DOI:
10.1039/C6RA06572D
(Paper)
RSC Adv., 2016,
6, 45452-45461
The influence of the carbohydrate anomeric linkage on the free radical scavenging activity of enzymatically-synthesized phenolic glycosides†
Received
11th March 2016
, Accepted 2nd May 2016
First published on 4th May 2016
Abstract
Phenolic glycosides, widely recognized for their nutraceutical properties exist in nature as α- and β-glycosides. Interestingly, the β-phenolic glycosides are the predominant form in natural products. In this work, the pure α- and β-glucosides and galactosides of homovanillyl alcohol (HVA) were enzymatically synthesized. Their free radical scavenger (FRS) activity was evaluated using the DPPH˙ endpoint assay. According to the EC50 values, all glycosides are better FRSs than the corresponding aglycone, with lower EC50 values found for the β-anomers. In contrast, a DPPH˙ kinetic analysis revealed that HVA-aglycone is more reactive than the glycosides. The analysis based on both EC50 and kinetic behaviour suggested that, although the HVA-aglycone is kinetically a better free radical scavenger, glycosidation renders HVA-aglycone more stable for long-term reactions. The molecular electrostatic potential and conformational analysis of α- and β-HVA glycosides demonstrated that β-HVA glycosides are better free radical scavengers than the corresponding α-anomers. In fact, the higher flexibility of β-HVA glycosides allow the exposure of advantageously negatively charged regions favouring their FRS capacity.
Introduction
Glycosides result from the condensation of a carbohydrate and a non-sugar moiety (aglycone) such as an amino acid, a lipid, a nucleotide, a phenolic compound, an alkaloid, or a terpenoid. The carbohydrate moiety in glycosides is significant in nature because it modulates the aglycone function, its pharmacokinetics, its nutraceutical activity and in some cases serves for molecular recognition, particularly in secondary metabolites.1 In fact, the most relevant effect of carbohydrates in glycoside molecules has been related to the modification of physicochemical properties such as solubility, stability and volatility.2–4 From a functional point of view, glycoside pharmacological activities, such as antitumoral, antiviral, anti-inflammatory, antimicrobial, free radical scavenging, or oxidative DNA damage protection, have been associated to the aglycone molecule.3–7
Phenolic glycosides (PGs) are an important class of glycosides, very common in plants, where their activity has been associated to cellular damage response and protection against stress.8 The aglycones in PGs are in general phenolic compounds (PCs) such as phenolic acids and phenolic alcohols, or polyphenols such as flavonoids, tannins, stilbenes, curcuminoids, coumarins, lignans and quinones. PCs in the form of aglycones usually show low solubility and chemical instability. In fact, in their natural environment they exist mainly in the glycosylated form, a chemical condition that improves their function and distribution inside the plant.9,10
A better understanding of the structure–function relationship of PGs, particularly those structural features associated to their free radical scavenger activity, is required for a rational synthesis of novel PGs with enhanced properties. Although it has been widely accepted that PGs functional properties are influenced by the nature of the PC, the effect of the number and nature of the sugar moieties as well as the type of linkage to the aglycone has received less attention. Indeed, while the presence of phenolic hydroxyls in aglycones, notably in the form of an ortho-diphenolic arrangement has been recognized as a requirement for an efficient free radical scavenger activity of the PGs, little is known concerning the requirements in the carbohydrate moiety.11–13 In recent years, we reported the enzymatic synthesis of a series of phenyl propanoid glycosides (PPGs) demonstrating that, from a mechanistic point of view, their radical scavenging activity is associated to the PC when analysed against 2,2-diphenyl-1-picrylhydrazyl radical (DPPH˙). From a Sequential Proton Loss Electronic Transfer mechanism, we came to the conclusion that the position of the hydroxyl group in these PPGs has an important effect on their scavenging activity.14 Nevertheless, not only aglycones may be responsible for the PGs free radical scavenger activity but, as recently reported, some carbohydrates such as glucose (Glc), sucrose, fructose, 1-kestotriose, inulin and trehalose may also act as good scavengers of the hydroxyl and superoxide radicals.15
In spite of the lack of knowledge concerning the structural characteristics that explain the contribution of carbohydrates to the free radicals scavenger activity of glycosides, it is a fact that glucose is frequently found as the first sugar linked to the aglycone in glycosylated PGs.11 An important additional feature is the eventual influence of the anomeric configuration. In fact, it has been reported that more than 80% of plant PGs exist as β-anomers,16–19 a configuration that makes them more stable in nature, where α-glucosidase activity is common and easily assimilated by humans through β-glucosidase activity of the gut microbiota.20,21
The contribution of the anomeric configuration to the biological and physical functions of carbohydrates has been widely recognized. For instance, crucial differences in functional properties of disaccharides are observed between maltose (α-glucose disaccharide), cellobiose (β-glucose disaccharide) and other disaccharides, commonly attributed to the flexibility and/or rigidity conferred to the molecules by the anomeric bond.22,23 However, as far as glycosides is concerned, information explaining how flexibility and/or rigidity impacts functionality in PGs, in particular, their free radical scavenger properties, is scarce. An exemption to this fact is the recent report by Sánchez et al.,24 describing the close relationship between flexibility in caffeic acid esters and amides, and its free radical scavenging capacity against DPPH˙.
In this work the effect of the sugar nature and the anomeric linkage (α and β anomers) on the free radical scavenging activity is reported. For this purpose, four different glycosides of homovanillyl alcohol (HVA) as aglycone were enzymatically synthesized (Scheme 1) and evaluated against DPPH˙ assay. As far as the sugar nature is concerned, glucose and galactose (Gal), the two most frequently found sugars in natural glycosides were selected. Results are reported in terms of both, the classical 50% inhibitory concentration parameter (EC50) and a first order rate constant, obtained from a simplified pseudo-first order kinetic model. Moreover, theoretical calculations and a conformational analysis are reported in order to explain the effects of the anomeric linkage on the radical scavenging activity.
