DOI:
10.1039/C6RA04192B
(Paper)
RSC Adv., 2016,
6, 36180-36191
Flexible Janus nanoribbons to help obtain simultaneous color-tunable enhanced photoluminescence, magnetism and electrical conduction trifunctionality†
Received
16th February 2016
, Accepted 3rd April 2016
First published on 5th April 2016
Abstract
A novel nanostructure of {[Eu(BA)3phen + Tb(BA)3phen]/PMMA}//[PANI/Fe3O4/PMMA] Janus nanoribbons with tunable luminescent–electrical–magnetic performance has been successfully fabricated by electrospinning technology using a specially designed parallel spinneret. The morphology and properties of the final products were investigated in detail by X-ray diffractometry (XRD), field emission scanning electron microscopy (FESEM), energy dispersive spectrometry (EDS), biological microscopy (BM), thermogravimetric analysis (TG), a Hall effect measurement system, vibrating sample magnetometry (VSM) and fluorescence spectroscopy. The results reveal that the Janus nanoribbons simultaneously possess excellent luminescent performance, electrical conduction and magnetic properties due to the peculiar nanostructure of Janus nanoribbons. The luminescent–electrical–magnetic trifunctional Janus nanoribbons possess better performances than their counterpart [Eu(BA)3phen + Tb(BA)3phen]/Fe3O4/PANI/PMMA composite nanoribbons which exhibit very weak excitation and emission spectra. The fluorescence intensity of Janus nanoribbons is ca. 70 times higher than that of composite nanoribbons. The color, luminescent intensity, electrical conductivity and magnetic property of the Janus nanoribbons can be tuned by adjusting different amounts of rare earth complexes, PANI and Fe3O4 NPs, respectively. The obtained Janus nanoribbons have potential applications in molecular electronics, microwave absorption and display devices owing to their excellent trifunctionality. Moreover, the construction technique for the Janus nanoribbons is of universal significance for the fabrication of other multifunctional Janus nanoribbons.
Introduction
In recent years, multifunctional materials have attracted inevasible attention of scientists.1–3 Especially, investigation on the luminescent–electrical–magnetic bi- or trifunctional materials has become one of the hot subjects in the field of materials science.4,5 As for luminescent materials, much attention has been paid to the multi-color and color-tunable luminescence for their potential applications in field-emission display devices, white light-emitting diodes, images and so on.6–8 Europium and terbium complexes have excellent luminescent properties due to the antenna effect of ligands and the f–f electron transition of Eu3+ and Tb3+ ions.9,10 However, pure rare earth complexes have serious limitations in practical applications due to their poor mechanical properties and physicochemical stabilities. In order to conquer these shortcomings, rare earth complexes are usually added into polymer matrix to form polymer-based composites, and the composites can present excellent fluorescent properties and the enhanced mechanical performance.11 Wang, et al.12 prepared color-tunable PVP/[Tb(BA)3phen + Eu(BA)3phen] luminescent composite nanofibers by single axial electrospinning, and the emitting color of the composite nanofibers could be tuned in a wide color range of red–yellow–green by adjusting the mass ratio of terbium complexes and europium complexes under the excitation of 274 nm single-wavelength ultraviolet light. Wang, et al.13 achieved a novel kind of solution processable luminescent materials based on organoclay, organic sensitizer, and Ln3+ through a simple, environmentally friendly, low-cost, and less time-consuming procedure. Various emission colors including white light with good color purity can be achieved by changing the molar ratio of Eu3+ and Tb3+ and altering the excitation wavelength. These characteristics make the composites suitable for optoelectronic devices such as thermosensors and white light LED. Magnetic nanomaterials have many applications in environmental protection, electronics, and biological images.14–16 Among them, Fe3O4 nanoparticles (NPs) have been focused on greatly because of their good biocompatibility and low toxicity. Conductive polymers combine the attractive properties of conventional polymers with unique electronic properties of metals or semiconductors.17 Polyaniline (PANI) is one of the best known conducting polymers due to its good environmental and thermal stability, fairly excellent processability and electrical conductivity.18 However, it remains a great challenge to apply electrospinning to PANI as limited by its molecular weight and solubility. To circumvent this problem, most of the researchers electrospun PANI through mixing it with other spinnable polymers.19,20
Magnetic-fluorescent bifunctional materials have been applied in drug target delivery and optical imaging.21,22 Gong, et al.23 synthesized magnetic-fluorescent bifunctional nanospheres composed of modified Fe3O4 nanoparticles as the core and rare earth complex Tb(AA)3phen as the shell. The excellent superparamagnetic and fluorescent properties render the nanospheres useful in biomedical engineering and optical imaging. Xi, et al.24 prepared [Fe3O4/PVP]//[NaYF4:Eu3+/PVP] Janus nanofibers which have potential applications in the fields of drug target delivery materials and nanodevices owing to their excellent magnetic and luminescent performance. Luminescent–electrical materials are expected to possess many potential applications in areas such as color display, electromagnetic shielding, molecular electronics and biomedicine. Lv, et al.25 prepared [PANI/PVP]//[Eu(BA)3phen/PVP] bistrand-aligned nanobundles by electrospinning. The obtained bistrand-aligned nanobundles possess excellent electrical conduction and luminescent performance due to their peculiar isolated structure. Lun, et al.26 fabricated novel [Tb(BA)3phen + Eu(BA)3phen]/PANI/PVP composite nanofibers with tunable color-electricity bifunctionality via facile one-pot electrospinning technology. The prepared [Tb(BA)3phen + Eu(BA)3phen]/PANI/PVP composite nanofibers have potential applications in many fields such as color display, molecular electronics and biomedicine. Among various multifunctional materials, those with electrical and magnetic properties are potential candidates for a wide range of applications such as nonlinear optics,27 microwave absorption28 and electromagnetic interference shielding29 and so on. Cui, et al.30 synthesized the composites consisting of Fe3O4 microspheres and polyaniline (PANI) through a two-step oxidative polymerization of aniline monomers in the presence of Fe3O4 microspheres. The obtained composites display very strong reflection loss over a wide frequency range that can be manipulated by the absorber thickness and have better microwave absorption and environmental stability.
In order to achieve a new nanostructure and have future applications in display devices, optoelectronic devices, magnetic resonance imaging and electromagnetic interference shielding, it is therefore of great interest to prepare luminescent–electrical–magnetic trifunctional nanomaterials. However, according to the ref. 5, 25, 26 and 31, the presence of PANI and Fe3O4 NPs will greatly decrease the luminescence intensity of rare earth complexes if the PANI or Fe3O4 NPs directly contact with the rare earth luminescent compounds. If strong luminescence is to be achieved, rare earth complexes must be effectively isolated from PANI and Fe3O4 NPs to ultimately reduce the impact of PANI and Fe3O4 NPs on the fluorescent property of the nanofibers or nanoribbons. At the same time, in order to achieve high conductivity, PANI must have good consecutiveness in the nanofibers or nanoribbons leading to more effective electrons transport. Whilst seeking a way to ultimately reduce the impact of PANI and Fe3O4 NPs on the fluorescent properties of the luminescent–electrical–magnetic trifunctional nanoribbons, we were inspired by the reports on Janus particles.32–34 ‘Janus’ is the name of an ancient Roman God, who has two faces peering into the past and the future. Named after this Roman God, Janus particles have two distinguishable surfaces/chemistries on their two sides. Pierre-Gilles de Gennes, winner of the Nobel Prize in Physics, made Janus particles known to the scientific community.
