DOI:
10.1039/C6RA03766F
(Paper)
RSC Adv., 2016,
6, 36171-36179
Role of capping agents in controlling silver nanoparticles size, antibacterial activity and potential application as optical hydrogen peroxide sensor†
Received
10th February 2016
, Accepted 5th April 2016
First published on 6th April 2016
Abstract
The influence of capping agents on silver nanoparticles (AgNPs) was investigated through a rapid and single-pot chemical reduction method. Four capping agents were tested: polyethylene glycol (PEG), ethylenediaminetetraacetic acid (EDTA), polyvinyl pyrrolidone (PVP) and polyvinyl alcohol (PVA). FTIR studies demonstrated that the formed AgNPs were properly encapsulated by their respective capping agents. Structural and morphological studies confirmed the following relative average particle sizes: PEG-AgNPs > EDTA-AgNPs > PVP-AgNPs > PVA-AgNPs. Optical absorption and photoluminescence studies showed, respectively, a greater absorption blue shift and greater emission intensity for the smaller capped particles. Zeta potential analysis of the PVA-AgNPs showed a value of −46.6 mV, indicating their high stability. The PVA-AgNPs were thus not only observed to be the smallest, most blue-shifted and most stable of the tested AgNPs, but also they displayed the highest antibacterial activity. The PVA-AgNPs were therefore applied as a localized surface plasmon resonance (LSPR)-based H2O2 sensor, which is important because the detection of reactive oxygen species such as H2O2 is of significance in the medical and environmental fields. The sensor based on the PVA-AgNPs successfully detected H2O2 at concentrations as low as 10−7 M. New biosensors using these NPs should thus find promising opportunities in a variety of fields.
1. Introduction
Recent advancements in nanotechnology have garnered increasing attention due to the improvements in producing and utilizing particles whose sizes are in the nanometer regime. There is particular interest in noble metal nanoparticles due to their distinctive properties, such as their optical, mechanical, electronic, chemical and magnetic properties which are entirely different from corresponding bulk material properties.1–3 Silver nanoparticles (AgNPs) have been of particular interest owing to their unique electrical, optical and physicochemical properties as well as their biomedical applications.4 AgNPs show good conductivity, chemical stability, catalytic, antibacterial activity and surface enhanced Raman spectroscopy (SERS) effects, and also constitute an ideal candidate for molecular labeling because of their large operative scattering cross section and surface plasmon resonance (SPR).5,6 Metal nanoparticles in general can be synthesized by various approaches such as chemical,7 electrochemical,8 chemical vapor deposition,9 photochemical,10 molecular beam epitaxy,11 and chemical reduction with and without stabilizing polymers,7,12 which is a particularly widely used chemical approach. Note that experimental factors, the interaction of metal precursor ions with reducing agents, and capping agent interface with metal nanoparticles greatly influence the size, shape, stability and physicochemical properties of the metal nanoparticles.13,14
In contrast to bulk material, the corresponding nanoparticles exhibit high surface energy values, due to their small sizes, and hence often congregate.15 Therefore, selecting an appropriate capping agent is often a prerequisite for stabilizing nanoparticles.16 Capping agents appear to work by using a variety of mechanisms, including electrostatic stabilization, steric stabilization, stabilization by hydration forces, depletion stabilization and stabilization using van der Waals forces,16 and a combination of some of these mechanisms may be at work for certain capping agents, such as branched polyethylenimine (BPEI).17 The choice of capping agent for nanoparticles is crucial, because the capping agent often influences various properties of the nanoparticle, including its size, shape and interactions with surrounding solvent.18 Apart from their main role in stabilizing nanoparticles, some capping agents (e.g., citrate) are capable of carrying out the additional role of reducing metal ions (e.g., silver ions) into metal nanoparticles.19 Therefore, the selection of the capping agent plays a vital role in the nanoparticle synthesis process, and capping agents also affect nanoparticle properties.20
Well-stabilized nanoparticles are important for their functions and applications, including their ability to serve as sensors of chemicals in the environment. One such chemical of concern is hydrogen peroxide (H2O2). H2O2 is a strong reducing agent and widely utilized in the food and pharmaceutical industries as well as for the bleaching of wood and pulp. However, H2O2 even in small amounts is highly toxic and is a serious threat to biological systems.21 Therefore it has been an upsurge of interest to develop H2O2 sensors in order to detect H2O2 promptly and accurately. The development of nanomaterial-based sensing devices and detection methods has largely relied on the use of localized surface plasmon resonance (LSPR) since the resonant wavelength is very sensitive to the environment surrounding the nanoparticles being analyzed. Noble metal nanostructures such as gold and silver are applicable as LSPR-based optical and chemical sensors/biosensors and are expected to be of use in high-sensitivity detection of target molecules in medical and food control applications.22,23 Due to the simplicity and low cost of fabricating hydrogen peroxide sensors, the analytical detection of hydrogen peroxide is extensively used in pharmaceutical, environmental, industrial and clinical research.24 Recently, Endo et al.25 developed an LSPR-based optical biosensor using PVP-capped AgNPs for the detection of hydrogen peroxide. Tagad et al.26 constructed a H2O2 sensor based on silver nanoparticles stabilized by polysaccharides.
