DOI:
10.1039/C6RA04293G
(Paper)
RSC Adv., 2016,
6, 29072-29079
Enhanced bioelectrochemical reduction of p-nitrophenols in the cathode of self-driven microbial fuel cells
Received
17th February 2016
, Accepted 9th March 2016
First published on 11th March 2016
Abstract
Reduction from p-nitrophenol (PNP) to p-aminophenol (PAP) was studied in the cathodes of self-driven microbial fuel cells (MFCs), and the influence of electron donor (CH3COONa) & acceptor concentration, external resistance and electrolyte conductivity were investigated. The results showed that PNP reduction efficiencies reached 100% when initial concentrations were lower than 50 mg L−1. PAP formation efficiencies were promoted from 31.7 ± 2.1% to 76.4 ± 4.1%, by increasing CH3COONa concentration from 2000 mg L−1 to 4000 mg L−1. Decreasing the external resistance from 1000 Ω to 240 Ω improved the PAP formation by 4–5 times for higher currents. Increasing the electrolyte conductivity (controlled by total dissolved solids, TDS) could accelerate the PNP degradation process within 24 h. Illumina high-throughput sequencing showed the dominating bacteria on the biocathodes were: Ignavibacterium 14.37%, Ottowia 4.04%, Pseudomonas 3.66%, Proteiniphilum 2.50%, Chlorobaculum 1.67%, Nitrospira 1.62% (at the genera level). The bacteria might play vital roles in more efficient nitrophenol degradation in biocathode MFCs. Compared to the abiotic control, the main advantages of PNP degradation in biocathodes are higher PNP reduction & PAP formation efficiencies, less electron donor consumption and higher system efficiency. The self-driven MFC system could be a promising way to deal with nitroaromatic pollutants.
1. Introduction
Nitrophenol is a typical toxic and refractory nitroaromatic pollutant. It widely exists in wastewater from pesticide factories, coking plants, paper mills, and printing & dyeing mills.1 Nitrophenols hold risks to human health and the environment for their bio-accumulating, mutagenic and carcinogenic effects.2 Conventional chemical methods (such as advanced oxidation and chemical reduction) used to remove nitroaromatic compounds are usually inefficient, expensive and most importantly, non-sustainable.3 Meanwhile, as xenobiotics, nitrophenols are difficult to be degraded with conventional biological process. Special acclimations of the microbes or genetically engineered bacteria are often needed, and the treating process is usually not easy to control.3
With the recent development of bioelectrochemical technology, it is considered to be a promising strategy to degrade nitroaromatic compounds.4 Bioelectrochemical methods have the advantages of renewable, sustainable and cost-effective. There are two types of operation modes of bioelectrochemical system: Microbial Electrolysis Cell (MEC) and Microbial Fuel Cell (MFC). In the cathode of a bioelectrochemical system, nitrophenols are transformed to APs, which are less toxic and easier to be mineralized. Afterwards, APs are hopeful to be further degraded in the anode.5 Several studies were conducted in MEC systems: Mu et al. removed nitrobenzene in a MEC system with bio-anode and chemical cathode.6 Wang et al. introduced effective nitrobenzene reduction to aniline in a MEC system with bio-anode and bio-cathode, and found less intermediate products during the reduction process.7 Besides, Sun et al. demonstrated in situ degradation of nitrobenzene with sequential reduction/oxidation, and determined the effect of applied voltage and initial concentrations.8 However, the above studies need energy input to the bioelectrochemical system to guarantee the reduction efficiency, and this may lead to certain amount of financial cost in future application. Li et al. assessed nitrobenzene reduction in a ferricyanide – cathode catalyzed by Pt in a MFC.9 The ferricyanide – cathode is also not a long-lasting way for the need to replace the cathode after a period of operation. Continuous cost is an inevitable problem in such systems. Thus much effort is still required to develop more economic methods for treatment of nitroaromatic compounds.
MFC equipped with bioanode and biocathode is a self-driven style of bioelectrochemical system. Reactions in the system are totally catalyzed by the microbes on the electrodes, instead of driven by applied voltage as that in MECs. No energy input is needed, and the biotic electrodes are self-renewable with the re-generation of the microbes. It is a low-cost strategy to degrade pollutants in this style. In this work, p-nitrophenol (PNP), a representative of nitrophenols, was selected as the target compound to study its enhanced reduction in a self-driven system. PNP were reduced in a MFC system with bio-catalyzed cathode, and not any energy input was applied. The key factors determining the reduction current (electron donor, electron acceptor and external resistance) were studied. The bacterial morphology and community composition on the cathode were explored to investigate the function of the microbes in the reduction process.
