, 45 CrossRef CAS PubMed.
- J. Kim, Y. Piao and T. Hyeon, Chem. Soc. Rev., 2009, 38, 372 RSC.
- H. B. Na, J. H. Lee, K. An, Y. I. Park, M. Park, I. S. Lee, D. H. Nam, S. T. Kim, S. H. Kim, S. W. Kim, K. H. Lim, K. S. Kim, S. O. Kim and T. Hyeon, Angew. Chem., Int. Ed., 2007, 46, 5397 CrossRef CAS PubMed.
- T. Kim, E. J. Cho, Y. Chae, M. Kim, A. Oh, J. Jin, E. S. Lee, H. Baik, S. Haam, J. S. Suh, Y. M. Huh and K. Lee, Angew. Chem., Int. Ed., 2011, 50, 10589 CrossRef CAS PubMed.
- Y. Chen, Q. Yin, X. Ji, S. Zhang, H. Chen, Y. Zheng, Y. Sun, H. Qu, Z. Wang, Y. Li, X. Wang, K. Zhang, L. Zhang and J. Shi, Biomaterials, 2012, 33, 7126 CrossRef CAS PubMed.
- Y. Chen, H. Chen, S. Zhang, F. Chen, S. Sun, Q. He, M. Ma, X. Wang, H. Wu, L. Zhang, L. Zhang and J. Shi, Biomaterials, 2012, 33, 2388 CrossRef CAS PubMed.
- A. Wang, M. Guo, N. Wang, J. Zhao, W. Qi, F. Muhammad, L. Chen, Y. Guo, N. T. Nguyen and G. Zhu, Nanoscale, 2014, 6, 5270 RSC.
- M. F. Bennewitz, T. L. Lobo, M. K. Nkansah, G. Ulas, G. W. Brudvig and E. M. Shapiro, ACS Nano, 2011, 5, 3438 CrossRef CAS PubMed.
- Y. Chen, P. Xu, Z. Shu, M. Wu, L. Wang, S. Zhang, Y. Zheng, H. Chen, J. Wang, Y. Li and J. Shi, Adv. Funct. Mater., 2014, 24, 4386 CrossRef CAS.
- J. Crossgrove and W. Zheng, NMR Biomed., 2004, 17, 544 CrossRef CAS PubMed.
- H. Yan, C. Teh, S. Sreejith, L. Zhu, A. Kwok, W. Fang, X. Ma, K. T. Nguyen, V. Korzh and Y. Zhao, Angew. Chem., Int. Ed., 2012, 51, 1 CrossRef.
- J. M. Rosenholm, C. Sahlgren and M. Lindén, Nanoscale, 2010, 2, 1870 RSC.
- A. J. Di Pasqua, K. K. Sharma, Y. L. Shi, B. B. Toms, W. Ouellette, J. C. Dabrowiak and T. Asefa, J. Inorg. Biochem., 2008, 102, 1416 CrossRef CAS PubMed.
- L. Yildirimer, N. T. K. Thanh, M. Loizidou and A. M. Seifalian, Nano Today, 2011, 6, 585 CrossRef CAS PubMed.
- J. Li, Z. Zhao, J. Feng, J. Gao and Z. Chen, Nanotechnology, 2013, 24, 455102 CrossRef PubMed.
- T. D. Schladt, T. Graf and W. Tremel, Chem. Mater., 2009, 21, 3183 CrossRef CAS.
- J. Gallo, I. S. Alam, I. Lavdas, M. Wylezinska-Arridge, E. O. Aboagye and N. J. Long, J. Mater. Chem. B, 2014, 2, 868 RSC.
- Y. Zhang, B. Y. W. Hsu, C. Ren, X. Li and J. Wang, Chem. Soc. Rev., 2015, 44, 315 RSC.
- B. Y. W. Hsu, M. Wang, Y. Zhang, V. Vijayaragavan, S. Y. Wong, A. Y. C. Chang, K. K. Bhakoo, X. Li and J. Wang, Nanoscale, 2014, 6, 293 RSC.