In the present study, we synthesized spherical AgNPs in the presence of different stabilizing capping agents through a simple chemical reduction method, and we optimized various features of the AgNPs produced. The capping agents tested included polyethylene glycol (PEG), ethylenediaminetetraacetic acid (EDTA), polyvinylpyrrolidone (PVP) and polyvinyl alcohol (PVA), and we determined the effects of these capping agents on the sizes of the resulting AgNPs. In addition, as part of the effort to develop improved detectors of H2O2, the current work focused on applying our synthesized AgNPs as an LSPR-based H2O2 optical sensor, and the characteristics of this sensor was evaluated by carrying out a UV-visible spectrophotometric analysis. We also evaluated the synthesized AgNPs for their antibacterial activity.
2. Experimental
2.1 Materials
Silver nitrate (AgNO3), sodium borohydride (NaBH4), ethanol (C2H5OH), PEG (C2nH4n+2On+1), PVP ((C6H9NO)n), EDTA (C10H16N2O8), PVA ((C2H4O)n) and sodium hydroxide (NaOH) were purchased from Sigma Aldrich. All the chemicals were of analytical reagent grade and used without further purification.
2.2 Procedure used to synthesize silver nanoparticles with different capping agents
In a typical procedure, say when using the capping agent PEG, the total volume of the reaction solution is fixed at 150 ml. 1 ml of 1% PEG aqueous solution is slowly added drop wise for 5 min into 98.5 ml of ultrapure water in a 250 ml three-necked flask, while heating at 75 °C in an oil bath under stirring. Then, 50 ml of 0.01 M aqueous AgNO3 solution is injected in to the above mixture using a programmable syringe pump at a rate of 50 ml h−1 and 150 μl of sodium hydroxide (0.02 M) solution is added rapidly at once. This is followed by the injection of ice cold freshly prepared aqueous NaBH4 solution (350 μl, 0.05 M) using a syringe pump with infusion rate set at 50 μl min−1 upon vigorous stirring; the color of this suspension changes to deep yellow. The reaction was allowed to proceed for two hours, after which the solution was cooled to room temperature. The products were obtained by repeated centrifugation for thrice and washed with water and ethanol twice at 4 °C in order to remove unreacted constituents and were finally subjected to vacuum drying. The same procedure was used with the other capping agents, which included EDTA, PVP and PVA. The final products (∼4.5 mg of each sample) were transferred into amber bottles and stored for further analysis.
2.3 Characterization of the materials
The synthesized AgNPs were characterized comprehensively by using a variety of techniques. The crystalline structure of the synthesized AgNPs was analyzed using a Seifert 3003 TT X-ray diffractometer with Cu Kα radiation (k = 0.1546 nm). The surface morphology of the AgNPs was visualized using a ZEISS, SUPRA 55 field emission scanning electron microscope (FESEM). A transmission electron microscopy (TEM) study was conducted using a Technai TF30 ST field emission transmission electron microscope (FE-TEM) working at an accelerating voltage of 300 kV. UV–visible absorption spectra were acquired on a Perkin Elmer Lambda 950 UV-Vis-NIR spectrophotometer with the wavelength set in the range 200–800 nm at a resolution of ±0.2 nm. Photoluminescence spectra were recorded using a Horiba Jobin–Yvon Fluorolog-3 spectrofluorometer (FL3-22PTI). Fourier transform infrared spectroscopy (FTIR) spectra of AgNPs were taken with an ATR-FTIR Bruker Vertex-80 spectrometer. The zeta potential of the prepared AgNPs was measured using a Nanopartica instrument (HORIBA).
2.4 Antibacterial assay of the synthesized AgNPs
The antibacterial activities of the synthesized AgNPs with different capping agents were tested against the bacterial isolates such as Escherichia coli and Pseudomonas spp. The antibacterial properties of these different AgNPs were investigated by employing standard Kirby–Bauer's disc diffusion method.27 The above-mentioned bacterial pathogens were cultured overnight in nutrient broth at 37 °C. The nutrient agar plates were prepared by dissolving the nutrient agar in distilled water and autoclaved and then allowed for solidification before adding the cultures through lawn culture method. Then, Whatman no. 1 filter paper discs impregnated with a volume of 6 μl of the AgNPs synthesized with different capping agents and dried under aseptic conditions, were placed in separate appropriately labeled plates. The plates were incubated at 37 °C for 24 h to assess the activity of the AgNPs. The size (in mm) of the clear zone of inhibition around each disc after the incubation period was recorded.
2.5 LSPR-based hydrogen peroxide detection method using PVA-capped AgNPs
The optical characteristics of PVA-capped AgNPs serving as an LSPR-based sensor of hydrogen peroxide were evaluated using various concentrations of H2O2 as specified in the previously used protocol.25 At first, a volume of 1 ml of a hydrogen peroxide solution of a specified concentration was diluted with 20 mM phosphate buffer (pH 7.4), and the resulting solution was added to PVA-capped AgNPs in a quartz cuvette and thoroughly mixed for evaluation of optical characteristics using a UV-vis spectrophotometer. The changes in the optical spectra of the AgNPs at a wavelength of about 425 nm were monitored at regular intervals of time (0, 5, 10, 15, 20 and 30 min).