2. Materials and methods
2.1 MFC reactors and electrode materials
Double-chambered MFCs operated in parallel were used in the study. The rectangular MFC reactors were fabricated of poly-acrylic plastic. The working volumes of both the anode and cathode chambers of the MFCs were 150 mL. Plain carbon cloth (NOK H2315 T10A, Japan) was used as the anode and cathode electrode material. The electrodes were washed in 1 mol L−1 HCl and 1 mol L−1 NaOH to remove contaminants. The anode and the cathode were connected externally through titanium wire. Proton exchange membrane (Nafion117, USA) was used to separate the anode and cathode chamber. The membranes were pretreated as described by Liu and Logan,10 and placed between the anode and cathode frames. External resistances (1000 Ω for startup, 240 Ω for operation) were used to close the circuit. Surface areas of the anodes, cathodes, and proton exchange membrane (PEM) were 50 cm2.
2.2 Reactor inoculation and operation
Anaerobic sewage sludge from a pesticide manufacturing plant was used as the inocula for anodes of the MFCs. The cathodes were inoculated with the effluent of a anaerobic reactor treating nitrophenols. Composition of the electrolyte in both anode and cathode chambers was: NH4Cl 310 mg L−1, KCl 130 mg L−1, NaH2PO4·2H2O 5.6 g L−1, Na2HPO4·12H2O 6.07 g L−1, with trace elements added.10 Sodium acetate was used as the electron donor and carbon source in the anode chambers. PNPs in the cathode chamber were the electron acceptor, and NaHCO3 was used as the inorganic carbon source for microbes in the cathode. The MFC reactors were operated in batch mode. Electrolyte and substrate in the MFCs were replaced at the end of an electricity generation cycle (when the voltage output dropped to 50 mV with an external resistance of 1000 Ω). Conductivity of the electrolyte was controlled by adjusting the total dissolved solid (TDS) with KCl in both the anodic and cathodic chambers. The reactors were operated at a controlled temperature of 30 °C in a water bath.
2.3 Electrical and chemical analysis
Voltage (V) across the external resistance (RE) was measured using a multimeter (Victor, VC9804A+, China). COD was measured according to the standard method. Samples taken from the reactors were immediately filtered through a 0.22 μm filter. Sodium acetate in the anode chamber were monitored by determining the CH3COO− with ion chromatography (DIONEX ICS-2100, USA). PNP and PAP were measured using high performance liquid chromatography (HPLC) (SHIMADZU LC-20AT, Japan) equipped with RP18 column (5 μm, 4.6 × 250 mm). The mobile phase was 40% methanol and 60% H2O pumped at 1 mL min−1. The detection was performed at 254 nm. pH and temperature were monitored with a pH meter (HANNA pH211, Italy).
2.4 Electrochemical analysis
Analysis of cyclic voltammetry (CV) was performed using electrochemical workstation (BIO-LOGIC, VMP3, France), equipped with three-electrode system. The cathode and anode of the MFCs were used as the working and counter electrode, respectively. The saturated calomel electrode inserted in the cathode chamber was used as the reference electrode. The potential range was set from −0.8 to +0.5 V, and the scanning rate was 10 mV s−1.
2.5 Scanning electron microscopy (SEM)
Samples of electrodes were cut from the reactor with sterile knife, and fixed in 4% glutaraldehyde, then rinsed with 0.1 mol L−1 PBS. Afterwards, they were postfixed overnight in 1% osmium tetroxide, then rinsed with 0.1 mol L−1 PBS, and dehydrated by a graded ethanol series (30, 50, 70, 90, and 100%), before exchanging in isoamyl acetate. Samples were CO2-critical point dried in cpd-2 (HITACHI, Japan), mounted on aluminum specimen mounts with contact adhesive, and conducting bridges were applied with isopropanol-based colloidal graphite paint. Then samples were sputter coated in an IB-5 Sputter Coater (Eiko-Engineering, Japan) using a gold–palladium target and observed in an scanning electron microscope (FEI Quanta 250, Netherlands). The SEM was operated at 20 kV, and images were captured digitally.
2.6 Microbial community analysis
Pieces of bio-cathode were cut off using with sterile knife to analyze the microbial community. After mixed with sterile saline solution, the treated sample was placed in sterile containers and vortexed for 1 min. The suspended biofilm was collected by centrifugation at 3000g for 10 min. Biofilms for 16S rRNA gene sequencing amplicon were processed as follows: DNA extraction with FastDNA™ Spin Kit for soil (MP Biomedicals, Santa Ana, CA), 16S rRNA gene PCR amplification and PCR products purification. To amplify and sequence the V1V2 hypervariable region of the 16S rRNA gene, forward primer (5′-AGAGTTTGATYMTGGCTCAG-3′) and reverse primer (5′-TGCTGCCTCCCGTAGGAGT-3′) were selected and different 8-bases barcodes and a guanine were linked to the 5′ end of each primer. Then the purified products were sent for sequencing using Illumina Miseq sequencing platform (Illumina Inc., USA) at Jiangsu Zhongyijinda Analytical and testing limited company (Jiangsu, China). The acquired data was processed by Sickle and Mothur program to remove the low quality sequences and reduce noises (https://www.github.com/najoshi/sickle). The 16S rRNA gene sequences were classified into operational taxonomic units (OTUs) at 3% cutoff (or 97% similarity). The filtered sequences were assigned to a taxon by the stand-alone RDP classifier (http://www.sourceforge.net/projects/rdp-classifier/).11,12 Sequences of the sample were submitted to NCBI SRA (Accession no. SRR 2489405, SRX1275672, SRS1081676).