- T. Kim, E. Momin, J. Choi, K. Yuan, H. Zaidi, J. Kim, M. Park, N. Lee, M. T. McMahon, A. Q. Hinojosa, J. W. M. Bulte, T. Hyeon and A. A. Gilad, J. Am. Chem. Soc., 2011, 133, 2955 CrossRef CAS PubMed.
- W. Xu, K. Kattel, J. Y. Park, Y. Chang, T. J. Kim and G. H. Lee, Phys. Chem. Chem. Phys., 2012, 14, 12687 RSC.
- R. Deng, X. Xie, M. Vendrell, Y.-T. Chang and X. Liu, J. Am. Chem. Soc., 2011, 133, 20168 CrossRef CAS PubMed.
- Z. Zhao, H. Fan, G. Zhou, H. Bai, H. Liang, R. Wang, X. Zhang and W. Tan, J. Am. Chem. Soc., 2014, 136, 11220 CrossRef CAS PubMed.
- Y. Li, X. Tian, Z. Lu, C. Yang, G. Yang, X. Zhou, H. Yao, Z. Zhu, Z. Xi and X. Yang, J. Nanosci. Nanotechnol., 2010, 10, 397 CrossRef CAS PubMed.
- G. H. Im, S. M. Kim, D.-G. Lee, W. J. Lee, J. H. Lee and I. S. Lee, Biomaterials, 2013, 34, 2069 CrossRef CAS PubMed.
- T. D. Schladt, M. I. Shukoor, K. Schneider, M. N. Tahir, F. Natalio, I. Ament, J. Becker, F. D. Jochum, S. Weber, O. Köhler, P. Theato, L. M. Schreiber, C. Sönnichsen, H. C. Schröder, W. E. G. Müller and W. Tremel, Angew. Chem., Int. Ed., 2010, 49, 3976 CrossRef CAS PubMed.
- N. Glaser, D. J. Adams, A. Böker and G. Krausch, Langmuir, 2006, 22, 5227 CrossRef CAS PubMed.
- I. Schick, S. Lorenz, D. Gehrig, A.-M. Schilmann, H. Bauer, M. Panthöfer, K. Fischer, D. Strand, F. Laquai and W. Tremel, J. Am. Chem. Soc., 2014, 136, 2473 CrossRef CAS PubMed.
- P. Caravan, C. T. Farrar, L. Frullano and R. Uppal, Contrast Media Mol. Imaging, 2009, 4, 89 CrossRef CAS PubMed.
- E. Belorizky, P. H. Fries, L. Helm, J. Kowalewski, D. Kruk, R. R. Sharp and P.-O. Westlund, J. Chem. Phys., 2008, 128, 052315 CrossRef PubMed.
- D. Kruk and J. Kowalewski, J. Chem. Phys., 2009, 130, 174104 CrossRef CAS PubMed.
- N. Bloembergen and L. O. Morgan, J. Chem. Phys., 1961, 34, 842 CrossRef CAS.
- C. C. Huang, N. H. Khu and C. S. Yeh, Biomaterials, 2010, 31, 4073 CrossRef CAS PubMed.
- J. Shin, R. M. Anisur, M. K. Ko, G. H. Im, J. H. Lee and I. S. Lee, Angew. Chem., Int. Ed., 2009, 48, 321 CrossRef CAS PubMed.
- R. M. Anisur, J. Shin, H. H. Choi, K. M. Yeo, E. J. Kang and I. S. Lee, J. Mater. Chem., 2010, 20, 10615 RSC.
- B. Y. W. Hsu, M. Ng, Y. Zhang, S. Y. Wong, K. Bhakoo, X. Li and J. Wang, Adv. Funct. Mater., 2015, 25, 5269 CrossRef CAS.
- Y. K. Peng, C. W. Lai, C. L. Liu, H. C. Chen, Y. H. Hsiao, W. L. Liu, K. C. Tang, Y. Chi, J. K. Hsiao, K. E. Lim, H. E. Liao, J. J. Shyue and P. T. Chou, ACS Nano, 2011, 5, 4177 CrossRef CAS PubMed.
- R. Xing, F. Zhang, J. Xie, M. Aronova, G. Zhang, N. Guo, X. Huang, X. Sun, G. Liu, L. H. Bryant, A. Bhirde, A. Liang, Y. Hou, R. D. Leapman, S. Sun and X. Chen, Nanoscale, 2011, 3, 4943 RSC.