In this work, we adopt electrospinning technique with a specially designed parallel spinneret to fabricate luminescent–electrical–magnetic trifunctional {[Eu(BA)3phen + Tb(BA)3phen]/PMMA}//[PANI/Fe3O4/PMMA] Janus nanoribbons. Herein, one half of the Janus nanoribbons is composed of PANI, Fe3O4 NPs and PMMA, and the other half consists of Eu(BA)3phen complexes, Tb(BA)3phen complexes and PMMA. The special nanostructure successfully realize the effective isolation of PANI and Fe3O4 NPs from Eu(BA)3phen and Tb(BA)3phen complexes, thus it is expected that trifunctional Janus nanoribbons with excellent and tunable luminescence, electrical conduction and magnetic properties will be obtained. The morphology, structure, and performances of the obtained Janus nanoribbons were studied systematically, and some new meaningful results were acquired.
Experimental sections
Chemicals
Methylmethacrylate (MMA), benzoylperoxide (BPO), benzoic acid (BA), phenanthroline (phen), N,N-dimethylformamide (DMF) and trichloromethane (CHCl3) were bought from Tianjin Guangfu Technology Development Co., terbium oxide (Tb4O7, 99.99%) was purchased from Aladdin Chemistry Co., Ltd. Europium oxide (Eu2O3, 99.99%), nitric acid (HNO3), ammonia (NH3·H2O), anhydrous ethanol, aniline (ANI), FeCl3·6H2O, FeSO4·7H2O, NH4NO3, polyethylene glycol (PEG, Mw = 20
000) and (IS)-(+)-camphor-10 sulfonic acid (CSA) were purchased from Sinopharm Chemical Reagent Co., Ltd. Ammonium persulfate (APS) were purchased from Guangdong Xilong Chemical Co., Ltd. All the reagents were of analytical grade and directly used as received without further purification. Deionized water was lab-made.
Fabrication of luminescent–electrical–magnetic trifunctional Janus nanoribbons via electrospinning
The synthetic processes of the starting materials, including Fe3O4 NPs, PMMA, Tb(BA)3phen and Eu(BA)3phen complexes are described in the ESI.† Two different kinds of spinning solutions were used in this study. The spinning solution containing PMMA, Fe3O4 NPs and PANI was prepared to fabricate one side of Janus nanoribbons, which was denoted as spinning solution I. The other spinning solution comprised of PMMA, Tb(BA)3phen and Eu(BA)3phen complexes was adopted to fabricate the other side.
For preparing the spinning solution I, Fe3O4 NPs were dispersed ultrasonically into the mixed solution of 0.3000 g of DMF and 3.0000 g of CHCl3 for 20 min, and then PMMA, ANI and CSA were added into the above solution with magnetic stirring for 48 h at room temperature. APS was used as an oxidant and dispersed into the mixture of 0.6000 g of DMF and 6.0000 g of CHCl3 with magnetic stirring for 2 h at room temperature. All the above solutions were cooled down to 0 °C in the refrigerator for 1 h, and then the APS solution was dropwise added into the solution containing ANI under magnetic stirring in an ice-bath. The mixture was allowed to react for 24 h at 0 °C. A mixed solution of Tb(BA)3phen, Eu(BA)3phen, 0.5 g of PMMA, 0.9 g of DMF and 9 g of CHCl3 was prepared as the spinning solution II. The dosages of these materials were summarized in Tables 1 and 2, respectively. The Janus nanoribbons prepared by Sa1//Sb1, Sa1//Sb2, Sa1//Sb3, Sa1//Sb4, Sa1//Sb5, Sa1//Sb6, Sa1//Sb7, Sa2//Sb4, Sa3//Sb4, Sa4//Sb4, Sa5//Sb4 are respectively denoted as S1–S11.
Table 1 Compositions of the spinning solution I
Spinning solution I |
ANI/g |
CSA/g |
APS/g |
Fe3O4/g |
PMMA/g |
Sa1 |
0.15 |
0.2809 |
0.3676 |
1.5 |
0.5 |
Sa2 |
0.15 |
0.2809 |
0.3676 |
0.5 |
0.5 |
Sa3 |
0.15 |
0.2809 |
0.3676 |
2.5 |
0.5 |
Sa4 |
0.25 |
0.4682 |
0.6126 |
1.5 |
0.5 |
Sa5 |
0.35 |
0.6554 |
0.8578 |
1.5 |
0.5 |
Table 2 Compositions of the spinning solution II
Spinning solution II |
Eu(BA)3phen : Tb(BA)3phen |
Eu(BA)3phen/g |
Tb(BA)3phen/g |
PMMA/g |
Sb1 |
10 : 0 |
0.0750 |
0 |
0.5 |
Sb2 |
9 : 1 |
0.0675 |
0.0075 |
0.5 |
Sb3 |
7 : 3 |
0.0525 |
0.0225 |
0.5 |
Sb4 |
5 : 5 |
0.0375 |
0.0375 |
0.5 |
Sb5 |
3 : 7 |
0.0225 |
0.0525 |
0.5 |
Sb6 |
1 : 9 |
0.0075 |
0.0675 |
0.5 |
Sb7 |
0 : 10 |
0 |
0.075 |
0.5 |
A specially designed parallel spinneret was used in this study and the electrospinning setup was indicated in Fig. 1. Spinning solution I was injected into one of the two syringes while the spinning solution II was loaded into the other one. A flat iron net was used as a collector and put about 15 cm away from the tip of the spinneret. A positive direct current (DC) voltage of 6 kV was applied between the spinneret and the collector to generate stable, continuous PMMA-based Janus nanoribbons under the ambient temperature of 20–24 °C and atmosphere relative humidity of 24–40%.
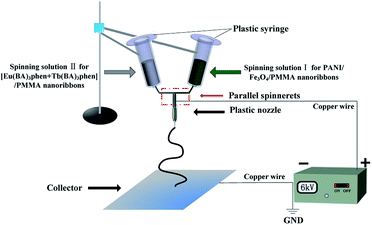 |
| Fig. 1 Schematic diagram of the specially designed parallel spinneret and electrospinning setup. | |
Preparation of [Eu(BA)3phen + Tb(BA)3phen]/PANI/Fe3O4/PMMA composite nanoribbons
For comparison, [Eu(BA)3phen + Tb(BA)3phen]/PANI/Fe3O4/PMMA composite nanoribbons (named as S12) were also fabricated by mixing the spinning solution I (Sa1) and spinning solution II (Sb4) together at the volume ratio of 1
:
1 and electrospun via traditional single-spinneret electrospinning method. This fabrication of [Eu(BA)3phen + Tb(BA)3phen]/PANI/Fe3O4/PMMA composite nanoribbons is a simple way of realizing the preparation of the luminescent–electrical–magnetic trifunctional nanoribbons-just mix the PANI, Fe3O4 NPs, [Eu(BA)3phen + Tb(BA)3phen] and PMMA together and electrospun into composite nanoribbons.