3. Results and discussion
3.1 Structural analysis
The structures of the AgNPs synthesized with different capping agents were investigated using X-ray diffraction (XRD) analysis. The XRD spectra with 2θ values ranging from 20° to 80° depicted four peaks corresponding to diffraction from the (111), (200), (220) and (311) planes (Fig. 1). Diffraction from the (111) plane was strongest. All the peaks were indexed based on the face-centered cubic (FCC) lattice of silver, and were consistent with the standard JCPDS (no. 04-0783) data. The average crystallite size was estimated from the FWHM of the diffraction peak using the Debye–Scherrer formula28 |
D = Kλ/β cos θ,
| (1) |
where K denotes Scherrer's constant (K = 0.94), D corresponds to crystallite size, β is the full width at half maximum (FWHM) of the XRD peak and is expressed in radians, and θ is the position of the diffraction peak. The FWHM was calculated using (111) preferred orientation and the average crystallite size was found to range from a high of 39 nm for the PEG-capped AgNPs to a low of 26 nm for the PVA-capped AgNPs.
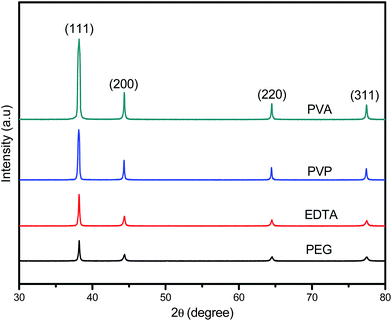 |
| Fig. 1 Representative XRD profiles of synthesized silver nanoparticles with different capping agents. | |
3.2 Morphological studies
FESEM measurements were taken to obtain the particle size distribution of the as-synthesized AgNPs. The FESEM images (Fig. 2) clearly showed the formation of spherical AgNPs synthesized with the different capping agents.
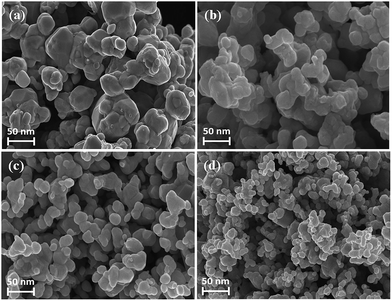 |
| Fig. 2 FESEM images of AgNPs prepared with (a) PEG, (b) EDTA, (c) PVP and (d) PVA. | |
The PEG-capped AgNPs were observed to agglomerate to a modest extent into larger particles, while the others agglomerated to lesser extents. The least agglomeration was observed for the PVA-capped AgNPs, which for the most part formed isotropic spherical nanoparticles. The average particle sizes of the PEG, EDTA, PVP and PVA capped AgNPs were found to be 44, 39, 35 and 31 nm, respectively. Therefore, PVA capped AgNPs are optimized as a smallest particle size.
The shapes and sizes of the synthesized AgNPs with different capping agents were further examined by using TEM. The typical TEM micrographs of PEG and PVA-capped AgNPs are shown in Fig. 3(a) and (b), and the average particle size was found to be 41 nm for the PEG-capped AgNPs and 27 nm for the PVA-capped AgNPs.
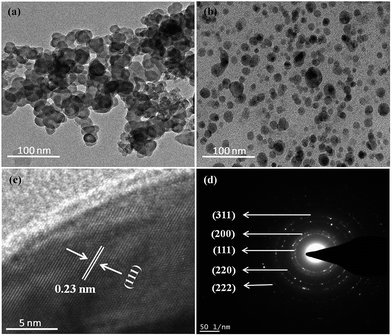 |
| Fig. 3 TEM images of AgNPs synthesized with (a) PEG and (b) PVA. (c) Typical HR-TEM and (d) SAED pattern of AgNPs prepared with PVA. | |
In these micrographs, the more spherical isotropic particles appeared smaller in size whereas the nonspherical ones appeared larger. For the PEG-capped case, the smaller particles apparently agglomerated to form larger nonspherical particles, perhaps because of a decreased interaction between the PEG and AgNPs relative to the interactions between the other capping agents and the AgNPs. Less agglomeration was observed for the PVA-capped AgNPs than for the others, and the PVA-capped AgNPs were observed to be generally spherical and homogeneously dispersed. Multiple and equispaced lattice fringes were clearly observed in the high-resolution TEM of the PVA-capped AgNPs (Fig. 3(c)). The lattice spacing (d) of the (111) plane was found to be 0.23 nm and is consistent with JCPDS data (no. 04-0783). Finally, the PVA-capped AgNPs were found to be smaller than the AgNPs made with the other capping agents. The PVA-capped AgNPs were also analyzed by electron diffraction directly on the transmission electron microscope. The selected-area electron diffraction (SAED) pattern (Fig. 3(d)) showed obvious bright circular rings mixed with several diffraction spots attributed to the polycrystalline structure of the formed AgNPs.