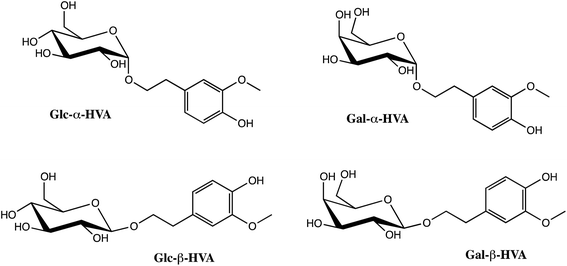 |
| Scheme 1 Glucosides (Glc) and galactosides (Gal) of homovanillyl alcohol (HVA) in α (Glc-α-HVA and Gal-α-HVA) and β (Glc-β-HVA and Gal-β-HVA) configuration. | |
Materials and methods
Commercially available enzymes were used in glycoside synthesis. Cyclodextrin-glucanotransferase (CGTase) from Thermoanaerobacter sp. was kindly provided by Novozymes A/C (Novozymes México, Mexico City); glucoamylase from Aspergillus niger was purchased from Enzymes Development Corporation (New York, NY, USA), while β-galactosidase from Kluyveromyces lactis (3000 LAU mL−1) from Maxilact (DSM Food Specialties, the Netherlands). Methanol, 2-methyl-2-propanol and acetonitrile HPLC grade, were purchased to J.T. Baker (Phillipsburg, NJ, USA); α-galactosidase from green coffee beans, homovanillyl alcohol (HVA), 6-hydroxy-2,5,7,8-tetramethylchroman-2-carboxylic acid and 2,2-diphenyl-1-picrylhydrazyl (DPPH˙) were all from Aldrich (WI, USA); β-ciclodextrin, fluorescein, starch, and cellobiose were from Sigma Chemical Co. (St. Louis, MO, USA). The enzyme β-glucosidase from Thermotoga maritima was obtained through recombinant strategies using the genome sequence provided by Dr Enrique Morett (IBT-UNAM, México) and cloned into plasmid pET-22b(+). E. coli C-43 was transformed with this construction. IPTG (0.2 mM) was used as inductor of gene expression during the 6 hours culture carried out at 37 °C in YT2x medium (triptone 16 g L−1; yeast extract 10 g L−1; NaCl 5 g L−1) agitated at 250 rpm. After cell disruption the recombinant enzyme in the protein extract was purified through an affinity chromatography column on a Ni-chelating column (HiTrap®Chelating HP column from Amersham Pharmacia Biotech). The proteins were eluted with a linear gradient (0–100%) with NaH2PO4
:
NaCl (20 mM
:
0.5 M) buffer containing 0.5 M imidazole as eluent.
Enzymatic synthesis and purification of glycosides
Glc-β-HVA. Glc-β-HVA (2-[4-hydroxy-3-methoxyphenyl]ethyl-β-D-glucopyranosyde) was synthesized with β-glucosidase from Thermotoga maritima at a concentration of 5 U mL−1. β-Glucosidase of Thermotoga maritima with cellobiose (0.8 M) as glucosyl donor and HVA (0.8 M) as acceptor in pH 7, 100 mM Na2HPO4 buffer. Reactions were incubated at 70 °C for 24 hour with samples taken from the reaction mixture at relevant time intervals, heated for 10 min at 100 °C to stop the reaction and centrifuged for 10 min at 1150 × g at 25 °C to eliminate suspended solids. The Glc-β-HVA was purified using a Flash Chromatography method on a Reveleris® equipment (GRACE®, Columbia, MD, USA). First, the samples were extracted from the crude reaction twice with chloroform in order to eliminate residual HVA. Afterwards, the products in the residual aqueous phases were extracted with 2-methyl-2-butanol (2M2B), which was then adsorbed onto silica gel (230–400 mesh, 0.04–0.063 mm) and evaporated. An Amino GRACE® column (12 g, 0.04 mm) was used as the stationary phase using an ethyl acetate
:
methanol gradient for elution, starting with ethyl acetate and increasing the methanol content to reach 45% (v/v) in 8 minutes, followed by 3 min of isocratic flow with the 45% methanol solvent at a flow rate of 20 mL min−1. The eluted products were identified with an UV detector at 280 nm. The yield of Glc-β-HVA quantified by HPLC was 24% and 10% after purification, both on the basis of HVA converted.
Gal-β-HVA. Gal-β-HVA (2-[4-hydroxy-3-methoxyphenyl]ethyl-β-D-galactopyranosyde) was synthesized as previously reported by López-Munguía et al.,14 using β-galactosidase from Kluveromices lactis (12 U mL−1) as biocatalyst with lactose as galactosyl donor (1 M), HVA (250 mM) as acceptor in 50 mM, pH 6.5 phosphate buffer at 35 °C. The products of both galactosylation reactions were analysed either by TLC using ethyl acetate/metanol/water (24
:
4
:
3 v/v/v) as a solvent or by HPLC (see later). Samples were stored at −18 °C until purification. The purification of Gal-β-HVA was carried out under the same conditions described above for β-glucoside of HVA. The yield of Gal-β-HVA quantified by HPLC was 47% and 30% after purification, both on the basis of HVA converted.
Glc-α-HVA. Glc-α-HVA (2-[4-hydroxy-3-methoxyphenyl]ethyl-α-D-glucopyranoside) was synthesized with CGTase of Thermoanaerobacter (20 U mL−1) using starch 10% w/v (0.1 M) as glucosyl donor and 1 M of HVA as acceptor in a pH 6.0, 50 mM Na2HPO4 buffer. Reactions were performed at 50 °C with continuous stirring. After 24 hours the reaction was heated for 5 min at 100 °C to stop the reaction. In order to transform all the glucosylated products to the monoglucosylated form, the reaction mixture was treated with 258 U mL−1 of glucoamylase from Aspergillus niger during 48 hours at 50 °C. Aliquots of the reaction mixture were withdrawn at relevant time intervals, heated for 5 min at 100 °C to stop the reaction and centrifuged for 10 min at 1150 × g at 25 °C to eliminate suspended solids. The purification of Glc-α-HVA was carried out under the same conditions described above for β-glycosides but and additional purification step was needed. The second purification step consisted of a C18 GRACE® column (12 g, 0.04 mm) eluted with a 0
:
100 to 40
:
60 methanol
:
water gradient. The products in this step were also identified with UV detector at 280 nm. The yield of Glc-α-HVA quantified by HPLC was 17% and 8% after purification, both on the basis of HVA converted.