3. Results and discussion
3.1 Voltage outputs with PNP reduction
During the startup period, the PNP in the cathodes were degraded completely in both the biotic and abiotic cathode reactors, but no PAP was detected. The reactor with 100 mg L−1 PNP in the cathode showed fluctuating voltage outputs, while that in the reactor with 50 mg L−1 PNP in the cathode was stable. When the voltage outputs of consecutive two cycles were the same, the stable operation period began. The voltage outputs (61 ± 5 mV, 240 Ω) of the bio-cathode reactors were lower in the operation period than in the end of the startup period (124 ± 11 mV, 240 Ω). After operation for 5 months, the voltage outputs of the bio-cathode reactors decreased gradually. The time-course decreasing of the voltages may be attributed to more PAP formation. PAP may be oxidized to organic compound which can be utilized by heterotrophs. Increasing PAP in the cathode chamber might suppress the growth of the autotrophs on the cathode. The autotrophs on the cathodes are related to electricity generation of the reactors.13 Therefore, the higher PAP formation led to the lower power outputs of the reactors. Besides, owing to the biocatalysis on both anode and cathode, the bioelectrochemical reduction of PNP and the formation of PAP in the bio-cathode reactor were faster than in the abiotic cathode reactor during the whole process.
3.2 Effect of initial PNP concentration on cathode reduction
PNPs act as the electron acceptor which received the electrons from the anode, and were reduced to PAPs in the cathode. Initial concentrations of PNP in the cathode were adjusted at 25 mg L−1, 50 mg L−1, and 100 mg L−1, to study the effect of initial PNP concentration on reduction efficiency (Fig. 1a and b). The concentrations of CH3COONa in the anode were 3000 mg L−1.
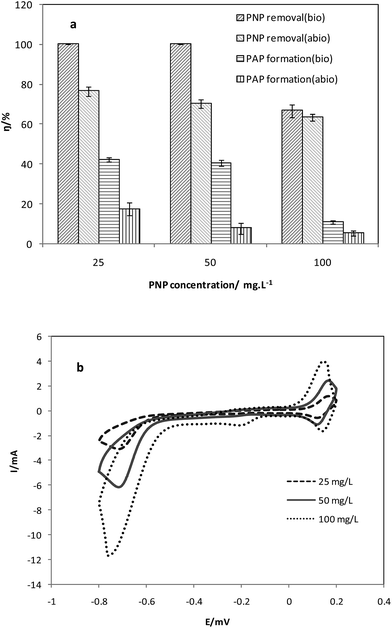 |
| Fig. 1 (a) PNP degradation and (b) CV curves at different initial concentrations (25, 50, 100 mg L−1). | |
Within 50 h, the reduction efficiencies of PNPs in the biotic cathode were 100%, 100%, and 66.7 ± 3.1% with the initial concentrations of 25 mg L−1, 50 mg L−1, and 100 mg L−1, respectively. In the abiotic cathode controls, the corresponding reduction efficiencies were 76.5 ± 2.3%, 70.2 ± 2.0%, and 63.4 ± 1.7%. Bacteria on the biotic cathode improved the PNP reduction efficiencies at all concentration levels. Lower reduction efficiency of PNPs at 100 mg L−1 might be attributed to competition with the adsorption process of the electrode.8
As to the AP formation efficiencies, 42.3 ± 1.1%, 40.6 ± 1.6%, and 10.9 ± 0.9% were achieved at initial PNP concentrations of 25 mg L−1, 50 mg L−1, and 100 mg L−1, respectively. Meanwhile, PAP formation efficiencies were 17.5 ± 3.3%, 7.8 ± 2.9% and 5.4 ± 1.3% in the abiotic controls. The significant improvements of AP formation in bio-cathodes were induced by better electron transfer aided by bacteria on the cathode. Besides, there might be less intermediate products in the bio-cathode.7
CH3COONa which was oxidized in the anode chamber served as the electron donor in the whole system. In the biotic cathode reactors, 29.5 ± 1.2%, 43.2 ± 2.1%, and 57.3 ± 1.5% of the added CH3COONa were consumed with initial PNP concentrations of 25 mg L−1, 50 mg L−1, and 100 mg L−1, respectively. However, in the abiotic cathode reactors, the added CH3COONa were consumed completely at all the three initial PNP concentration conditions. Therefore, the electron donors were utilized more efficiently in the biotic cathode reactors as a result of the microbial catalysis.