3.3 Optical properties
UV-vis absorption spectroscopy is a fundamental technique for assessing the formation of stable AgNPs, since metal nanoparticles have been shown to exhibit an intense absorption peak due to the surface plasmon resonance (SPR) phenomenon (collective oscillation of surface electrons) at 395–425 nm.29 According to Mie's theory30 only a single SPR band is expected in the absorption spectrum of spherical nanoparticles, whereas two or more SPR bands are expected for anisotropic nanoparticles, depending on the specific shapes of the particles. The number of SPR peaks increases as the symmetry of the nanoparticles decreases.31
Fig. 4(a) displays the absorption spectra of the AgNPs prepared with different capping agents. A single and fairly symmetrical SPR band was exhibited by each of the AgNP samples in the range of 440–420 nm. AgNPs synthesized with PEG, EDTA, PVP and PVA yielded maximum absorption peaks at 445, 438, 431 and 425 nm, respectively, indicating the formation of relatively small nanoparticles with narrow size distributions. The SPR data was well correlated with the shapes of the synthesized nanoparticles in the micrographs described above. From this, we can state that the relatively short wavelength at maximum absorption for the PVA-capped AgNPs was due to the relatively small average size of the particles.
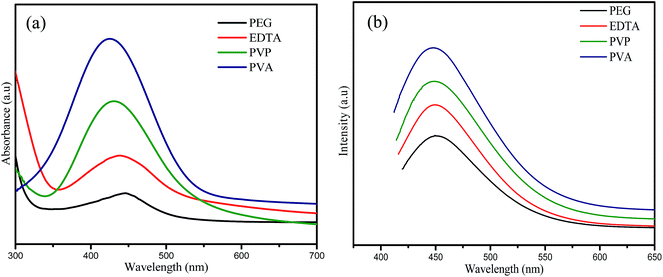 |
| Fig. 4 (a) UV-vis absorption spectra and (b) PL spectra of the synthesized AgNPs with the various capping agents. | |
To further explore the size-dependent optical properties, we carried out photoluminescence studies. Fig. 4(b) shows the PL spectra of the AgNPs synthesized with the PEG, EDTA, PVP and PVA capping agents, respectively. It has been suggested that the photoemission wavelength is independent of the particle size, while the intensity increases with decreasing particle size.32 Irrespective of the capping agent used, all AgNPs excited with 400 nm wavelength light yielded a maximum emission at a similar wavelength, mainly located in the visible region at 450 nm. The PVA-capped AgNPs yielded the most intense emission, consistent with their smallest average particle size. As the AgNPs were excited in the SPR range, the radiation absorbed by these nanoparticles is thought to be radiative energy transfer to the emitting particles.
3.4 FTIR studies
In order to determine the possible functional groups involved in reduction and capping process and their unique interactions with AgNPs, FTIR spectroscopy studies were undertaken. Fig. 5(a) shows the FTIR spectra of the AgNPs synthesized with the PEG, EDTA, PVP and PVA capping agents, respectively. PEG-capped AgNPs yielded a broad peak centered around 3412 cm−1 attributed to the stretching vibration of –OH group. The spectral bands at 1070 cm−1, 1260 cm−1, 1380 cm−1, and 1587 cm−1 arose from the C–OH stretching, C–O–C asymmetric stretching, alkyl C–H deforming vibrations, and C–H mode vibrations, respectively. The small peaks at 530 cm−1 and 415 cm−1 correspond to the AgNPs bonding with oxygen of hydroxyl groups of PEG units, and C–C skeletal vibrations were observed at 989 and 840 cm−1. All of the above spectral peaks are in accordance with the previous report and reveal that PEG stabilized the NPs through steric and electrostatic interactions of –OH groups.33 EDTA-capped AgNPs yielded absorptions at 1061 cm−1, 1401 cm−1, 1632 cm−1 and 3446 cm−1, which were assigned to C–O single-bond vibrations, asymmetric carboxylate group stretching, amide-I C
O stretching, and O–H stretching vibrations, respectively.34 This IR spectrum strongly confirmed the capping of the AgNPs through direct bonding of EDTA.
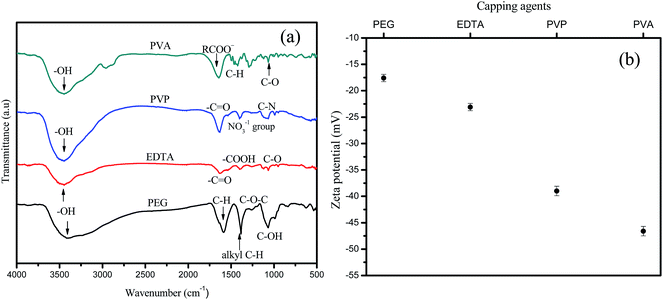 |
| Fig. 5 (a) FTIR spectra and (b) zeta potential values for AgNPs synthesized with different capping agents. | |
Each repeating unit of PVP has two functional groups, a C–N group and a C
O group. These groups, due to their N and O atoms, have great affinity for silver ions and can coordinate metallic silver.35 PVP-coated AgNPs yielded main absorption of C–N peak of pure PVP at 1019 cm−1 which is separated into two peaks at 1056, and 1007 cm−1.36 Other peaks at 1378 cm−1, 1631 cm−1, 3491 cm−1 are characteristic of bond vibrations of the NO3−1 group, C
O bonds, and O–H stretching vibrations, respectively.36 Thus, the FTIR spectra showed the presence of molecular interactions between AgNPs and PVP chain.