Gal-α-HVA. Gal-α-HVA (2-[4-hydroxy-3-methoxyphenyl]ethyl-α-D-galactopyranoside) was synthesized by α-galactosidase from green coffee beans (20 U mL−1) with 2-nitrophenyl α-D-galactopyranoside (0.2 M) as galactosyl donor and HVA (0.1 M) as acceptor in 50 mM, pH 6.5 malic acid buffer containing 20% v/v acetonitrile (20%) as the reaction medium. Reactions were incubated at 27 °C with stirring for 24 hours. At the end of the reaction the crude of reaction was heated for 5 min at 100 °C to stop the reaction and centrifuged for 10 min at 1150 × g at 25 °C to eliminate suspended solids. The purification of Gal-α-HVA was carried with the two step purification strategy described above for α-glucoside. The yield of Gal-α-HVA quantified by HPLC was 5% and 3% after purification, both on the basis of HVA converted.
HPLC analysis of HVA glycosides
The glycosides were analysed by HPLC using a Waters 600E system controller (Waters Corp., Milford, MA) at 35 °C, equipped with both a Waters 996 photodiode array detector and a refraction index detector (Waters Corp., Milford, MA). A Kromasil NH2 column (4.6 mm × 250 mm, 5 μm; Sigma-Aldrich, USA) was used for separation, using an isocratic phase of acetonitrile/water (80
:
20) as a mobile phase at a flow rate of 1 mL min−1.
NMR and HRMS analysis of HVA glycosides
HRMS FAB + spectra in a matrix of m-nitrobenzyl alcohol were recorded on a JEOL JMX-AX 505 HA mass spectrometer. The NMR spectra of Gal-β-HVA were recorded on a Varian Unity spectrometer at 400 MHz. While the Glc-α-HVA, Glc-β-HVA and Gal-α-HVA were recorded in a Brucker Advance III at 500 Mhz. The experiments realized to each molecule included 1H NMR, 1H-1H COSY, HSQC and HMBC and 100 MHz for 13C NMR using CD3OD as solvent. Chemical shifts are reported in parts per million (ppm) and referenced to the proton resonance of the solvent used for each sample.
Evaluation of radical scavenging activity in glycosides
The diphenyl-picrylhydrazine (DPPH˙) was performed as reported by Yamaguchi et al.25 The reaction mixture containing 2.95 mL of a 100 mM ethanolic DPPH˙ solution and 0.050 mL of the sample dissolved in methanol at an adequate concentration was shaken vigorously on a vortex mixer and incubated at room temperature until no changes in absorbance were observed. Ascorbic acid, a well-known and common free radical scavenger molecule, was used as reference. Unless otherwise stated, for all DPPH˙ EC50 determinations, samples were taken considering that all reactions reached steady state after 90 min. The non-reacted DPPH˙ was determined colorimetrically at 517 nm using a Beckman DU 650 spectrophotometer. Test compounds and changes in the absorbance of the samples were expressed in terms of EC50, indicating the concentration of a compound required for a 50% decrease in the DPPH˙ absorbance. The EC50 parameter was calculated from a concentration–response curve. For kinetic determinations, the decay profile on DPPH˙ absorbance was evaluated during short times (<4 min of reaction) considering an initial DPPH˙ concentration in excess with respect to glycosides in order to analyse the data as a pseudo-first order kinetic model. All analyses were carried out in triplicates. The standard error of the mean (SEM) and EC50 values were obtained with Prism (GraphPad Software, Inc.®, La Jolla, CA, USA).
Statistical analysis
Data of DPPH˙ tests were expressed as mean ± SEM. Scavenging activity was expressed as EC50 value, which denotes the concentration required to give a 50% reduction in scavenging capacity of the radical. The data was compared using one way analysis of variance (ANOVA) followed by the Dunnett comparison test. Values with p < 0.05 were considered significant.
Computational details
Conformers were generated using the Marvin Sketch software (ChemAxon version 6.1) and their geometry-optimized using the MMFF94 force field method.26–30 The search was limited to 20 confomers, with a diversity limit of one and 10
000 seconds of optimization time. The visualization of conformers and measurement of distances and dihedral angles (ωHVA-gly) were performed using the PyMOL software (Schröedinger version 1.74).
For the geometry optimization with density functional theory, the initial geometries were set up analysing different conformers generated previously with a force field MMFF94.26–30 Conformers with the lowest energy were fully optimized. Geometry optimizations and frequency calculations were carried put using the B3LYP31 functional in conjunction with the 6-311G(d) basis set with water as solvent (smd). Local minima were identified by the absence of imaginary frequencies. All electronic calculations were performed with Gaussian 09 program series.32 Two reaction mechanisms were analysed in order to study the free radical scavenger capacity: single electron transfer (SET) and hydrogen atom transfer (HAT). For the SET mechanism it is necessary to calculate the vertical ionization energy (IE) and the vertical electron affinity (EA). In order to compute IE and EA single-point calculations using the optimized structures of the neutral molecule were performed with a different basis set (6-311+G(d)). IE was calculated as the difference between the energy of the cation and the neutral molecule, assuming that both have the ground-state nuclear configuration of the neutral molecule. EA was also calculated as vertical, and represents the energy difference between the neutral and the anion, calculated with the ground-state nuclear configuration of the neutral molecule. For the HAT mechanism, we analysed the bond dissociation energy (D0) of one hydrogen atom. To calculate the D0 values, the hydrogen atoms were dissociated one by one. The corresponding dehydrogenated molecules were fully optimized. Molecular electrostatic potential is the force acting on a positive test charge (a proton) and indicates the molecular charge distribution. It is visualized through mapping its values onto a surface. In this case, a surface with an isovalue of 0.0004 e au−3 was used.