Characterizations
The samples were identified by an X-ray powder diffractometer (XRD, Bruker, D8 FOCUS) with Cu Kα radiation. The morphologies, structures and sizes of the samples were observed by a field emission scanning electron microscope (FESEM, XL-30), an energy dispersive spectrometer (EDS, Oxford ISIS 300) attached to the FESEM, a biological microscope (BM, CVM500E), and a transmission electron microscope (TEM, JEOL, JEM-2010, Tokyo, Japan), respectively. A thermogravimetric analyzer (TG, TAQ600) was used to measure the thermal stability of the Janus nanoribbons (S4) under air atmosphere, and the heating rate was kept at 10 °C min−1. The fluorescent properties of the samples were investigated by using a Hitachi fluorescence spectrophotometer F-7000. The electrical properties and the magnetic performance of samples were measured by an ECOPIA HMS-3000 Hall effect measurement system and a vibrating sample magnetometer (VSM, MPMS SQUID XL), respectively. The ultraviolet-visible spectra of samples were determined by a UV-1240 ultraviolet-visible spectrophotometer. All measurements were performed at room temperature except for thermal analysis.
Results and discussion
Phase analyses
The phase compositions of Fe3O4 NPs, {[Eu(BA)3phen + Tb(BA)3phen]/PMMA}//[PANI/Fe3O4/PMMA] Janus nanoribbons (S4) and [Eu(BA)3phen + Tb(BA)3phen]/PANI/Fe3O4/PMMA composite nanoribbons (S12) were characterized by means of XRD analysis, as shown in Fig. 2. The XRD patterns in Fig. 2a reveal that the as-prepared Fe3O4 NPs are conformed to the cubic structure of Fe3O4 (PDF 74-0748). No other characteristic diffraction peaks are detected. XRD analysis results of {[Eu(BA)3phen + Tb(BA)3phen]/PMMA}//[PANI/Fe3O4/PMMA] Janus nanoribbons (S4) and [Eu(BA)3phen + Tb(BA)3phen]/PANI/Fe3O4/PMMA composite nanoribbons (S12) demonstrate that they both contain Fe3O4 NPs, but the diffraction intensities of Fe3O4 are reduced due to the existence of amorphous Eu3+ and Tb3+ complexes, PANI and PMMA, as shown in Fig. 2b and c.
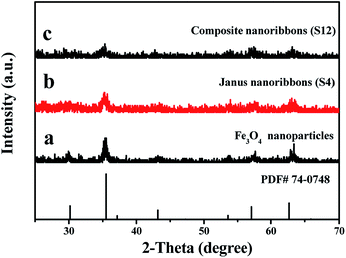 |
| Fig. 2 XRD patterns of Fe3O4 NPs (a), {[Eu(BA)3phen + Tb(BA)3phen]/PMMA}//[PANI/Fe3O4/PMMA] Janus nanoribbons (S4, b) and [Eu(BA)3phen + Tb(BA)3phen]/PANI/Fe3O4/PMMA composite nanoribbons (S12, c) with PDF standard card of Fe3O4 NPs. | |
Morphology and structure
The morphology of the as-prepared Fe3O4 NPs was observed by means of TEM, as presented in Fig. S1.† The size distribution of the spherical Fe3O4 NPs is almost uniform, and the diameter of the NPs is 8–10 nm. The morphology and structure of {[Eu(BA)3phen + Tb(BA)3phen]}//[PANI/Fe3O4/PMMA] Janus nanoribbons (S4) were characterized by the combination of BM, SEM and EDS line scan analyses. Depending on the transmission light of the BM, the inner structure of the {[Eu(BA)3phen + Tb(BA)3phen]/PMMA}//[PANI/Fe3O4/PMMA] Janus nanoribbons can be evidently observed. As revealed in Fig. 3a, an obvious asymmetry dual-sided structure can be seen in the Janus nanoribbons. A stripe of Janus nanoribbon is composed of a transparent [Eu(BA)3phen + Tb(BA)3phen]/PMMA half side and a dark-colored PANI/Fe3O4/PMMA half side. As seen from Fig. 3b and c, the thickness of the Janus nanoribbons is less than a micrometer and the width is 6.66 ± 0.01 μm under the confidence level of 95%, and some particle aggregates are faintly visible in one half side of the Janus nanoribbon.
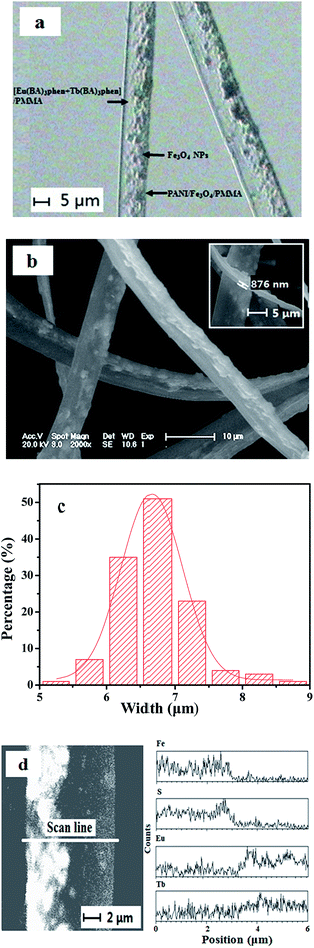 |
| Fig. 3 BM image of {[Eu(BA)3phen + Tb(BA)3phen]/PMMA}//[PANI/Fe3O4/PMMA] Janus nanoribbons (S4, a); SEM image (b) and histogram of width distribution (c) of {[Eu(BA)3phen + Tb(BA)3phen]/PMMA}//[PANI/Fe3O4/PMMA] Janus nanoribbons (S4); and EDS line scan analysis of a single stripe of {[Eu(BA)3phen + Tb(BA)3phen]/PMMA}//[PANI/Fe3O4/PMMA] Janus nanoribbon (S4, d). | |
For further proving the asymmetry dual-sided structure of {[Eu(BA)3phen + Tb(BA)3phen]/PMMA}//[PANI/Fe3O4/PMMA] Janus nanoribbons, EDS line scan analysis was performed in this work, as indicated in Fig. 3d, in which Eu, Tb, S and Fe elements respectively represent Eu(BA)3phen, Tb(BA)3phen, PANI and Fe3O4. It is found that Eu/Tb and S/Fe elements are only respectively existed in each one half side of the {[Eu(BA)3phen + Tb(BA)3phen]/PMMA}//[PANI/Fe3O4/PMMA] Janus nanoribbon. These results are well consistent with the asymmetry dual-sided structure of the Janus nanoribbon.
From the above analyses, we can safely draw a conclusion that the {[Eu(BA)3phen + Tb(BA)3phen]/PMMA}//[PANI/Fe3O4/PMMA] Janus nanoribbons have been successfully fabricated.
Thermal stability analysis
Thermogravimetric analysis of the sample (S4) was performed in air atmosphere, as shown in Fig. S2.† Janus nanoribbons lose about 4% of their initial weight when the temperature arises from 25 °C to 102 °C due to the volatilization of residual solvents and surface absorbed water. With the continuous increasing in temperature, Eu(BA)3phen and Tb(BA)3phen complexes start to decompose, and weight loss of Janus nanoribbons is 11.3% when the temperature reaches up to 260 °C. At this temperature, PMMA and PANI begin to decompose, and the decomposition process is finished at 357 °C. No weight loss is detected when temperature is above 357 °C, and the total weight loss percentage is 84.5% for the Janus nanoribbons. Inorganic materials, such as Fe3O4 has higher stability and do not easily decompose at a lower temperature range. These results reveal that the safe temperature for using the Janus nanoribbons is lower than 102 °C.