PVA-capped AgNPs yielded strong absorption peaks at 3446 cm−1, 1641 cm−1, 1420 cm−1, and 1062 cm−1, which were ascribed to typical hydroxyl bands, symmetric stretching of carboxylate anion, O–H and C–H bending, and C–O stretching, respectively.7 In addition, small bands were also observed at 2948 and 2897 cm−1, corresponding to the asymmetric and the symmetric stretching of –CH2–, respectively. As a result, we may conclude that all of the synthesized AgNPs were properly encapsulated by their capping agents.
3.5 Zeta potential analysis
Zeta potential is an important parameter for the assessing the stability of AgNPs in aqueous suspensions. Particles that have a large negative or positive zeta potential are likely to repel each other, and thereby have enhanced suspension stability. However, if the particles have low zeta potential values, then attractive forces between the particles become dominant and particles undergo aggregation and flocculation. In general, a minimum value of ±30 mV for the zeta potential is necessary to validate a stable nanosuspension.37 Fig. 5(b) shows the zeta potential values of the synthesized AgNPs with different capping agents and indicates the high stability of the prepared AgNPs. Moreover, the zeta potential results revealed the synthesized PVA-AgNPs to be more stable than the other AgNP samples. Additionally, the negative zeta potential value (−46.6 mV) of PVA-AgNPs also suggests an active role of the capping agent in augmenting the stability of the AgNPs for a longer period of time than other capped AgNPs.
3.6 Formation of AgNPs with capping agents
Different capping agents (polyethylene glycol (PEG), ethylenediaminetetraacetic acid (EDTA), polyvinylpyrrolidone (PVP) and polyvinyl alcohol (PVA)) were used for the synthesis of AgNPs and formed AgNPs were encapsulated by their own capping agent. In case of PEG, the hydroxyl group acts as a capping agent and can make a cover on the surface of AgNPs. This is probably due to the presence of van der Waals forces between negatively charged oxygen groups present in PEG and positively charged that surround the surface of the inert AgNPs.38 The possible interaction between the positively charged Ag and PEG is shown in Fig. S1(a).† The EDTA molecular structure allows it to completely surround a metal atom (chelating effect) and avoids the metal from reacting with other molecules by forming cage-like structure as EDTA is a bifunctional chelating agent.39 Since, chelating agent may bond to a metal ion in more than one place simultaneously and increase its stability. Herein, the EDTA bond up with the silver ion and the schematic representation of silver ion wrapped by EDTA is shown in Fig. S1(b).†
PVP has a polyvinyl skeleton with polar groups, act as a capping agent for dissolved metallic salts through steric and electrostatic stabilization of amide groups of the pyrrolidone rings. PVP donates loan pair electrons of oxygen and nitrogen atoms to silver ions and thus forms the Ag ions and PVP complex structure.40 The schematic reaction between silver cation and PVP is depicted in Fig. S1(c).† PVA is a non-toxic green polymer and it reacts with silver nitrate resulting in the formation of Ag+ ions bonded to PVA polymer structure. As PVA is heated while stirring, the reactive PVA molecules with activated OH-groups free from H-bonding are formed.41 Thus, these active OH-groups act as head-groups to adsorb Ag+ cations and drive a directional growth, Ag+ → Ag0, which eventually produce a Ag-metal attached to the PVA surface (Fig. S1(d)†).
3.7 Antibacterial studies
The antibacterial properties of the AgNPs with different capping agents (PEG, EDTA, PVP and PVA) were analyzed by means of Kirby–Bauer's disc diffusion method on the basis of the zone of inhibition. The antibacterial activity of the AgNPs is solely due to physical effects, such as adsorption of AgNPs to the cell wall, interaction of Ag ions with the cell wall protein, denaturation of the cell wall proteins, and the formation of porous structures along with severe changes in the membrane structure of the bacteria, which all increase the permeability and accumulation of external fluids. In particular, AgNPs can damage the structure of the cell membrane by anchoring onto and penetrating the membrane through electrostatic attractions, and hence deactivate certain membranous enzymes, dissipate the proton motive force, and finally cause cell lysis.42,43 Fig. 6 shows the antibacterial activity of the AgNPs synthesized with the various capping agents. Martínez-Castañón et al.44 reported that relatively small particles display relatively high antibacterial activity. The PVA-capped AgNPs, shown above to be small, indeed showed better antibacterial efficacy than the other AgNPs (Fig. 6). Also, the high aspect ratio and the crystallographic structure of the nanoparticles govern their antimicrobial potency, which are favored by {111} facets.45
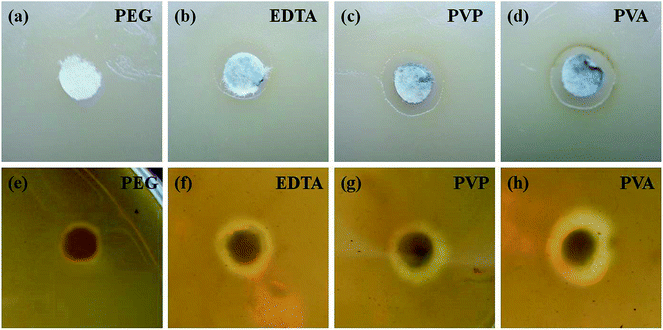 |
| Fig. 6 Bactericidal activity of AgNPs with different capping agents against E. coli (a–d) and Pseudomonas spp. (e–h). | |
The AgNPs exhibited better antibacterial efficacy by inhibiting the growth and division of pathogenic bacteria like Escherichia coli and Pseudomonas spp. In general, AgNPs destabilize the outer membrane and split the plasma membrane, thereby causing reduction of intracellular ATP.46 The sizes in millimeters of the zones of inhibition of the respective discs loaded with different concentrations of AgNPs against the tested pathogenic bacteria are listed in Table 1. Of the different capping agents tested, the PVA-capped AgNPs yielded the largest such zones of inhibition, which revealed these AgNPs to be novel antibacterial agents due to their small average size.