Results and discussion
Synthesis of glycosides and free radical scavenger measurements
In a previous work we reported the enzymatic synthesis of PPGs using vanillyl and homovainillyl β-galactosides as precursors. We found that both PPGs and β-galactosides showed free radical scavenging activity.14 In the course of those syntheses, some important questions arose regarding the effect of the anomeric linkage (α and β anomers) and the sugar nature of the glycosides in this property. We therefore decided to further explore the structure–function relationship based on the already described HVA β-galactoside and extending the enzymatic synthesis to the corresponding α-galactoside, including the α- and β-glucosides of HVA to explore the effect of the chemical nature of the sugar. For this purpose, synthesis reactions employing K. lactis β-galactosidase, green coffee beans α-galactosidase, Toruzyme® CGTase and T. maritima β-glucosidase were performed. The HPLC analyses of these reactions showed yields from low (5% for Gal-α-HVA) to moderate (47% for Gal-β-HVA) on the basis of HVA converted (see ESI†). The products from these reactions were purified and structurally characterized. Under these reaction conditions, high regio-, chemo- and enantioselective glycosylated molecules were obtained, as all products showed 100% anomeric purity, with glycosylation performed exclusively on the primary hydroxyl group of HVA with no alternative glycosylation in the phenolic hydroxyl.
Indeed, HMBC experiments and/or δ 13C confirmed that HVA substrate was linked to sugars through the C-8 carbon atom (–CH2–OH). In one hand, considering the long-range correlations between C-1′ of sugars and the H-8 pair of aglycone or the long-range correlation between C-8 of aglycone and the H-1′ of sugars. On the other hand, the downfield chemical shift of the C-8 carbon in glycosides from around δ 61.77 for the free C-8 of HVA to around δ 68.75–72.05 observed in HVA glycosides (see ESI for details†).
The DPPH˙ assay has been commonly used to evaluate and compare free radical scavenging activity of several isolated molecules or natural extracts. Despite some limitations in the application of this assay,33,34 the assay was here selected due to the stability of the radical, the easiness of application and its generalized adoption.
The free radical scavenger activity of the four synthesized glycosides (Gal-α and -β-HVA, Glc-α and -β-HVA) was evaluated using the radical DPPH˙ and reported as their corresponding EC50 values (EC50-DPPH˙). These results are shown in Table 1, using ascorbic acid as reference. As described, all glycosylated HVAs have lower EC50 values than the corresponding aglycone (HVA). It is clear that glycosylated HVAs are not better free radical scavengers than ascorbic acid, but nevertheless, they are better scavengers than non-glycosylated HVA. In quantitative terms, considering the EC50-DPPH˙ values, HVA glycosides show an increment of the free radical scavenging activity of 12–14% for the α-glycosides and up to 21–22% for the β-glycosides, as compared to the HVA-glycone. Although it may not be surprising to find that glycosylation improves the free radical scavenging capacity of the aglycone, it is interesting to point out that the improvement is higher for the β-glycosides. We may conclude that, under the perspective of the EC50 values, glycosylation alone is not enough to explain the improvement of the free radical scavenging activity of HVA, as the nature of anomeric linkage between the sugar and the aglycone seems to contribute also to this effect.
Table 1 Free radical scavenging activity of HVA-glycosides evaluated by DPPH˙ assay. Activity is reported as the half maximal effective concentration (EC50). Ascorbic acid is included for comparison. The relative increment of the free radical scavenging activity is also reported
Molecule |
EC50-DPPH˙ (μM) |
Relative increment DPPH˙a (%) |
Relative increment of free radical scavenging activity of the EC50 compared to EC50 of HVA aglycone. Data are mean ± SEM. p < 0.1 vs. Glc-β-HVA. |
HVA |
56.10 ± 2.91 |
— |
Glc-α-HVA |
49.64 ± 1.07 |
12 |
Gal-α-HVA |
48.31 ± 0.36 |
14 |
Glc-β-HVA |
44.03 ± 1.11 |
21 |
Gal-β-HVA |
44.47 ± 0.67 |
22 |
Ascorbic acid |
22.60 ± 0.13 |
60 |
Kinetic description of the DPPH˙ measurements
Although the determination of the free radical scavenging activity of chemicals based on EC50 values of the DPPH˙ assay is an extensively applied method in food science and natural product research, the single EC50 value determination is a limited view of the free radical scavenging capacity of the evaluated compound. Actually, as the DPPH˙ assay is nothing but a chemical reaction, it has been suggested that this evaluation should include the reaction kinetic parameters avoiding the comparison made with fixed endpoint measurements.33 In fact, EC50 endpoint assays employing DPPH˙ have recently been strongly criticised as not reliable.34 Foti (2015), emphasized that EC50 is not a kinetic parameter and its purported correlation with the antioxidant properties of chemical substances is not always justified. One of the eventual problems associated with EC50 data, is that for large reaction times the secondary product formed from phenolic antioxidants can also scavenge free radicals. Under this consideration, we evaluated the effect of glycoside concentration in the initial rate of the DPPH˙ radical consumption (VO). For this purpose, the DPPH˙ radical consumption was followed during the first four minutes of reaction, from an initial DPPH˙ concentration in excess with respect to the initial concentration of glycosides, as reported in Fig. 1. From these results, it may be observed that a first order kinetic behaviour is established for the rate dependence for HVA and each HVA glycoside. Considering that this is actually a second order reaction in which the initial DPPH˙ radical concentration was the same for all the reaction assays, and well in excess with respect of the glycoside (AGly) we can state that:
VO = k′′[DPPH˙][AGLy] = k′[AGLy] |
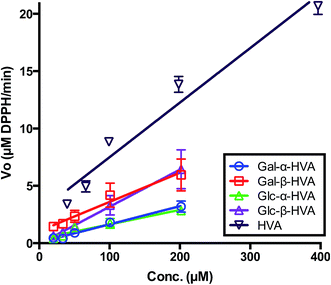 |
| Fig. 1 Initial rates of DPPH˙ free radical scavenging as a function of glycoside concentration (μM). The initial rates were obtained from lineal adjustment on the residual DPPH˙ concentration determined during the initial reaction time. Data are mean ± SEM. | |
The k′ values obtained for the evaluated glycosides, are shown in Fig. 2 and Table 2, where an interesting feature may be observed, supporting the need for kinetic evaluation of the free radical scavenging data instead of a single EC50-DPPH˙ measurement. In effect, contrary to the conclusions driven by the EC50-DPPH˙ data for HVA and its glycosides, the k′ values indicate that HVA, the aglycone, is a faster free radical scavenger of DPPH˙ than its glycosides. Moreover, in terms of the nature of the glycosylation, the kinetic results presented in Fig. 1 and 2, as well as in Table 2, show that independently of the sugar incorporated to the HVA glycoside, the compounds bearing the β anomeric linkage are faster free radical scavengers than those bearing α anomeric linkage. These results confirm the observations drawn from results shown in Table 1, where β glycosides also showed the best free radical scavenging properties.