As is shown in the CV curve (Fig. 1b), with the increase of PNP concentration from 25 mg L−1 to 100 mg L−1, the peak of reduction current on the curve became stronger. PNP was the driving force of the reduction process in the cathode chamber. The result proved that the reduction process was enhanced by increasing PNP concentrations.
3.3 Effect of initial CH3COONa concentration on PNP reduction
CH3COONa served as an easily-utilized carbon source for the microbes on the anode of the MFC system. At the same time, CH3COONa in the anode was the electron donor for PNP reduction in the system. The electrons produced from CH3COONa oxidation get the cathode through the external circuit, and then were consumed for PNP reduction. Initial CH3COONa concentrations in the anode chamber were set as 2000 mg L−1, 3000 mg L−1, and 4000 mg L−1, to study the influence of electron donor on the cathodic reduction process (Fig. 2). The concentrations of PNP in the cathode chamber were 50 mg L−1 in the test.
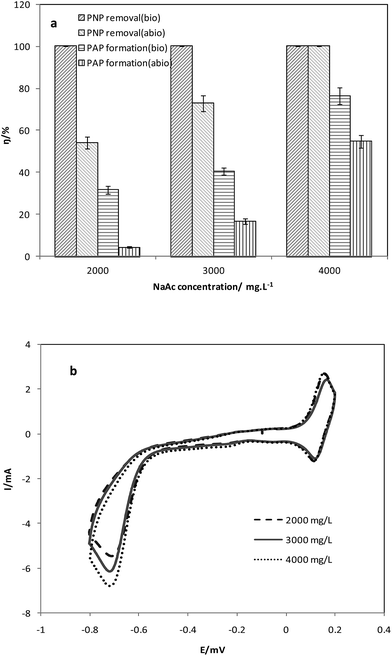 |
| Fig. 2 (a) PNP degradation and (b) CV curves at different electron donor concentrations (2000, 3000, 4000 mg L−1). | |
The reduction efficiencies of PNP in the biotic cathode achieved 100% at all the three initial CH3COONa concentrations. The time for PNP reduction was shortened from 80 h (2000 mg L−1 CH3COONa) to less than 50 h (3000 mg L−1 and 4000 mg L−1 CH3COONa) with the increase of CH3COONa concentrations. The increase of electron donors in the anode accelerated the reduction process of PNPs in the biotic cathodes. In contrast, the reduction efficiencies of PNP in the abiotic cathode were 54.1 ± 2.9%, 72.9 ± 3.8%, and 100% at CH3COONa concentrations of 2000 mg L−1, 3000 mg L−1, and 4000 mg L−1. The reduction rates of PNP were also promoted by increasing electron donors. Higher concentrations of CH3COONa could provide more electrons. More electrons get the cathode enhanced the driving force for reduction process. As a result, the PNP reduction was improved.
In the abiotic cathode control, the PAP formation efficiencies were 4.3 ± 0.4%, 16.7 ± 1.3%, and 54.8 ± 3.1% with CH3COONa concentrations at 2000 mg L−1, 3000 mg L−1, and 4000 mg L−1. In the biotic cathode reactors, the PAP formation efficiencies increased notably to 31.7 ± 2.1%, 40.6 ± 1.6%, and 76.4 ± 4.1%, respectively. The higher PAP formation in the biocathode could be attributed to two reasons: firstly, microbes on the biocathode overcame some of the overpotential on the cathode and resulted in less electron loss; secondly, microbial catalysis effect on the biocathode decreased the intermediate compounds during the PNP reduction. The cathodic potential was −350 mV (vs. Ag/AgCl electrode) in the biotic cathode, which was more positive than −450 mV (vs. Ag/AgCl electrode) in the abiotic cathode. The result is similar to that of Wang et al.7 It led to higher voltage output in biotic cathode reactors.
Under different concentrations added, the CH3COONa in the anodes was completely consumed in the abiotic cathode reactors. However, the highest consumption of CH3COO− in the biotic cathode reactors was 63.7 ± 4.5%, which much lower than that in the abiotic ones. It also implied that the loss of electrons in the system was reduced on the biocathode. Thus the electron donors were more efficiently used in the biotic cathode reactors.
As is shown in the CV curve (Fig. 2b), with the increase of CH3COONa concentration from 2000 mg L−1 to 4000 mg L−1, the higher peak of oxidation current was achieved. The result induced the promoting effect of increasing electron donors on the electrochemical reactions in the system. Thus the PNP reduction process was improved with higher CH3COONa concentrations.
3.4 Effect of external resistance and electrolyte conductivity on system performance
There are two charge flows in bioelectrochemical systems: one is the electron flow from anode to cathode through the external circuit, the other is the ion flow to equalize the electron flow in the chambers. In the MFC system, external resistance influences the electron flow, and conductivity of the electrolyte affects the ion flow. Therefore, the electricity generation and PNP removal was studied at different external resistance and total dissolved solids (TDS) levels.