Table 1 Antibacterial activity of AgNPs, measured against different pathogenic organisms
AgNPs capping agent |
Zones of inhibition (mm) |
Escherichia coli |
Pseudomonas spp. |
PEG |
3 |
3 |
EDTA |
4 |
5 |
PVP |
6 |
6 |
PVA |
7 |
8 |
3.8 Evaluation of PVA-capped AgNPs as LSPR-based optical hydrogen peroxide sensors
The catalytic reaction between PVA-capped AgNPs and hydrogen peroxide would be expected to inevitably result in the decomposition of hydrogen peroxide, and induce the degradation of clustered AgNPs through PVA layer with their oxidation (Fig. 7(a) and (b)). The dependence of the absorbance of the PVA-capped AgNPs on its concentration is shown in Fig. 7(c). The absorption intensity of the PVA-capped AgNP increased as its concentration was increased from 0.0005% to 0.001% and to 0.002%. As a result, the 0.002% concentration of the PVA-capped AgNP solution was chosen as a hydrogen peroxide sensor.
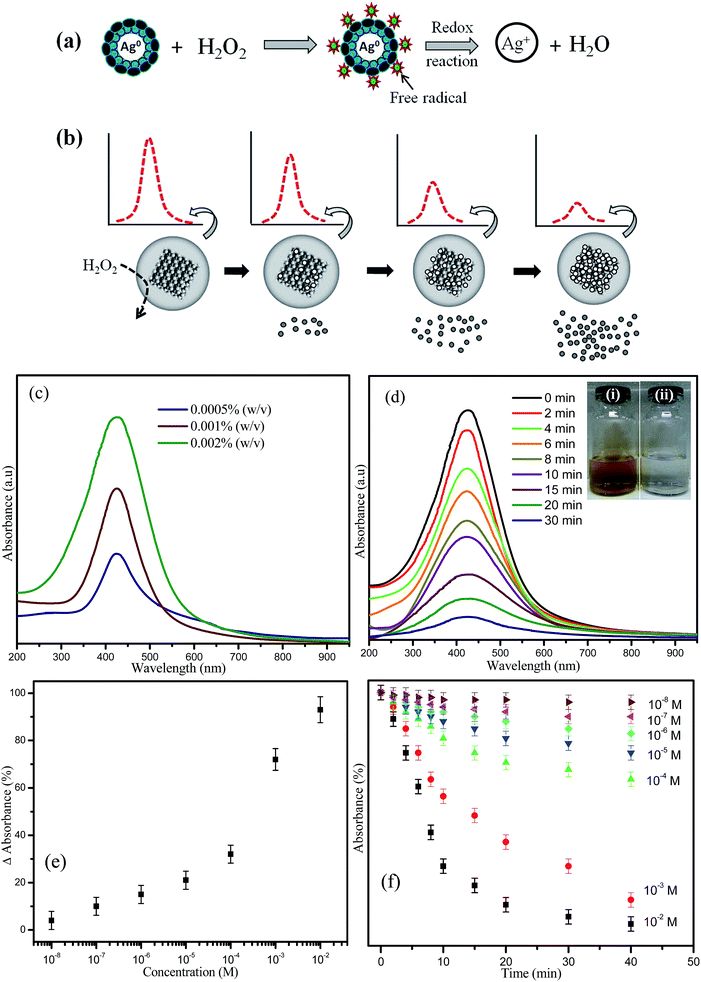 |
| Fig. 7 Possible mechanism and schematic representation of (a) the reaction between PVA-coated AgNPs and H2O2, and (b) the decrease of absorbance intensity with increasing H2O2 concentration. (c) Optical absorption characteristics of different concentrations of PVA-capped AgNPs. (d) LSPR optical absorbance spectra of AgNPs as a function of time after the addition of 10−3 M H2O2. (Inset: AgNPs solution (i) before and (ii) after addition of H2O2). (e) The correlation between the optical absorbance strength changes after 30 min with respect to different concentrations of H2O2 added to the PVA-AgNPs. (f) Relationship between the absorbance intensity and reaction time for different concentrations of H2O2. | |
Fig. 7(d) shows the change in the LSPR-based optical H2O2 sensor absorbance characteristics of the PVA-capped AgNPs with time due to the introduction of 1 mM (10−3 M) H2O2. As shown in the inset of Fig. 7(d), the signature yellow color (vial (i)) disappeared and finally became colorless (vial (ii)) after 30 min. The absorbance was observed to decrease with time of reaction, and after 30 min a drastic change in its optical absorbance characteristics was observed. The observed decrease in the absorbance intensity was attributed to the decrease in the AgNP concentration. The change in color of the AgNP solution and decrease of the LSPR peak absorbance were attributed to the ability of silver to catalyze the decomposition of hydrogen peroxide.47 Hence, a notable change in the intensity of the LSPR absorbance was possibly observed.