Fluorescent performance
We firstly investigate the luminescent characteristics of {[Eu(BA)3phen + Tb(BA)3phen]/PMMA}//[PANI/Fe3O4/PMMA] Janus nanoribbons. In order to perform the contrast experiments, the mass ratio of Fe3O4 to PMMA was settled as 3
:
1, and the mass percentage of PANI to PMMA was fixed at 30%. The excitation spectra of {[Eu(BA)3phen + Tb(BA)3phen]/PMMA}//[PANI/Fe3O4/PMMA] Janus nanoribbons with different mass ratios of Eu(BA)3phen to Tb(BA)3phen monitored at 616 nm (a) and 545 nm (b) are indicated in Fig. 4. The mass ratios of Eu(BA)3phen to Tb(BA)3phen are 10
:
0 (S1), 9
:
1 (S2), 7
:
3 (S3), 5
:
5 (S4), 3
:
7 (S5), 1
:
9 (S6) and 0
:
10 (S7), respectively.
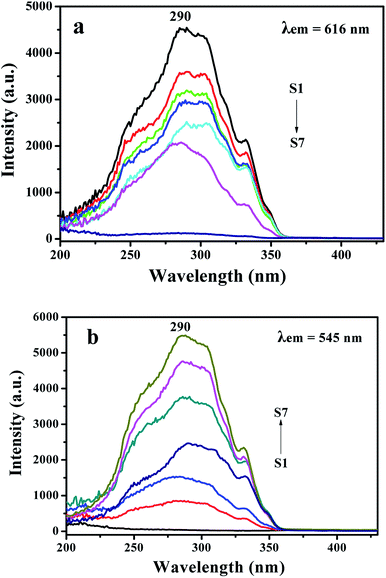 |
| Fig. 4 Excitation spectra of {[Eu(BA)3phen + Tb(BA)3phen]/PMMA}//[PANI/Fe3O4/PMMA] Janus nanoribbons with different mass ratios of Eu(BA)3phen to Tb(BA)3phen monitored at 616 nm (a) and 545 nm (b). | |
As revealed in Fig. 4a, when the monitoring wavelength is 616 nm, a broad excitation band extending from 200 to 430 nm with the maximum at 290 nm is observed, which is assigned to the π → π* electron transition of the conjugated double bonds of the ligand.35 The excitation intensity is decreased with reducing Eu(BA)3phen complexes. A broad excitation band extending from 200 to 430 nm is also observed from each sample when monitoring wavelength is 545 nm. The strongest peak at 290 nm assigned to the π → π* electron transition of the ligands could also be identified, as shown in Fig. 4b, and the excitation intensity is increased along with adding more Tb(BA)3phen complexes. Thus, one can see that both the Eu(BA)3phen and Tb(BA)3phen complexes can be simultaneously and most effectively excited by 290 nm single-wavelength ultraviolet light.
Fig. 5a shows emission spectra of {[Eu(BA)3phen + Tb(BA)3phen]/PMMA}//[PANI/Fe3O4/PMMA] Janus nanoribbons (S1–S7) with different mass ratios of Eu(BA)3phen to Tb(BA)3phen under the excitation of 290 nm ultraviolet light. Four predominant peaks in the emission spectra are observed for the {[Eu(BA)3phen + Tb(BA)3phen]/PMMA}//[PANI/Fe3O4/PMMA] Janus nanoribbons. The green, orange and red fluorescence emissions generated from the predominant peaks at 490, 545, 592 and 616 nm can be simultaneously observed. These emission peaks are attributed to the 5D4 → 7F6 (490 nm) and 5D4 → 7F5 (545 nm) energy level transitions of Tb3+ ions, and 5D0 → 7F1 (592 nm) and 5D0 → 7F2 (616 nm) energy level transitions of Eu3+ ions. With adding more Eu(BA)3phen complexes, the emission peaks attributed to Eu3+ are increased and those assigned to Tb3+ are decreased, and vice versa. In order to clearly discuss the variation trend, the intensities of the characteristic emission peaks of each sample versus different samples are plotted in Fig. 5b. The variation of the photoluminescence intensity of the Eu3+ and Tb3+ can be attributed to the energy distribution. Therefore, one can see that the emission intensity of {[Eu(BA)3phen + Tb(BA)3phen]/PMMA}//[PANI/Fe3O4/PMMA] Janus nanoribbons could be tuned by adjusting the mass ratio of europium complexes and terbium complexes under the excitation of 290 nm single-wavelength ultraviolet light.
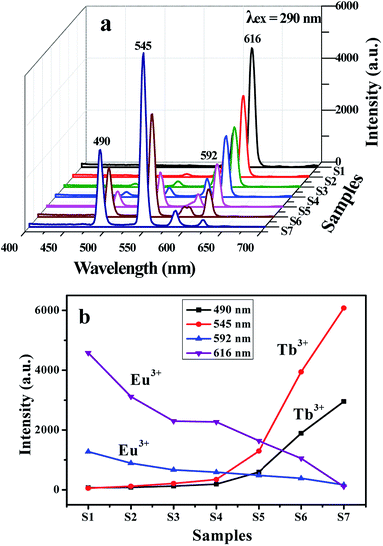 |
| Fig. 5 Emission spectra of {[Eu(BA)3phen + Tb(BA)3phen]/PMMA}//[PANI/Fe3O4/PMMA] Janus nanoribbons with different mass ratios of Eu(BA)3phen to Tb(BA)3phen (a), and the plots for intensities of the characteristic emission peaks of each sample versus different samples (b). | |
The fluorescence decay curves of Tb3+ and Eu3+ ions in {[Eu(BA)3phen + Tb(BA)3phen]/PMMA}//[PANI/Fe3O4/PMMA] Janus nanoribbons (S1–S7) with different mass ratios of Eu(BA)3phen to Tb(BA)3phen are shown in Fig. S3,† which can be used to calculate the lifetime and investigate the fluorescence dynamics of these samples. Fig. S3a and b† demonstrate the fluorescence decay curves of samples excited by 290 nm single-wavelength ultraviolet light and monitored at 545 nm and 616 nm, respectively. It is found that the curves follow the single-exponential decay:
It = I0 exp(−t/τ) |
where
It is the intensity at time
t,
I0 is the intensity at
t = 0 and
τ is the decay lifetime, and the obtained average lifetime values (
τ/ms) of Eu
3+ ions and Tb
3+ ions are shown in Fig. S3.
† It is obviously seen that the fluorescence lifetime of Tb
3+ ions is decreased slowly with increase of Tb(BA)
3phen content, and the same is true for Eu
3+ ions. The possible reasons for these results are as follows. More aggregates of Tb(BA)
3phen are formed in the polymer matrix with introducing more Tb(BA)
3phen. The exciton migration between the Tb(BA)
3phen molecules shortens the fluorescence lifetime of Tb
3+, and similarly for the Eu(BA)
3phen.