External resistance is a major factor that decides the reduction current in the MFC system. Different external resistances were tested to determine if the PNP reduction efficiency was dependent on external resistance (Fig. 3). The bioelectrochemical reactors were operated at external resistance of 1000 Ω, 500 Ω and 240 Ω, and the according currents through the wire were 0.21 mA, 0.32 mA, and 0.56 mA, respectively. Over 80 h, 90.7 ± 3.2% of the PNPs were removed in the 1000 Ω reactor. However, in the reactors with external resistances of 500 Ω and 240 Ω, the PNPs were removed completely within 30 h and 50 h, respectively. The reduction rate of PNP was improved with decreasing external resistances, partly as a result of higher current density.14 The longer reduction time at 240 Ω might be attributed to ohmic loss at relatively higher currents in the reactor system.8 The maximum efficiencies of PAP formation were 7.5 ± 1.8%, 21.4 ± 2.1% and 32.5 ± 2.6% at external resistance of 1000 Ω, 500 Ω and 240 Ω. The results revealed that decreasing the external resistance to some extent could improve the system efficiency.
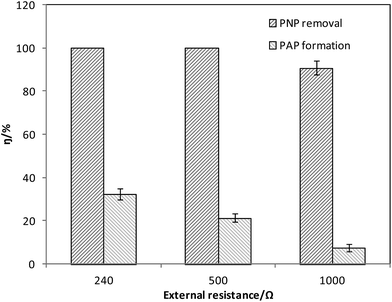 |
| Fig. 3 PNP degradation at different external resistance (240, 500, 1000 Ω). | |
TDS of the electrolyte represents conductivity. The biotic and abiotic cathode MFCs were operated at TDS of 5 g L−1, 10 g L−1, 20 g L−1, and 30 g L−1. The highest current in the circuit of the biotic cathode reactors was 0.27 mA at TDS 20 g L−1. Increasing conductivity did not consecutively improve the electricity generation in the bio-cathode reactors. It implied that TDS 30 g L−1 might exceed the salt tolerance level of the electricity-generating bacteria in the system. Cell wall damage and metabolic repression of the microbes would be induced at higher TDS.15 The PNP removal efficiencies at different TDSs were showed in Fig. 4a and b. More than 94.4 ± 8.0% of PNPs in the bio-cathode were removed within 96 h at all the TDS levels. However, the highest PNP removal efficiency was 85.3 ± 6.3% at TDS 30 g L−1 in the abiotic cathode controls. Thus the microbes on the bio-cathode were able to improve the PNP reduction process at different TDSs. Besides, in the bio-cathode reactors, higher TDS accelerated the PNP removal notably within 24 h. The PNP removal efficiency increased from 48.4 ± 3.7% at TDS 5 g L−1, to 69.3 ± 5.2% at TDS 30 g L−1. However, the improvement was not significant when the residual PNP concentration was lower than 10 mg L−1. Therefore, the promoting effect from increasing TDS is relevant to PNP concentration levels.
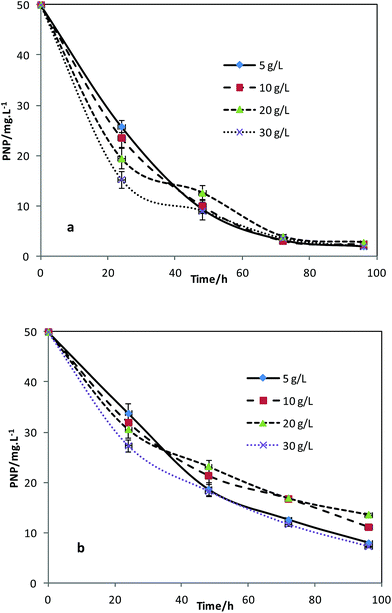 |
| Fig. 4 PNP degradation in (a) biotic and (b) abiotic cathodes at different salt concentrations (TDS 5, 10, 20, 30 g L−1). | |
3.5 Surface morphology of the cathodes
Surface morphology of the cathodes was observed with Scanning Electron Microscope (SEM) at the magnification of 500× and 1500×. Fig. 5 is the SEM images of the cathodic carbon cloth in the biotic (a and c) and abiotic (b and d) cathodes. It showed that surface of the carbon fibers in the abiotic cathodes was clean, and the fibers were arranged in order. While in the biotic cathodes, the carbon fibers were covered scatteredly or clusteredly with biofilms, and the formerly ordered arrangement was disturbed to some extent by the cathodic biofilm. The result was similar to previous studies on biocathodes.16,17
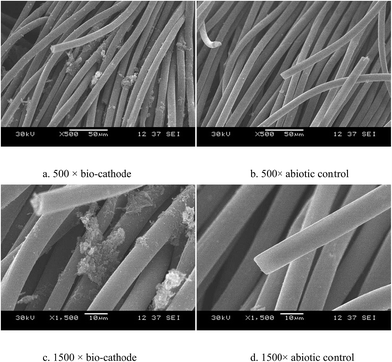 |
| Fig. 5 Surface morphology of the biotic (a and c) and abiotic (b and d) cathodes. | |
The difference between the biotic and abiotic cathodes proved the successful formation of biofilm on the biocathode. As a result, the microbes in the biofilm were fixed onto the biocathodes and could play vital roles in more efficient reduction of nitrophenols. Details of the function bacteria was discussed in Section 3.6.