In order to prove that the catalytic reaction involving hydrogen peroxide was the major reason for the decrease in the intensity of the LSPR absorbance with time, a control in which only phosphate buffer and distilled water were added to the PVA-capped AgNPs was also analyzed. In this case, no change in the intensity of the LSPR absorbance was observed. Based on the aforementioned mechanism of the LSPR-based optical sensors and the experimental conditions, an assessment of the calibration characteristics was performed. For this purpose, different concentrations of hydrogen peroxide were added to the PVA-capped AgNP solution and the change of the absorbance with time was monitored. The change in the intensity of the absorbance after 30 minutes of reaction time with respect to different concentrations of H2O2 (Fig. 7(e)), and change in absorbance strength with reaction time for different H2O2 concentrations were measured (Fig. 7(f)). Furthermore, the detection limit of this optical sensor was determined to be 10−7 M.
4. Conclusions
In the present investigation, AgNPs were synthesized using different capping agents by using a simple chemical reduction route. The X-ray diffraction analysis revealed a face-centered cubic lattice for all four tested types of capped AgNPs, but indicated that the average crystallite size was greatest for the PEG-capped nanoparticles and smallest for the PVA-capped ones. Morphological studies revealed the formation of spherically shaped particles, and visually confirmed the PVA-capped particles to have the smallest diameters. Optical studies revealed the blue shift trend due to the decrease of particle size with different capping agents. The PVA-capped AgNPs were not only observed to be the smallest and most blue-shifted of the tested AgNPs, but were also observed to be the most stable and to display the highest antibacterial activity. For these reasons, PVA-capped AgNPs were chosen for use in an LSPR-based hydrogen peroxide sensor. The LSPR absorbance strength was observed to change markedly with changes in the hydrogen peroxide concentration and time of reaction due to the catalytic degradation of the AgNPs. The ability of AgNPs to catalyze the reduction of H2O2 was thus successfully applied to the fabrication of an optical sensor of H2O2. Furthermore, this detection method is simple, relatively inexpensive, and reliable, and can be suitably employed in medical and environmental monitoring.
Acknowledgements
This research was supported by the National Research Foundation of Korea (NRF-2015K1A4A3047100, NRF-2015M3A7B6027973, and NRF-2015M3A6B3068660).
References
- J. Prikulis, F. Svedberg and M. Krall, J. Nanosci. Lett., 2004, 4, 115–118 CrossRef CAS.
- S. He, J. Yao, P. Jiang, D. Shi, H. Zhang, S. Pang and H. Gao, Langmuir, 2001, 171, 571–575 Search PubMed.
- B. Wiley, Y. Sun, B. Mayers and Y. Xia, Chem.–Eur. J., 2005, 11, 454–463 CrossRef CAS PubMed.
- A. Ravindran, P. Chandran and S. S. Khan, Colloids Surf., B, 2013, 105, 342–352 CrossRef CAS PubMed.
- C. N. Lok, C. M. Ho, R. Chen, Q. Y. He, W. Y. Yu, H. Sun, P. K. H. Tam, J. F. Chiu and C. M. Che, J. Proteome Res., 2006, 5, 916–924 CrossRef CAS PubMed.
- C. F. Mao and M. A. Vannice, J. Catal., 1995, 154, 230–244 CrossRef CAS.
- B. Ajitha, Y. A. K. Reddy and P. S. Reddy, Powder Technol., 2015, 269, 110–117 CrossRef CAS.
- S. A. Vorobyova, A. I. Lesnikovich and N. S. Sobal, Colloids Surf., A, 1999, 152, 375–379 CrossRef CAS.
- N. Satoh and K. Kimura, Bull. Chem. Soc. Jpn., 1989, 62, 1758–1763 CrossRef CAS.
- Z. Li, Y. Li, X. F. Qian, J. Yin and Z. K. Zhu, Appl. Surf. Sci., 2005, 250, 109–116 CrossRef CAS.
- D. W. Bahnemann, Isr. J. Chem., 1993, 33, 115–136 CrossRef CAS.
- L. M. L. Marzan and A. P. Philipse, J. Phys. Chem., 1995, 99, 15120–15128 CrossRef.
- W. Chen, W. Cai, L. Zhang and G. Wang, J. Colloid Interface Sci., 2001, 238, 291–295 CrossRef CAS PubMed.
- S. Sengupta, D. Eavarone, I. Capila, G. L. Zhao, N. Watson and T. Kiziltepe, Nature, 2005, 436, 568–572 CrossRef CAS PubMed.
- C. N. R. Rao, G. U. Kulkarni, P. J. Thomas and P. P. Edwards, Chem. Soc. Rev., 2000, 29, 27–35 RSC.
- K. D. Kim, D. N. Han and H. T. Kim, Chem. Eng. J., 2004, 104, 55–61 CrossRef CAS.
- A. M. E. Badawy, K. G. Scheckel, M. Suidan and T. Tolaymat, Sci. Total Environ., 2012, 429, 325–331 CrossRef PubMed.