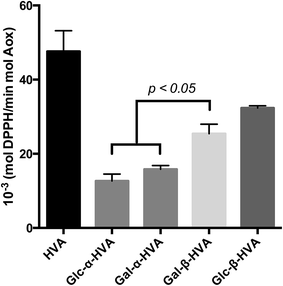 |
| Fig. 2 Pseudo-first order rate constant (k′) of the radical scavenging of DPPH˙ reaction with the corresponding glycosides. Data are mean ± SEM. * = p < 0.05 vs. Glc-β-HVA. ** = p < 0.05 vs. Gal-β-HVA. | |
Table 2 Pseudo first-order rate constant (k′vel) of the radical scavenging of DPPH˙ reaction with the corresponding glycosidea
Molecule |
k′vel (mol DPPH˙ per min mol glycoside) |
Data are mean ± SEM. |
HVA |
47.57 ± 5.6 |
Glc-α-HVA |
12.67 ± 1.84 |
Gal-α-HVA |
15.79 ± 1.04 |
Gal-β-HVA |
25.41 ± 2.58 |
Glc-β-HVA |
32.36 ± 0.57 |
From the results of both EC50 values and the kinetic behaviour of HVA and its glycosides, it is worth mentioning that in one hand, HVA is the best free radical scavenger, as this phenyl alcohol reacts faster with DPPH˙ than all the glycosides evaluated. Conversely, the conclusions derived from the EC50-DPPH˙ determinations suggest that HVA glycosides have a higher free radical scavenger capacity. Certainly, for all cases, lower concentration of glycosides was required to achieve a decrease in 50% of the initial DPPH˙ concentration. From both analysis it seems that glycosidation decrease the reactivity of the aglycone but enhances its long-term reaction stability against free radical scavengers. In agreement with previous reports, it is important to stress the fact that the sole determination of EC50 in the DPPH˙ assay provides limited information concerning the free radical scavenging activity of a given compound, particularly for a comparative purpose. Hence, and as recently reviewed, the evaluation of both, the EC50 and the kinetic parameters allow a better evaluation of the free radical scavenging activity of any given compound.33,34
Up to this point, these results allow us to conclude that HVA glycosylation results in stable long-term free radical scavengers. Moreover, it has been also established that in all the assays the compounds bearing the β anomeric linkage are better free radical scavengers (DPPH˙ assay) than the corresponding α-anomers.
In order to contribute to a better understanding of the structural HVA-glycosides determinants of these enhanced properties, particularly those between the α and β anomers, two structural analysis were applied: a quantum chemistry study in order to define the electrostatic potential surface of the glycosides and to explore how the negatively charged part of the molecules is exposed, and a conformational analysis, in order to establish geometrical differences in the glycosides structure, describing their flexibility and their ability to expose of their negative charge.
Theoretical study
In order to analyse the antiradical capacity of Glc-α-HVA and Glc-β-HVA, Table 3 reports IE, EA, and D0 of both compounds. Experimental information concerning the antiradical capacity is also included. IE and EA are practically the same for both molecules, indicating that the SET mechanism does not explain the experimental results. For the HAT mechanism, the D0 value for Glc-β-HVA is slightly lower than for Glc-α-HVA. This indicating that Glc-β-HVA is better antiradical than Glc-α-HVA, in agreement with the experimental results.
Table 3 Vertical Ionization Energy (IE), Electron Affinities (EA) and dissociation energies (D0) for the molecules under study. Experimental values for the antiradical activity is also included
Molecule |
IE (eV) |
AE (eV) |
D0 (eV) |
EC50 (μM) (DPPH˙) |
Glc-α-HVA |
5.95 |
0.79 |
3.51 |
49.64 |
Glc-β-HVA |
5.97 |
0.79 |
3.46 |
44.03 |
The experiments of the antiradical capacity using the DPPH˙ as free radical are not fully explained with the bond dissociation energy because DPPH˙ is a big molecule and usually free radical scavengers transferred one electron to DPPH˙ and do not transfer and H atom. As explained in the methodology section, the SET mechanism is associated with IE and EA and in this case, these two parameters do not explain the experimental differences between Glc-α-HVA and Glc-β-HVA with the DPPH˙ assay. In summary, the hydrogen bond dissociation energy give us an indication that Glc-β-HVA is a better free radical scavenger than Glc-α-HVA, but does not explain the reaction with DPPH˙.
The main difference between Glc-α-HVA and Glc-β-HVA is the geometry. For this reason, the explanation concerning the differences in reactivity against DPPH˙ should be rather related with the structure and with the molecular electrostatic potential. Since glycosides transferred electrons to DPPH˙, it is important to analyse the region of the molecules that is negatively charged. Electrons would be preferentially transferred from this section of the molecule to the DPPH˙. Fig. 3 presents the molecular electrostatic potentials of Glc-α-HVA (Fig. 3A) and Glc-β-HVA (Fig. 3B). In red is the negatively charged zone and blue indicates the region with less electrons. For Glc-α-HVA, electrons are mainly in the middle of the molecule, whilst for Glc-β-HVA the external part of the molecule is negatively charged. Electrons are more available in Glc-β-HVA than in Glc-α-HVA, and this may explain the differences as electron donors. In summary, Glc-β-HVA may be a better antiradical because the negatively charged part of the molecule is more exposed than in Glc-α-HVA.