3.6 Microbial community analysis on the bio-cathode
The species diversity indices (3% cutoff) of bacteria sample from the bio-cathode are listed in Table 1. Microbial communities on the bio-cathode were analyzed with Illumina Mi-seq High-throughput sequencing. At the phylum level, the most abundant bacteria on the cathodes were: Proteobacteria 31.32%, Chlorobi 30.53%, Bacteroidetes 14.87%, Chloroflexi 5.05%, Actinobacteria 2.96%, Nitrospira 1.62%, Gemmatimonadetes 1.04%, Firmicutes 0.73% (Fig. 6a). The major communities at the genera level were: Ignavibacterium 14.37%, Ottowia 4.04%, Pseudomonas 3.66%, Proteiniphilum 2.50%, Chlorobaculum 1.67%, Nitrospira 1.62%, Azospirillum 1.49%, Geobacter 1.37% (Fig. 6b).
Table 1 Species diversity indices (3% cutoff) of bacteria on bio-cathode
OTUs |
Chao1 |
Shannon |
ACE |
Simpson |
Goods coverage |
1319 |
3152 |
4.01 |
5199 |
0.0736 |
0.982 |
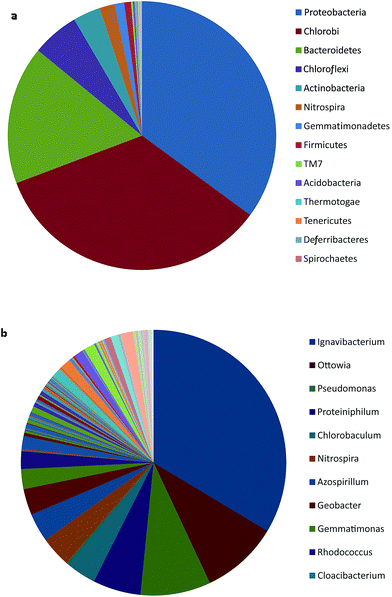 |
| Fig. 6 Microbial community composition on the bio-cathode ((a) at the phylum level, (b) at the genera level). | |
Ignavibacterium is a facultatively anaerobe from the phylum Chlorobi (Liu et al., 2012). It had been frequently found in anammox reactors and could utilize nitrite as the electron acceptor.18 As the major genera on the biocathode, Ignavibacterium may lead the reduction process and played a key role in reducing intermediates in the cathodic degradation. Ottowia (belonging to Comamonadaceae) was reported to be a facultatively anaerobic bacteria which could take nitrate and nitrite as electron acceptors.19,20 It was often found in coking wastewater activated sludge and had phenol-degrading ability.21 Ottowia could participate in the transition from PNP to PAP, and related phenol degrading process in this study. Pseudomonas (belonging to γ-Proteobacteria) was reported to degrade a wide range of phenol compounds and facilitate the electricity generation in MFC by producing mediators.22 Cometabolic biodegradations of phenol and 4-chlorophenol by Pseudomonas were studied by researchers.23,24 Thus the multi-function bacteria could function in both the electricity generation and bioelectrochemical reduction.25 Proteiniphilum was reported to be a proteolytic anaerobic bacteria, and was once isolated from the granule sludge of an upflow anaerobic sludge blanket reactor treating brewery wastewater.26 It was also used for bioaugmentation of sewage sludge to improve the removal of polycyclic aromatic hydrocarbons.27 Its existence might promote the degradation process in the biocathode. Nitrospira was a nitrifying bacteria which had the high affinity for nitrite as electron donor.28 It usually prevails in sewage treatment system with micro anoxic environment.29 Therefore its function here in this system may be the reduction of nitro-groups of nitrophenols. Azospirillum is a rhizosphere-dwelling, N2-fixing bacterium. It is versatile in nitrogen fixation assimilating NH4+, NO3−, or NO2− under microaerobic conditions, while also aid denitrifying under anaerobic conditions.30 Its presence in the cathode could facilitate the reduction from PNP to PAP. Geobacter is a wellknown anode-respiring bacteria (ARB) which is able to generate electricity in MFCs.31 It was also found in microbial fuel cells with high phenol removal efficiency.32 In this study, it existed in the biocathode and might function in voltage generation and PNP degradation. Based on the synergetic effects of the bacteria mentioned above, the PNP reduction was more efficient in the bio-cathode reactors.