36
Generally, color can be represented by the Commission Internationale de L'Eclairage (CIE) 1931 chromaticity coordinates. Table 3 and Fig. 6 give the CIE (x, y) chromaticity coordinates and diagram of {[Eu(BA)3phen + Tb(BA)3phen]/PMMA}//[PANI/Fe3O4/PMMA] Janus nanoribbons with their corresponding photographs upon excitation by 290 nm ultraviolet light. The CIE chromaticity coordinates of samples S1 are determined to be (0.574, 0.323), corresponding to red color, and S2 are determined to be (0.520, 0.351), corresponding to orange red emission. The CIE chromaticity coordinates of samples S3, S4 and S5 are respectively determined to be (0.485, 0.377), (0.442, 0.434), (0.385, 0.465), which are placed in yellow region. Sample S6 locates at (0.323, 0.513), which represents yellow green emission. The CIE chromaticity coordinates of samples S7 are determined to be (0.261, 0.564), which belongs to green emission. It is clearly to see that the fluorescence color could be tuned by adjusting the mass ratios of Eu(BA)3phen complexes to Tb(BA)3phen complexes in a wide color range of red-yellow-green under the excitation of 290 nm single-wavelength ultraviolet light.
Table 3 Comparison among the CIE chromaticity coordinates (x, y) for the {[Eu(BA)3phen + Tb(BA)3phen]/PMMA}//[PANI/Fe3O4/PMMA] Janus nanoribbons excited by 290 nm ultraviolet light
Samples |
Concentration |
CIE coordinates (x, y) |
S1 |
100% Eu3+, 0% Tb3+ |
(0.574, 0.323) |
S2 |
90% Eu3+, 10% Tb3+ |
(0.520, 0.351) |
S3 |
70% Eu3+, 30% Tb3+ |
(0.485, 0.377) |
S4 |
50% Eu3+, 50% Tb3+ |
(0.442, 0.434) |
S5 |
30% Eu3+, 70% Tb3+ |
(0.385, 0.465) |
S6 |
10% Eu3+, 90% Tb3+ |
(0.323, 0.513) |
S7 |
0% Eu3+, 100% Tb3+ |
(0.261, 0.564) |
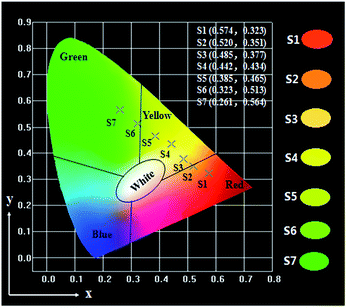 |
| Fig. 6 CIE chromaticity coordinates diagram of {[Eu(BA)3phen + Tb(BA)3phen]/PMMA}//[PANI/Fe3O4/PMMA] Janus nanoribbons with different mass ratios of Eu(BA)3phen to Tb(BA)3phen and their corresponding colors upon excitation by 290 nm ultraviolet light. | |
In order to illustrate the advantage of the nanostructure of luminescent–electrical–magnetic trifunctional Janus nanoribbons, the fluorescent properties of the {[Eu(BA)3phen + Tb(BA)3phen]/PMMA}//[PANI/Fe3O4/PMMA] Janus nanoribbons (S4) are further investigated by comparing with that of the [Eu(BA)3phen + Tb(BA)3phen]/PANI/Fe3O4/PMMA composite nanoribbons (S12) under the same components and contents of the two kinds of nanostructures. As manifested in Fig. 7, {[Eu(BA)3phen + Tb(BA)3phen]/PMMA}//[PANI/Fe3O4/PMMA] Janus nanoribbons possess excellent luminescent performance, while the [Eu(BA)3phen + Tb(BA)3phen]/PANI/Fe3O4/PMMA composite nanoribbons exhibit very weak excitation and emission spectra. The fluorescence intensity of Janus nanoribbons is ca. 40 times higher than that of composite nanoribbons. This phenomenon can be explained by the strong light absorbance of the dark-colored PANI and Fe3O4 NPs when PANI, Fe3O4 NPs, Eu(BA)3phen and Tb(BA)3phen are in direct contact with each other. As illustrated in Fig. 8, Eu(BA)3phen and Tb(BA)3phen complexes are directly distributed into the PANI/Fe3O4/PMMA nanoribbons, the exciting light has to pass through PANI and Fe3O4 NPs to reach and excite the Eu(BA)3phen and Tb(BA)3phen complexes. In this process, a large part of the exciting light has been absorbed by the PANI and Fe3O4 NPs, and thus the exciting light is much weakened before it reaches the Eu(BA)3phen and Tb(BA)3phen complexes. Similarly, the emitting light emitted by the Eu(BA)3phen and Tb(BA)3phen complexes also has to pass through PANI and Fe3O4 NPs and is absorbed by them, as a result, the exciting and emitting light are than the [Eu(BA)3phen + Tb(BA)3phen]/PANI/Fe3O4/PMMA composite nanoribbons.
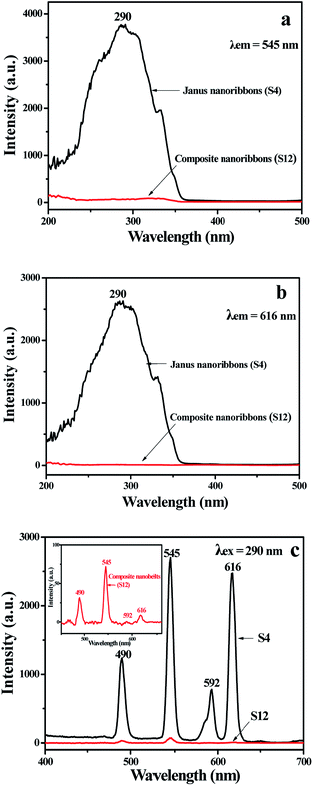 |
| Fig. 7 Excitation spectra monitored at 545 nm (a), 616 nm (b) and emission spectra (c) of {[Eu(BA)3phen + Tb(BA)3phen]/PMMA}//[PANI/Fe3O4/PMMA] Janus nanoribbons (S4) and [Eu(BA)3phen + Tb(BA)3phen]/PANI/Fe3O4/PMMA composite nanoribbons (S12). | |
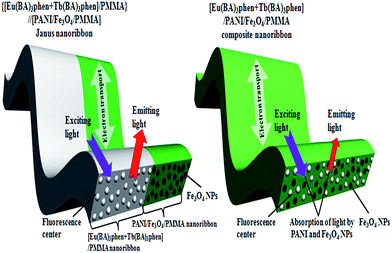 |
| Fig. 8 Schematic diagrams of the exciting light and emitting light in {[Eu(BA)3phen + Tb(BA)3phen]/PMMA}//[PANI/Fe3O4/PMMA] Janus nanoribbons (S4) and [Eu(BA)3phen + Tb(BA)3phen]/PANI/Fe3O4/PMMA composite nanoribbons (S12). | |
Besides, the fluorescent properties of the {[50% Eu(BA)3phen + 50% Tb(BA)3phen]/PMMA}//[PANI/Fe3O4/PMMA] Janus nanoribbons with different PANI contents (samples S4, S10 and S11) are also investigated. The mass ratios of Fe3O4 NPs to PMMA and Eu(BA)3phen to Tb(BA)3phen are fixed at 3
:
1 and 1
:
1, respectively. As shown in Fig. 9a, it is clearly observed that emission intensity decreases with the increase of the PANI content. From the UV-Vis absorption spectrum of PANI illustrated in Fig. 9b, it can be seen that the PANI can absorb visible light (400–760 nm) and much more easily absorb the ultraviolet light (<400 nm). The exciting light and emitting light in the Janus nanoribbons are absorbed by PANI, resulting in that the intensities of exciting light and emitting light are decreased, and the light absorption becomes stronger with introducing more PANI into the Janus nanoribbons. Moreover, as shown in Fig. 9b, a broad absorption peak at 620 nm is also observed, meaning that PANI absorbs red light much stronger. The CIE chromaticity coordinates and diagram of {[50% Eu(BA)3phen + 50% Tb(BA)3phen]/PMMA}//[PANI/Fe3O4/PMMA] Janus nanoribbons with different PANI contents under the excitation of 290 nm ultraviolet light is shown in Table 4 and Fig. 10. It demonstrates that the emitting color of the {[50% Eu(BA)3phen + 50% Tb(BA)3phen]/PMMA}//[PANI/Fe3O4/PMMA] Janus nanoribbons becomes more green with introducing more PANI, due to the stronger absorption of red light by PANI.