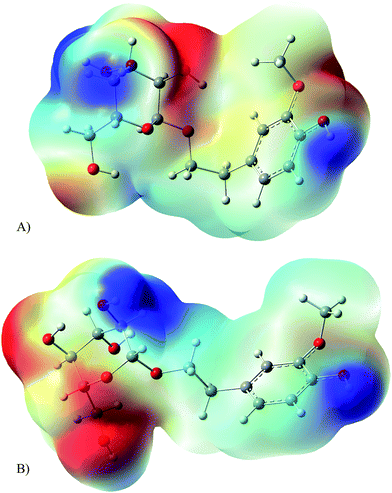 |
| Fig. 3 Molecular electrostatic potential of (A) Glc-α-HVA; (B) Glc-β-HVA. Negative values in red, positive values in blue. | |
Conformational analysis
The theoretical study suggests that the more exposed the negatively charged region of Glc-β-HVA the better its antiradical properties. The ability of a molecule to expose a specific structural region is dependent on its flexibility and some molecular properties depend on this structural feature. Indeed, Castro et al. suggest that the anomeric configuration of alkyl glycosides determines the flexibility and spatial orientation of their alkyl moiety and therefore the three dimensional arrangement of the polar and non-polar moieties of the surfactant.35 In disaccharides it has been reported that this flexibility is dependent on the accessible conformational space of the molecules.36
It is worth mentioning that in terms of free radical scavenging activity assayed against DPPH˙ it has been also reported that the molecular flexibility seems to play a very important role in these properties, where the more flexible molecules showed higher inhibition of the DPPH˙ radical than those with higher rigidity.24
To explore changes on flexibility of HVA glycosides resulting from the accessible conformational space of these molecules, we accomplished a conformational analysis of the α- and β glycosides. Considering that one of the main differences between these compounds is their spatial geometry, we searched for 20 conformers of each glycoside under study using the MMFF94 force field (see Materials and methods section). The aim of the conformational analysis was to explore the effect of rotation of the dihedral angle around the glycosidic linkage on the free radical scavenger properties of the glycosides. Indeed, although structural properties of disaccharides have been well described using ϕ, ψ and Ω dihedral angles around the O–C–O–C arrangement, it seems that the torsion angle should have different parameters when O–C–C–C arrangement is present like in glycosides (blue line in Fig. 5A).
Visual analysis of the generated conformers of HVA glycosides showed different free rotation around HVA. Accordingly, there are structures with a more closed conformation, in which the distant residues may become closer due to the higher flexibility of the molecule (Fig. 4). Actually, in Fig. 4 it may be observed that Glc-β-HVA may adopt a more closed conformation (Fig. 4A; 3.91 Å between the two most distant atoms, shown in red in Fig. 5A) than Glc-α-HVA (Fig. 4B; 6.59 Å between the two most distant atoms, also shown in red in Fig. 5A), suggesting that Glc-β-HVA, is a better antiradical molecule, due to its higher flexibility with respect to Glc-α-HVA.
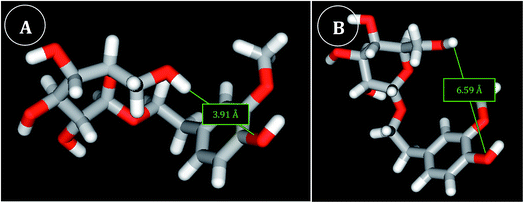 |
| Fig. 4 View of the most closed conformers generated for Glc-β-HVA (A) and Glc-α-HVA (B). The shortest IRD observed for Glc-β-HVA (conformer 3, see ESI†) and Glc-α-HVA (conformer 40, see ESI†) conformers are 3.91 Å and 6.59 Å respectively. | |
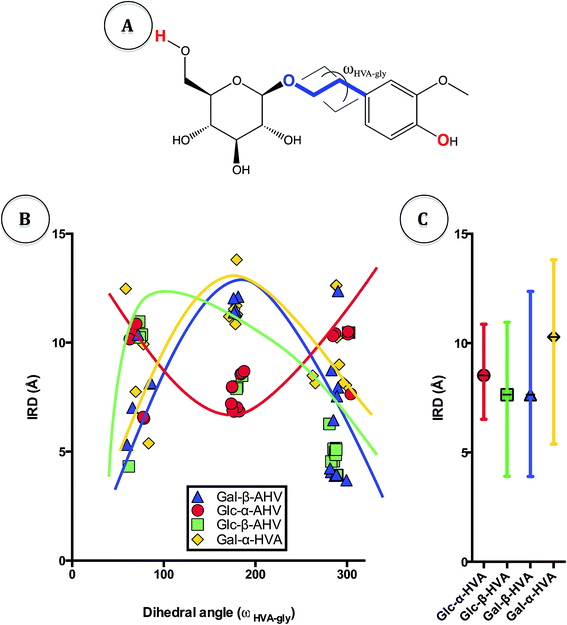 |
| Fig. 5 Relationship between dihedral angle ωHVA-gly and IRD (B), and mean and dispersion of IRD values (C). The IRD values were determined from distances between the two most distant atoms in the planar molecules, atoms in red (A). The dihedral angles (ωHVA-gly) were defined as the angle formed between the more flexible atoms in the structure, blue line in structure (A). For the sake of clarity, tendency lines are included in (B). The molecule shown is Glc-β-HVA (A). | |
In order to visualize the flexibility of conformers as a function of the distances between the more distant atoms in the conformers, we established the Intramolecular Relative Distance (IRD) between the two most distant atoms in the planar molecules (CH2OH oxygen in the sugar molecule and –OH phenolic hydroxyl in HVA, atoms shown in red in Fig. 5A). In addition, we also determined the dihedral angle of glycosides (ωHVA-gly) formed between the more flexible atoms in the structure (blue line in Fig. 5A). For the analysis of relative mobility, the relationship between ωHVA-gly and IRD was defined and is shown in Fig. 5B. It may be observed in this figure that for all Glc-β-HVA and Gal-β-HVA conformers the shortest IRD are found at ωHVA-gly of 80 and 280 degrees. On the contrary, for all Glc-α-HVA and Gal-α-HVA conformers the shortest IRD were observed at ωHVA-gly near to 180 degrees. Nevertheless, in all cases the shortest IRD in the α-glycosides were higher than those observed for β-glycosides. It is interesting to note that Glc-β-HVA and Gal-β-HVA conformers showed a wider dispersion of the mean IRD than the dispersion of the mean IRD for Glc-α-HVA and Gal-α-HVA conformers (Fig. 5C). This indicating that these β-glycosides may adopt a wider conformational dispersion between open and closed conformations. In consequence, β-glycosides showing a higher flexibility than α-glycosides may expose easily their negatively charged groups and therefore exhibit better free radical scavenger properties.