- Z. Cheng, D. L. J. Thorek and A. Tsourkas, Adv. Funct. Mater., 2009, 19, 3753 CrossRef CAS PubMed.
- J. Huang, J. Xie, K. Chen, L. Bu, S. Lee, Z. Cheng, X. Li and X. Chen, Chem. Commun., 2010, 46, 6684 RSC.
- H. F. Krug, Angew. Chem., Int. Ed., 2014, 53, 12304 CAS.
- M. Howell, J. Mallela, C. Wang, S. Ravi, S. Dixit, U. Garapati and S. Mohapatra, J. Controlled Release, 2013, 167, 210 CrossRef CAS PubMed.
- H. Hu, A. Dai, J. Sun, X. Li, F. Gao, L. Wu, Y. Fang, H. Yang, L. An, H. Wu and S. Yang, Nanoscale, 2013, 5, 10447 RSC.
- X. Yang, Z. Zhou, L. Wang, C. Tang, H. Yang and S. Yang, Mater. Res. Bull., 2014, 57, 97 CrossRef CAS.
- T. D. Schladt, K. Schneider, M. I. Shukoor, F. Natalio, H. Bauer, M. N. Tahir, S. Weber, L. M. Schreiber, W. E. G. Müller and W. Tremel, J. Mater. Chem., 2010, 20, 8297 RSC.
- M. I. Shukoor, F. Natalio, M. N. Tahir, M. Wiens, M. Tarantola, H. A. Therese, M. Barz, S. Weber, M. Terekhov, H. C. Schröder, W. E. G. Müller, A. Janshoff, P. Theato, R. Zentel, L. M. Schreiber and W. Tremel, Adv. Funct. Mater., 2009, 19, 3717 CrossRef CAS.
- T. D. Schladt, K. Koll, S. Prüfer, H. Bauer, F. Natalio, O. Dumele, R. Raidoo, S. Weber, U. Wolfum, L. M. Schreiber, M. P. Radsak, H. Schild and W. Tremel, J. Mater. Chem., 2012, 22, 9253 RSC.
- A. Liberman, N. Mendez, W. C. Trogler and A. C. Kummel, Surf. Sci. Rep., 2014, 69, 132 CrossRef CAS PubMed.
- A. E. Garcia-Bennett, Nanomedicine, 2011, 6, 867 CrossRef CAS PubMed.
- C. Fruijtier-Pölloth, Toxicology, 2012, 294, 61 CrossRef PubMed.
- Y.-S. Lin and C. L. Haynes, Chem. Mater., 2009, 21, 3979 CrossRef CAS.
- Y. C. Lee, D. Y. Chen, S. J. Dodd, N. Bouraoud, A. P. Koretsky and K. M. Krishnan, Biomaterials, 2012, 33, 3560 CrossRef CAS PubMed.
- T. D. Schladt, K. Schneider, H. Schild and W. Tremel, Dalton Trans., 2011, 40, 6315 RSC.
- B. D. Chithrani and W. C. Chan, Nano Lett., 2007, 7, 1542 CrossRef CAS PubMed.
- R. F. Hamilton, N. Wu, D. Porter, M. Buford, M. Wolfarth and A. Holian, Part. Fibre Toxicol., 2009, 6, 35 CrossRef PubMed.
- P. Chevallier, A. Walter, A. Garofalo, I. Veksler, J. Lagueux, S. Bégin-Colin, D. Felder-Flesch and M. A. Fortin, J. Mater. Chem. B, 2014, 2, 1779 RSC.
- Y. Lu, L. Zhang, J. Li, Y.-D. Su, Y. Liu, Y.-J. Xu, L. Dong, H.-L. Gao, J. Lin, N. Man, P.-F. Wei, W.-P. Xu, S.-H. Yu and L.-P. Wen, Adv. Funct. Mater., 2013, 23, 1534 CrossRef CAS.
|
This journal is © The Royal Society of Chemistry 2016 |
Click here to see how this site uses Cookies. View our privacy policy here.