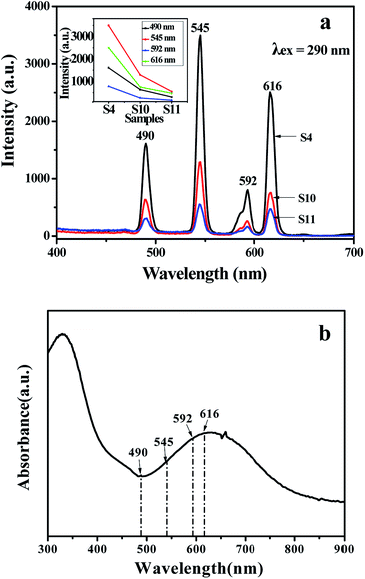 |
| Fig. 9 Comparison among emission spectra of {[50% Eu(BA)3phen + 50% Tb(BA)3phen]/PMMA}//[PANI/Fe3O4/PMMA] Janus nanoribbons containing different percentages of PANI (a) and UV-Vis absorbance spectrum of PANI (b). | |
Table 4 Comparison among the CIE chromaticity coordinates (x, y) for the {[50% Eu(BA)3phen + 50% Tb(BA)3phen]/PMMA}//[PANI/Fe3O4/PMMA] Janus nanoribbons containing different percentages of PANI
Samples |
PANI : PMMA |
CIE coordinates (x, y) |
S4 |
30% |
(0.371, 0.488) |
S10 |
50% |
(0.355, 0.447) |
S11 |
70% |
(0.317, 0.356) |
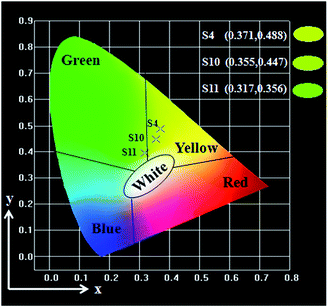 |
| Fig. 10 CIE chromaticity coordinates diagram of {[50% Eu(BA)3phen + 50% Tb(BA)3phen]/PMMA}//[PANI/Fe3O4/PMMA] Janus nanoribbons containing different percentages of PANI. | |
As illustrated in Fig. 11a, the exciting light and emitting light in the [Eu(BA)3phen + Tb(BA)3phen]/PMMA half side of a single Janus nanoribbon are little influenced by the PANI owing to the peculiar isolated nanostructure, and thus in theory, the fluorescent performance of a single Janus nanoribbon is constant with adding different amounts of PANI. However, it is difficult to characterize the fluorescent performance of a single Janus nanoribbon using current testing technology due to the restrictions on the conditions such as the sensitivity and resolution of instruments. Actually, the samples used for fluorescent measurements are composed of plenty of Janus nanoribbons. The [Eu(BA)3phen + Tb(BA)3phen]/PMMA half sides of the Janus nanoribbons in the top layers of the samples are little influenced by the [PANI/Fe3O4/PMMA] half sides, but the exciting light has to pass though the top layers to reach the Janus nanoribbons in the lower layers, which will be absorbed by the [PANI/Fe3O4/PMMA] half sides in upper layers, and the light absorption is intensified with adding more PANI, and the same is true for the emitting light. Consequently, the fluorescence intensity of the Janus nanoribbons mat is decreased with adding more PANI, as seen in Fig. 11b.
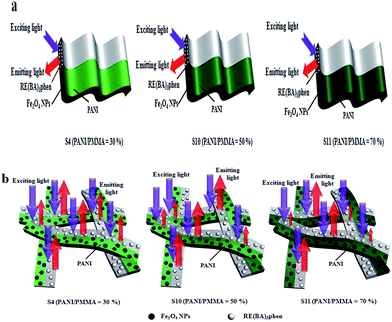 |
| Fig. 11 Schematic diagrams of the situation of the exciting light and emitting light in a single {[50% Eu(BA)3phen + 50% Tb(BA)3phen]/PMMA}//[PANI/Fe3O4/PMMA] Janus nanoribbon (a) and multiple-layered Janus nanoribbons (b) containing different percentages of PANI. | |
Meanwhile, in order to study the influence of Fe3O4 NPs on the fluorescence property, the mass ratio of Eu(BA)3phen to Tb(BA)3phen was settled as 1
:
1, and the mass percentage of PANI to PMMA was fixed at 30%. The fluorescent emission spectra (excited by 290 nm) of Janus nanoribbons containing different amounts of Fe3O4 NPs are indicated in Fig. 12a. From comparing emission spectrum (samples S8, S4 and S9) in Fig. 12a, it is found that the emission intensity of the Janus nanoribbons is decreased with the increase of the amount of Fe3O4 NPs introduced into the Janus nanoribbons. This phenomenon can be explained as the light absorption of Fe3O4 NPs which are mixed into the nanoribbons.37 From the absorbance spectrum of Fe3O4 NPs illustrated in Fig. 12b, it is seen that the Fe3O4 NPs can absorb light at ultraviolet wavelength (<400 nm) much more strongly than visible range (400–760 nm). Thus, the exciting light and emitting light are absorbed by the Fe3O4 NPs, resulting that the intensities of exciting and emitting light are decreased. Furthermore, the light absorption would become stronger with introducing more Fe3O4 NPs into the Janus nanoribbons. Fig. 13 and Table 5 are the CIE coordinates diagram and chromaticity coordinates of {[50% Eu(BA)3phen + 50% Tb(BA)3phen]/PMMA}//[PANI/Fe3O4/PMMA] Janus nanoribbons with different Fe3O4 contents under the excitation of 290 nm ultraviolet light, respectively. It demonstrates that the emitting color of the {[50% Eu(BA)3phen + 50% Tb(BA)3phen]/PMMA}//[PANI/Fe3O4/PMMA] Janus nanoribbons has changed with introducing more Fe3O4, which can be attributed to the fact that Fe3O4 has stronger light absorption at lower wavelength.