Conclusions
Four different α- and β-glycosides of HVA containing glucose and galactose were enzymatically-synthesized using different glycosidases. Contrary to most chemical methods, this straightforward and highly selective enzymatic method allowed us to glycosylate exclusively the primary hydroxyl of HVA to obtain 100% pure anomers without protection-de-protection strategies. According to the EC50 values obtained in DPPH˙ assay, these enzymatically-synthesized glycosides displayed better free radical scavenger properties than the corresponding HVA-aglycone. Among all glycosides, the β-glycosides exhibited the highest free radical scavenger activity. Thus, these endpoint assays allow us to support the conclusion that glycosyl-residue on glycosides has an important contribution to the free radical scavenging activity of the PPG.
The kinetic study of the DPPH˙ assay demonstrated that the HVA aglycone is more reactive than its glucose or galactose glycosides, two of the most frequently found sugars in natural glycosides. Interestingly, more significant differences in free radical scavenger activity were found based on the nature of the anomeric linkage rather than on the nature of the sugar residue, with β-glycosides displaying better free radical scavenging activity than α-glycosides.
We propose that the increase of the free radical scavenger capacity found for β-glycosides is due to the flexibility of the glycosides and is explained with the aid of the molecular electrostatic potential. This parameter indicates that Glc-β-HVA is a better free radical scavenger than Glc-α-HVA due to the large exposure of the negatively charged region on the β-glycoside molecule. Furthermore, β-glycosides show higher flexibility than α-glycosides exposing advantageously their negatively charged groups, something that favours the free radical scavenger capacity. These results are very good examples of the correlation between the structure, the molecular charge distribution and the free radical scavenger capacity.
Acknowledgements
This research was supported by PAPIIT-UNAM (grant IN209016) and CONACyT (grant 219728). J.A. González-Ríos, Y. Romero and M. Reina thank CONACyT for scholarships 313184, 332509, 3077279 respectively. Authors thank Fernando Gonzalez for technical assistance and Novozymes-Mexico for their generous donation of CGTase. We thank DGTIC-UNAM for the assistance. Mitztli supercomputer of DGTIC to do all the quantum chemical calculations.
References
- V. Puchart, Glycoside phosphorylases: structure, catalytic properties and biotechnological potential, Biotechnol. Adv., 2015, 33(2), 261–276 CrossRef CAS PubMed.
- M. De Roode, M. Franssen, A. van der Padt and R. Boom, Perspectives for the industrial enzymatic production of glycosides, Biotechnol. Prog., 2003, 19, 1391–1402 CrossRef PubMed.
- G. H. Meulenbeld and S. Hartmans, Transglycosylation by Streptococus mutans GS-5 glucosyltransferase-D: acceptor specificity and engineering of reaction conditions, Biotechnol. Bioeng., 2000, 70, 363–369 CrossRef CAS PubMed.
- F. van Rantwijk, M. W. V. Oosterom and R. A. Sheldon, Glycosidase-catalysed synthesis of alkyl glycosides, J. Mol. Catal. B: Enzym., 1999, 6, 511–532 CrossRef CAS.
- T. Desmet, W. Soetaert, P. Bojarová, V. Křen, L. Dijkhuizen, V. Eastwick-Field and A. Schiller, Enzymatic glycosylation of small molecules: challenging substrates require tailored catalysts, Chem.–Eur. J., 2012, 18(35), 10786–10801 CrossRef CAS PubMed.
- G. Marini, G. Forno, R. Kratje and M. Etcheverrigaray, Recombinant human granulocyte-macrophage colony-stimulating factor: effect of glycosylation on pharmacokinetic parameters, Electron. J. Biotechnol., 2007, 10, 272–278 Search PubMed.
- B. Mishra, K. I. Priyadarsini, M. S. Kumar, M. K. Unnikrishnan and H. Mohan, Effect of o-glycosylation on the antioxidant activity and free radical reactions of a plant flavonoid, chrysoeriol, Bioorg. Med. Chem., 2003, 11, 2677–2685 CrossRef CAS PubMed.
- J. Vaya and M. Aviram, Nutritional antioxidants mechanisms of action, analyses of activities and medical applications, Curr. Med. Chem.: Immunol., Endocr. Metab. Agents, 2001, 1, 99–117 CrossRef CAS.
- F. E. Vaistij, E. K. Lim, R. Edwards and D. J. Bowles, Glycosylation of secondary metabolites and xenobiotics, in Plant-derived natural products, Springer, USA, 2009, pp. 209–228 Search PubMed.
- K. Robards, P. Prenzler, G. Tucker, P. Swatsitang and W. Glover, Phenolic compounds and their role in oxidative processes in fruits, Food Chem., 1999, 66(4), 401–436 CrossRef CAS.
- C. Rice-Evans, N. Miller and G. Paganga, Structure-antioxidant activity relationships of flavonoids and phenolic acids, Free Radical Biol. Med., 1996, 20(7), 933–956 CrossRef CAS PubMed.
- M. Soobrattee, V. Neergheen, A. Luximon-Ramma, O. Aruoma and T. Bahorun, Phenolics as potential antioxidant therapeutic agents: Mechanism and actions, Mutat. Res., Fundam. Mol. Mech. Mutagen., 2005, 579, 200–213 CrossRef CAS PubMed.