3.7 Implications of this work
Aside from MFC systems introduced in this study, nitroaromatic pollutants can also be reduced in MEC systems7,33 and conventional anaerobic systems.34 Performances of different systems in nitroaromatic pollutant reductions are compared in Table 2. In the self-driven MFC system, the voltage output and reduction efficiency are significantly higher than in the abiocathode control. Compared to the MEC system with power input, slightly higher reduction efficiency (100% vs. 96.2%) and much shorter reduction time (50 h vs. 120 h) are the advantage of the self-driven MFC system. However, the formation efficiencies of corresponding amines are enhanced remarkably in the MEC system (88.3%) than the MFC system (40.6%). The phenomenon may be due to the relatively stronger driving force of the electrical energy input into the MEC system. The effect is also implied by the smaller gap between the biocathode and abiocathode in the MEC system. Nevertheless, the reduction process in the self-driven MFC system is totally driven by the microbes on the electrodes, and no energy input is applied. The degradation of PNP in the UASB system shows shorter retention time (30 h vs. 50 h), but under lower PNP loading (0.25 mM vs. 0.36 mM) than in the MFC system. Meanwhile, the efficiencies of PNP reduction and PAP formation are similar in the two systems.
Table 2 Comparison of nitroaromatic pollutant reductions in different systems
Reactor |
Voltage output |
Pollutants |
Time |
Effremoval-maxa |
Effformation-max |
Reference |
Eff: efficiency. UASB: upflow anaerobic sludge blanket. |
MFC-biocathode (self-driven) |
+0.06 V |
PNP (0.36 mM) |
50 h |
100% |
40.6% (PNP to PAP) |
This study |
MFC-abiocathode |
+0.04 V |
PNP (0.36 mM) |
— |
70.2% |
7.8% (PNP to PAP) |
This study |
MEC-biocathode |
−0.15 V (input) |
NB (0.5 mM) |
120 h |
96.2% |
88.3% (NB to AN) |
7 |
MEC-abiocathode |
−0.15 V (input) |
NB (0.5 mM) |
— |
94.3% |
75.6% (NB to AN) |
7 |
UASBb |
— |
PNP (0.25 mM) |
30 h (HRT) |
98.4% |
47.4% (PNP to PAP) |
34 |
These results strongly demonstrate that the self-driven MFC system holds great potentials for efficient removal of nitroaromatic pollutants. Most importantly, among the different treatment systems, MFC system is the only one which could produce power (voltage output) during reduction process. Therefore, the self-driven MFC system could be a promising strategy to reduce nitroaromatic pollutants efficiently and economically.
4. Conclusions
Nitrophenols were degraded to aminophenols in a self-driven MFC system without any energy input. Compared to abiotic cathodes, biotic cathodes were superior in higher PNP reduction rates, higher PAP formation efficiency, higher current generation, and lower electron donor consumption. PNP reduction was enhanced by decreasing external resistance and increasing TDS. The microbes on the bio-cathodes facilitated and improved the PNP reduction process, and also promoted the system performance. The self-driven MFC system could be a promising alternative to current technologies for treating nitroaromatic pollutants.
Acknowledgements
This research is financed by National Science Foundation of China (No. 51348007, 51508275 and 51378261), Specialized Research Fund for the Doctoral Program of Higher Education (No. 2013321912001), China Postdoctoral Science Foundation (No. 2014M560426), Jiangsu Postdoctoral Science Foundation (No. 1302100C).
References
- H. Liu, T. Hu, G. Zeng, X. Yuan, J. Wu, Y. Shen and L. Yin, Int. Biodeterior. Biodegrad., 2013, 76, 108–111 CrossRef CAS.
- J. Shen, C. Feng, Y. Zhang, F. Jia, X. Sun, J. Li, W. Han, L. Wang and Y. Mu, J. Hazard. Mater., 2012, 209–210, 516–519 CrossRef CAS PubMed.
- M. Kulkarni and A. Chaudhari, J. Environ. Manage., 2007, 85, 496–512 CrossRef CAS PubMed.
- J. Shen, X. Xu, X. Jiang, C. Hua, L. Zhang, X. Sun, J. Li, Y. Mu and L. Wang, Water Res., 2014, 67, 11–18 CrossRef CAS PubMed.
- R. Liu, W. Li, G. Sheng, Z. Tong, M. H. Lam and H. Yu, Electrochim. Acta, 2015, 154, 294–299 CrossRef CAS.
- Y. Mu, R. A. Rozendal, K. Rabaey and J. Keller, Environ. Sci. Technol., 2009, 43, 8690–8695 CrossRef CAS PubMed.
- A. Wang, H. Cheng, B. Liang, N. Ren, D. Cui, N. Lin, B. H. Kim and K. Rabaey, Environ. Sci. Technol., 2011, 45, 10186–10193 CrossRef CAS PubMed.
- M. Sun, D. D. Reible, G. V. Lowry and K. B. Gregory, Environ. Sci. Technol., 2012, 46, 6174–6181 CrossRef CAS PubMed.
- J. Li, G. Liu, R. Zhang, Y. Luo, C. Zhang and M. Li, Bioresour. Technol., 2010, 101, 4013–4020 CrossRef CAS PubMed.