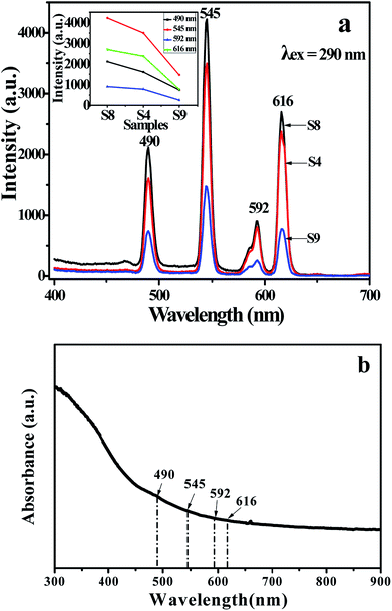 |
| Fig. 12 Comparison among emission spectra of {[50% Eu(BA)3phen + 50% Tb(BA)3phen]/PMMA}//[PANI/Fe3O4/PMMA] Janus nanoribbons containing different mass ratios of Fe3O4 (a) and UV-Vis absorbance spectrum of Fe3O4 (b). | |
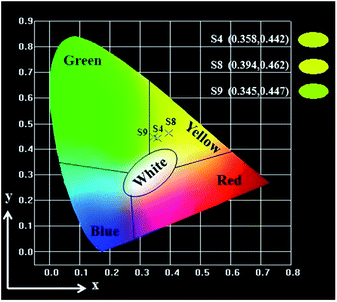 |
| Fig. 13 CIE chromaticity coordinates diagram of {[50% Eu(BA)3phen + 50% Tb(BA)3phen]/PMMA}//[PANI/Fe3O4/PMMA] Janus nanoribbons containing different mass ratios of Fe3O4 NPs. | |
Table 5 Comparison among the CIE chromaticity coordinates (x, y) for the {[50% Eu(BA)3phen + 50% Tb(BA)3phen]/PMMA}//[PANI/Fe3O4/PMMA] Janus nanoribbons containing different mass ratios of Fe3O4 NPs
Samples |
Fe3O4 : PMMA |
CIE coordinates (x, y) |
S8 |
1 : 1 |
(0.394, 0.462) |
S4 |
3 : 1 |
(0.358, 0.442) |
S9 |
5 : 1 |
(0.345, 0.447) |
Fig. 14 is schematic diagrams of the situation of the exciting light and emitting light in a single Janus nanoribbon and multiple-layered Janus nanoribbons containing different mass ratios of Fe3O4 NPs. The similar conclusions can be drawn as we have discussed in Fig. 11: the increase of the Fe3O4 NPs amounts barely impacts on the fluorescent performance of a single Janus nanoribbon, but the fluorescent intensity of multiple-layered Janus nanoribbons is decreased.
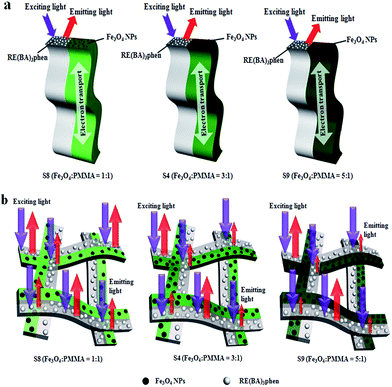 |
| Fig. 14 Schematic diagrams of the situation of the exciting light and emitting light in a single {[50% Eu(BA)3phen + 50% Tb(BA)3phen]/PMMA}//[PANI/Fe3O4/PMMA] Janus nanoribbon (a) and multiple-layered Janus nanoribbons (b) containing different mass ratios of Fe3O4 NPs. | |
Electrical conductivity analyses
The average electrical conductivity values of the samples are summarized in Table 6. The conductivities of the samples S4, S10, S11 and S12 are 7.500 × 10−4 S cm−1, 1.210 × 10−3 S cm−1, 1.430 × 10−2 S cm−1 and 5.780 × 10−4 S cm−1, respectively. Obviously, the more PANI introduced into the Janus nanoribbons, the higher electrical conductivity of the Janus nanoribbons, as PANI is consecutive in the Janus nanoribbons and probably forms the conducting network more easily, which render more efficient charge transport. The conductivity of Janus nanoribbons (S4) is slightly higher than that of composite nanoribbons (S12) under the same components and contents of the two kinds of nanostructures. The reason is probably that the insulating materials such as Fe3O4 NPs, PMMA, Eu(BA)3phen and Tb(BA)3phen are dispersed in the [Eu(BA)3phen + Tb(BA)3phen]/PANI/Fe3O4/PMMA composite nanoribbons (S12), resulting in hindering to form the continuous conducting network.
Table 6 Electrical conductivity of the samples doped with various amount of PANI
Samples |
Conductivity (S cm−1) |
Janus nanoribbons (PANI : PMMA = 30%, S4) |
7.500 × 10−4 |
Janus nanoribbons (PANI : PMMA = 50%, S10) |
1.210 × 10−3 |
Janus nanoribbons (PANI : PMMA = 70%, S11) |
1.430 × 10−2 |
Composite nanoribbons (PANI : PMMA = 30%, S12) |
5.780 × 10−4 |
Magnetic properties
The typical hysteresis loops for Fe3O4 NPs, {[Eu(BA)3phen + Tb(BA)3phen]/PMMA}//[PANI/Fe3O4/PMMA] Janus nanoribbons containing different mass ratios of Fe3O4 NPs and [Eu(BA)3phen + Tb(BA)3phen]/Fe3O4/PANI/PMMA composite nanoribbons are shown in Fig. S4,† and their saturation magnetizations are listed in Table 4. It is well known that the saturation magnetization of a magnetic composite material depends on the mass percentage of the magnetic substance in the material. It is found from Table 7 that the saturation magnetization of the {[Eu(BA)3phen + Tb(BA)3phen]/PMMA}//[PANI/Fe3O4/PMMA] Janus nanoribbons is increased from 7.03 emu g−1 to 20.87 emu g−1 with the increase of Fe3O4 NPs. It is found that the {[Eu(BA)3phen + Tb(BA)3phen]/PMMA}//[PANI/Fe3O4/PMMA] Janus nanoribbons (S4) have close magnetic properties to those of [Eu(BA)3phen + Tb(BA)3phen]/Fe3O4/PANI/PMMA composite nanoribbons (S12), because they were both prepared under the same conditions except for the spinneret. Analyses from combining the fluorescent performance with the electrical conductivity reveal that the {[Eu(BA)3phen + Tb(BA)3phen]/PMMA}//[PANI/Fe3O4/PMMA] Janus nanoribbons (S4) have the close magnetic property compared with [Eu(BA)3phen + Tb(BA)3phen]/Fe3O4/PANI/PMMA composite nanoribbons (S12), but the fluorescent intensity and the electrical conductivity of Janus nanoribbons (S4) are much higher than those of composite nanoribbons (S12), and this further demonstrates that the novel Janus nanoribbons have better.