- T. Vogt, Phenylpropanoid Biosynthesis, Mol. Plant, 2009, 3(1), 2–20 CrossRef PubMed.
- A. López-Munguía, Y. Hernández-Romero, J. Pedraza-Chaverri, A. Miranda-Molina, I. Regla, A. Martínez and E. Castillo, Phenylpropanoid glycoside analogues: enzymatic synthesis, antioxidant activity and theoretical study of their free radical scavenger mechanism, PLoS One, 2011, 6(6), 1–9 Search PubMed.
- S. Stoyanova, J. Geuns, É. Hideg and W. Ende, The food additives inulin and stevioside counteract oxidative stress, Int. J. Food Sci. Nutr., 2011, 62(3), 207–214 CrossRef CAS PubMed.
- A. J. Day, M. S. DuPont, S. Ridley, M. Rhodes, M. J. Rhodes, M. R. Morgan and G. Williamson, Deglycosylation of flavonoid and isoflavonoid glycosides by human small intestine and liver β-glucosidase activity, FEBS Lett., 1998, 436(1), 71–75 CrossRef CAS PubMed.
- G. Gattuso, C. Caristi, C. Gargiulli, E. Bellocco, G. Toscano and U. Leuzzi, Flavonoid glycosides in bergamot juice (Citrus bergamia Risso), J. Agric. Food Chem., 2006, 54(11), 3929–3935 CrossRef CAS PubMed.
- H. Tapiero, K. D. Tew, G. N. Ba and G. Mathe, Polyphenols: do they play a role in the
prevention of human pathologies?, Biomed. Pharmacother., 2002, 56(4), 200–207 CrossRef CAS.
- A. AlQathama and J. M. Prieto, Natural products with therapeutic potential in melanoma metastasis, Nat. Prod. Rep., 2015, 32, 1170–1182 RSC.
- K. D. Setchell, N. M. Brown, P. Desai, L. Zimmer-Nechemias, B. E. Wolfe, W. T. Brashear and J. E. Heubi, Bioavailability of pure isoflavones in healthy humans and analysis of commercial soy isoflavone supplements, J. Nutr., 2001, 131(4), 1362–1375 Search PubMed.
- R. A. Walgren, K. J. Karnaky, G. E. Lindenmayer and T. Walle, Efflux of dietary flavonoid quercetin 4′-β-glucoside across human intestinal Caco-2 cell monolayers by apical multidrug resistance-associated protein-2, J. Pharmacol. Exp. Ther., 2000, 294(3), 830–836 CAS.
- A. D. French, Comparisons of rigid and relaxed conformational maps for cellobiose and maltose, Carbohydr. Res., 1989, 188, 206–211 CrossRef CAS.
- C. A. Stortz, G. P. Johnson, A. D. French and G. I. Csonka, Comparison of different force fields for the study of disaccharides, Carbohydr. Res., 2009, 344(16), 2217–2228 CrossRef CAS PubMed.
- A. Sánchez, O. Martínez-Mora, E. Martínez-Benavidez, J. Hernández, Z. Domínguez and M. Salas-Reyes, Electrochemical behaviour of new dimeric esters and amides derived from caffeic acid in dimethylsulfoxide, Org. Biomol. Chem., 2014, 12(31), 5981–5989 Search PubMed.
- T. Yamaguchi, H. Takamura, T. Matoba and J. Terao, HPLC method for evaluation of the free radical-scavenging activity of foods by using 1,1-diphenyl-2-picrylhydrazyl, Biosci., Biotechnol., Biochem., 1998, 62, 1201–1204 CrossRef CAS PubMed.
- T. A. Halgren, Merck Molecular Force Field. I. Basis, form, scope, parameterization, and performance of MMFF94, J. Comput. Chem., 1996, 17, 490–519 CrossRef CAS.
- T. A. Halgren, Merck Molecular Force Field. II. MMFF94 van der Waals and electrostatic parameters for intermolecular interactions, J. Comput. Chem., 1996, 17, 520–552 CrossRef CAS.
- T. A. Halgren, Merck Molecular Force Field. III. Molecular geometries and vibrational frequencies for MMFF94, J. Comput. Chem., 1996, 17, 553–586 CrossRef CAS.
- T. A. Halgren and R. B. Nachbar, Merck Molecular Force Field. IV. Conformational energies and geometries for MMFF94, J. Comput. Chem., 1996, 17, 587–615 CAS.
- T. A. Halgren, Merck Molecular Force Field. V. Extension of MMFF94 using experimental data, additional computational data, and empirical rules, J. Comput. Chem., 1996, 17, 616–641 CrossRef CAS.
- A. D. Becke, Density-functional exchange-energy approximation with correct asymptotic behaviour, Phys. Rev. A, 1988, 38, 3098–3100 CrossRef CAS.
- M. J. Frisch, et al., Gaussian 09, Revision A.08, Gaussian, Inc., Wallingford CT, 2009 Search PubMed.
- R. Amorati and L. Valgimigli, Advantages and limitations of common testing methods for antioxidants, Free Radical Res., 2015, 49(5), 633–649 CrossRef CAS PubMed.
- M. C. Foti, Use and Abuse of the DPPH˙ Radical, J. Agric. Food Chem., 2015, 63(40), 8765–8776 CrossRef CAS PubMed.
- M. J. Castro, J. Kovensky and A. F. Cirelli, Synthesis of new dimeric amphiphiles. Influence of anomeric configuration and spacer functionality on interfacial properties, J. Carbohydr. Chem., 2000, 19(9), 1175–1184 CrossRef CAS.
- R. B. Best, G. E. Jackson and K. J. Naidoo, Molecular dynamics and NMR study of the α(1→4) and α(1→6) glycosidic linkages: maltose and isomaltose, J. Phys. Chem. B, 2001, 105(20), 4742–4751 CrossRef CAS.
Footnote |
† Electronic supplementary information (ESI) available. See DOI: 10.1039/c6ra06572d |
|
This journal is © The Royal Society of Chemistry 2016 |