- H. Liu and B. E. Logan, Environ. Sci. Technol., 2004, 38, 4040–4046 CrossRef CAS PubMed.
- Y. Zhu, Y. Zhang, H. Ren, J. Geng, K. Xu, H. Huang and L. Ding, Bioresour. Technol., 2015, 180, 345–351 CrossRef CAS PubMed.
- B. Liang, H. Cheng, J. D. Van Nostrand, J. Ma, H. Yu, D. Kong, W. Li, N. Ren, L. Wu, A. Wang, D. J. Lee and J. Zhou, Water Res., 2014, 54, 137–148 CrossRef CAS PubMed.
- Y. Xiao, Y. Zheng, S. Wu, Z. Yang and F. Zhao, Microb. Ecol., 2015, 69, 492–499 CrossRef CAS PubMed.
- Q. Wen, T. Yang, S. Wang, Y. Chen, L. Cong and Y. Qu, J. Hazard. Mater., 2013, 244–245, 743–749 CrossRef CAS PubMed.
- G. Ji, T. Sun, J. Ni and J. Tong, Bioresour. Technol., 2009, 100, 1108–1114 CrossRef CAS PubMed.
- L. Huang, X. Chai, X. Quan, B. E. Logan and G. Chen, Bioresour. Technol., 2012, 111, 167–174 CrossRef CAS PubMed.
- L. Huang, Q. Wang, X. Quan, Y. Liu and G. Chen, Bioelectrochemistry, 2013, 94, 13–22 CrossRef CAS PubMed.
- Y. Li, Z. Huang, W. Ruan, H. Ren and M. Zhao, J. Chem. Technol. Biotechnol., 2015, 90, 139–148 CrossRef CAS.
- S. Geng, X. Pan, R. Mei, Y. Wang, J. Sun, X. Liu, Y. Tang and X. Wu, Curr. Microbiol., 2014, 68(3), 324–329 CrossRef CAS PubMed.
- S. Spring, U. Jäckel, M. Wagner and P. Kämpfer, Int. J. Syst. Evol. Microbiol., 2004, 54, 99–106 CrossRef CAS PubMed.
- J. Cao, Q. Lai, Y. Liu, G. Li and Z. Shao, Int. J. Syst. Evol. Microbiol., 2014, 64, 963–967 CrossRef CAS PubMed.
- C. Jayashree, P. Arulazhagan, S. A. Kumar, S. Kaliappan, I. T. Yeom and J. R. Banu, Biomass Bioenergy, 2014, 69, 249–254 CrossRef CAS.
- Y. Liu, J. Liu, C. Li, J. Wen, R. Ban and X. Jia, Biochem. Eng. J., 2014, 90, 316–323 CrossRef CAS.
- Q. Wang, Y. Li, J. Li, Y. Wang, C. Wang and P. Wang, Environ. Sci. Pollut. Res., 2015, 22, 565–573 CrossRef CAS PubMed.
- B. Liang, H. Cheng, D. Kong, S. Gao, F. Sun, D. Cui, F. Kong, A. Zhou, W. Liu, N. Ren, W. Wu, A. Wang and D. Lee, Environ. Sci. Technol., 2013, 47, 5353–5361 CrossRef CAS PubMed.
- S. Chen and X. Dong, Int. J. Syst. Evol. Microbiol., 2005, 55, 2257–2261 CrossRef CAS PubMed.
- S. B. Larsen, D. Karakashev, I. Angelidaki and J. E. Schmidt, J. Hazard. Mater., 2009, 164, 1568–1572 CrossRef CAS PubMed.
- H. Park, S. Sundar, Y. Ma and K. Chandran, Biotechnol. Bioeng., 2015, 112, 272–279 CrossRef CAS PubMed.
- Y. Liang, D. Li, X. Zhang, H. Zeng, Z. Yang, S. Cui and J. Zhang, Bioresour. Technol., 2015, 175, 189–194 CrossRef CAS PubMed.
- D. Cho, R. Ramanan, J. Heo, J. Lee, B. Kim, H. Oh and H. Kim, Bioresour. Technol., 2015, 175, 578–585 CrossRef CAS PubMed.
- T. Yoshizawa, M. Miyahara, A. Kouzuma and K. Watanabe, J. Biosci. Bioeng., 2014, 118, 533–539 CrossRef CAS PubMed.
- G. Buitrón and I. Moreno-Andrade, Appl. Biochem. Biotechnol., 2014, 174, 2471–2481 CrossRef PubMed.
- J. Shen, Y. Zhang, X. Xu, C. Hua, X. Sun, J. Li, Y. Mu and L. Wang, Water Res., 2013, 47, 5511–5519 CrossRef CAS PubMed.
- K. Karim and S. K. Gupta, Bioresour. Technol., 2001, 80, 179–186 CrossRef CAS PubMed.
|
This journal is © The Royal Society of Chemistry 2016 |