- P. Raveendran, J. Fu and S. L. Wallen, J. Am. Chem. Soc., 2003, 125, 13940–13941 CrossRef CAS PubMed.
- A. Pyatenko, M. Yamaguchi and M. Suzuki, J. Phys. Chem. B, 2005, 109, 21608–21611 CrossRef CAS PubMed.
- J. N. Kuhn, C. K. Tsung, W. Huang and G. A. Somorjai, J. Catal., 2009, 265, 209–215 CrossRef CAS.
- K. R. Hoyt, A. J. Gallagher, T. G. Hastings and I. J. Reynolds, Neurochem. Res., 1997, 22, 333–340 CrossRef CAS PubMed.
- T. Endo, R. Ikeda, Y. Yanagida and T. Hatsuzawa, Anal. Chim. Acta, 2008, 611, 205–211 CrossRef CAS PubMed.
- N. Nath and A. Chilkoti, J. Fluoresc., 2004, 14, 377–389 CrossRef CAS PubMed.
- F. Wang, R. Yuan, Y. Chai and D. Tang, Anal. Bioanal. Chem., 2007, 387, 709–717 CrossRef CAS PubMed.
- T. Endo, Y. Yanagida and T. Hatsuzawa, Measurement, 2008, 41, 1045–1053 CrossRef.
- C. K. Tagad, H. U. Kim, R. C. Aiyer, P. More, T. Kim, S. H. Moh, A. Kulkarni and S. G. Sabharwal, RSC Adv., 2013, 3, 22940–22943 RSC.
- A. L. Barry, F. Garcia and L. D. Thrupp, Am. J. Clin. Pathol., 1970, 53, 149–158 CrossRef CAS PubMed.
- B. D. Cullity, Elements of X-ray Diffraction, Addison-Wesley Pub Inc, MA, 2nd edn, 1978 Search PubMed.
- P. Mulvaney, Langmuir, 1996, 12, 788–800 CrossRef CAS.
- G. Mie, Ann. Phys., 1908, 25, 377–445 CrossRef CAS.
- I. O. Sosa, C. Noguez and R. G. Barrera, J. Phys. Chem. B, 2003, 107, 6269–6275 CrossRef CAS.
- S. Ashokkumar, S. Ravi, V. Kathiravan and S. Velmurugan, Spectrochim. Acta, Part A, 2014, 121, 88–93 CrossRef CAS PubMed.
- S. Tunc and O. Duman, Colloids Surf., A, 2008, 37, 93–99 CrossRef.
- S. Mondal and S. Verma, Z. Anorg. Allg. Chem., 2014, 640, 1095–1101 CrossRef CAS.
- P. Silvert, R. Herrera-urbina, N. Duvauchelle, V. Vijayakrishnan and K. T. Elhsissen, J. Mater. Chem., 1996, 6, 573–577 RSC.
- Z. Zhang, B. Zhao and L. Hu, J. Solid State Chem., 1996, 121, 105–110 CrossRef CAS.
- C. Jacobs and R. H. Muller, Pharm. Res., 2002, 19, 189–194 CrossRef CAS.
- P. Dallas, V. K. Sharma and R. Zboril, Adv. Colloid Interface Sci., 2011, 166, 119–135 CrossRef CAS PubMed.
- K. P. McGrath, B. T. Do and J. G. MacDonald, US Pat., US2005/0084464 A1, 2005.
- Z. Jovanovic, A. N. Radosavljevic, N. Bibic, M. Siljegovic, Z. M. Kacarevic-Popovic and V. B. Miskovic-Stankovic, Radiat. Phys. Chem., 2012, 81, 1720–1728 CrossRef CAS.
- A. Gautam, P. Tripathy and S. Ram, J. Mater. Sci., 2006, 41, 3007–3016 CrossRef CAS.
- J. R. Morones, J. L. Elechiguerra, A. Camacho, K. Holt, J. B. Kouri, J. T. Ramirez and M. J. Yacaman, Nanotechnology, 2005, 16, 2346–2353 CrossRef CAS PubMed.
- J. Kim, E. Kuk and K. Yu, Nanomedicine, 2007, 3, 95–101 CAS.
- G. A. Martínez-Castañón, N. Niño-Martínez, F. Martínez-Gutierrez, J. R. Martínez-Mendoza and F. Ruiz, J. Nanopart. Res., 2008, 10, 1343–1348 CrossRef.
- S. Shrivastava, T. Bera, A. Roy, G. Singh, P. Ramchandrarao and D. Dash, Nanotechnology, 2007, 18, 225103–225112 CrossRef.
- M. J. Hajipour, K. M. Fromm, A. A. Ashkarran, D. J. Aberasturi, I. R. Larramendi, T. Rojo, V. Serpooshan, W. J. Parak and M. Mahmoudi, Trends Biotechnol., 2012, 30, 499–511 CrossRef CAS PubMed.
- T. Pal, D. S. Maity and A. Ganguly, Talanta, 1988, 35, 658–660 CrossRef CAS PubMed.
Footnotes |
† Electronic supplementary information (ESI) available. See DOI: 10.1039/c6ra03766f |
‡ Authors contributed equally. |
|
This journal is © The Royal Society of Chemistry 2016 |