Table 7 Saturation magnetization of Fe3O4 NPs, [Eu(BA)3phen + Tb(BA)3phen]/Fe3O4/PANI/PMMA composite nanoribbons and {[50% Eu(BA)3phen + 50% Tb(BA)3phen]/PMMA}//[PANI/Fe3O4/PMMA] Janus nanoribbons containing different mass ratios of Fe3O4 NPs
Samples |
Saturation magnetization (Ms)/(emu g−1) |
Fe3O4 nanoparticles |
68.54 |
Composite nanoribbons (S12) |
12.49 |
Janus nanoribbons (Fe3O4 : PMMA = 1 : 1, S8) |
7.03 |
Janus nanoribbons (Fe3O4 : PMMA = 3 : 1, S4) |
11.59 |
Janus nanoribbons (Fe3O4 : PMMA = 5 : 1, S9) |
20.87 |
Conclusions
In summary, {[Eu(BA)3phen + Tb(BA)3phen]/PMMA}//[PANI/Fe3O4/PMMA] Janus nanoribbons which have the tunable luminescent–electrical–magnetic performance were successfully synthesized by electrospinning technology using a specially designed parallel spinneret. One side of the Janus nanoribbons is composed of PANI, Fe3O4 NPs and PMMA, and the other side consists of Eu(BA)3phen complexes, Tb(BA)3phen complexes and PMMA. The width of the Janus nanoribbons is ca. 6.66 μm and the thickness is less than a micrometer. It is very gratifying to see that the Janus nanoribbons simultaneously possess excellent luminescent performance, electrical conduction and magnetic properties. Under the excitation of 290 nm single-wavelength ultraviolet light, the emitting color of the Janus nanoribbons can be tuned in a wide color range of red-yellow-green by adjusting the contents of europium complexes, terbium complexes, PANI or Fe3O4 NPs. The electrical conductivity and magnetic property of the Janus nanoribbons can be respectively tuned by adjusting different amounts of PANI and Fe3O4 NPs. Besides, the design concept and preparation method for the Janus nanoribbons are of universal significance for the fabrication of other tunable electricity, magnetism and color multifunctional Janus nanoribbons. The new electricity, magnetism and color-tunable {[Eu(BA)3phen + Tb(BA)3phen]/PMMA}//[PANI/Fe3O4/PMMA] trifunctional Janus nanoribbons have potential applications in many fields such as full-color display, electromagnetic shielding, molecular electronics, microwave absorption and nanodevices.
Acknowledgements
This work was financially supported by the National Natural Science Foundation of China (51573023, 50972020, 51072026), Specialized Research Fund for the Doctoral Program of Higher Education (20102216110002, 20112216120003), the Science and Technology Development Planning Project of Jilin Province (Grant No. 20130101001JC, 20070402).
Notes and references
- G. Mallesham, C. Swetha, S. Niveditha, M. E. Mohanty, N. J. Babu, A. Kumar, K. Bhanuprakash and V. J. Rao, Phosphine oxide functionalized pyrenes as efficient blue light emitting multifunctional materials for organic light emitting diodes, J. Mater. Chem. C, 2015, 3, 1208–1224 RSC.
- P. W. Menezes, A. Indra, N. R. Sahraie, A. Bergmann, P. Strasser and M. Driess, Cobalt–Manganese-Based Spinels as Multifunctional Materials that Unify Catalytic Water Oxidation and Oxygen Reduction Reactions, ChemSusChem, 2015, 8, 164–171 CrossRef CAS PubMed.
- C. Cha, S. R. Shin, N. Annabi, M. R. Dokmeci and A. Khademhosseini, Carbon-Based Nanomaterials: Multifunctional Materials for Biomedical Engineering, ACS Nano, 2013, 7, 2891–2897 CrossRef CAS PubMed.
- D. C. Guo, Z. Y. Sun, L. D. Xu, Y. Gao, M. M. Dai, S. H. Wang, Q. Chang, C. Wang and D. G. Ma, Water-soluble luminescent–electrical–magnetic trifunctional composite nanofibers prepared via electrospinning technique, Mater. Lett., 2015, 159, 159–162 CrossRef CAS.
- N. Lv, Q. L. Ma, X. T. Dong, J. X. Wang, W. S. Yu and G. X. Liu, Parallel spinnerets electrospinning fabrication of novel flexible luminescent–electrical–magnetic trifunctional bistrand-aligned nanobundles, Chem. Eng. J., 2014, 243, 500–508 CrossRef CAS.
- R. Q. Li, L. L. Li, W. W. Zi, J. J. Zhang, L. Liu, L. C. Zou and S. C. Gan, Morphology control and multicolor-tunable luminescence of YOF:Ln3+ (Ln = Eu, Tb, Dy, Tm) nano-/microcrystals, New J. Chem., 2015, 39, 115–121 RSC.
- Y. Zhang, D. L. Geng, M. M. Shang, X. Zhang, X. J. Li, Z. Y. Cheng, H. Z. Lian and J. Lin, Soft-chemical synthesis and tunable luminescence of Tb3+, Tm3+/Dy3+-doped SrY2O4 phosphors for field emission displays, Dalton Trans., 2013, 42, 4799–4808 RSC.
- T. T. Jiang, J. L. Song, H. J. Wang, X. C. Ye, H. Wang, W. T. Zhang, M. Y. Yang, R. X. Xia, L. X. Zhu and X. L. Xu, Aqueous synthesis of color tunable Cu doped Zn-In-S/ZnS nanoparticles in the whole visible region for cellular imaging, J. Mater. Chem. B, 2015, 3, 2402–2410 RSC.
- S. Quici, A. Casoni, F. Foschi, L. Armelao, G. Bottaro, R. Seraglia, C. Bolzati, N. Salvarese, D. Carpanese and A. Rosato, Folic Acid-Conjugated Europium Complexes as Luminescent Probes for Selective Targeting of Cancer Cells, J. Med. Chem., 2015, 58, 2003–2014 CrossRef CAS PubMed.
- F. Morgner, A. Lecointre, L. J. Charbonniere and H. G. Lohmannsroben, Detecting free hemoglobin in blood plasma and serum with luminescent terbium complexes, Phys. Chem. Chem. Phys., 2015, 17, 1740–1745 RSC.
- X. J. Zhou, Q. L. Ma, X. T. Dong, J. X. Wang, W. S. Yu and G. X. Liu, Flexible Janus nanofibers: a feasible route to realize simultaneously tuned magnetism and enhanced color-tunable luminescence bifunctionality, RSC Adv., 2015, 5, 35948–35957 RSC.
- L. Y. Wang, X. T. Dong, G. Q. Gai and L. Zhao, Electrospinning fabrication of color-tunable flexible composite nanofibers containing lanthanide ions, J. Mater. Sci.: Mater. Electron., 2014, 25, 1633–1638 CrossRef CAS.
- T. R. Wang, P. Li and H. R. Li, Color-Tunable Luminescence of Organoclay-Based Hybrid Materials Showing Potential Applications in White LED and Thermosensors, ACS Appl. Mater. Interfaces, 2014, 6, 12915–12921 CAS.
- S. C. N. Tang and I. M. C. Lo, Magnetic nanoparticles: Essential factors for sustainable environmental applications, Water Res., 2013, 47, 2613–2632 CrossRef CAS PubMed.
- H. Teymourian, A. Salimi and S. Khezrian, Fe3O4 magnetic nanoparticles/reduced graphene oxide nanosheets as a novel electrochemical and bioelectrochemical sensing platform, Biosens. Bioelectron., 2013, 49, 1–8 CrossRef CAS PubMed.
- T. H. Shin, Y. Choi, S. Kim and J. Cheon, Recent advances in magnetic nanoparticle-based multi-modal imaging, Chem. Soc. Rev., 2015, 44, 4501–4516 RSC.
- Y. Shi, L. L. Peng, Y. Ding, Y. Zhao and G. H. Yu, Nanostructured conductive polymers for advanced energy storage, Chem. Soc. Rev., 2015, 44, 6684–6696 RSC.
- S. Yalçınkaya, C. Demirbilek and C. Dinç, Preparation and characterization of polypyrrole/dextran sulphate composite: its electrochemical and thermal behaviors, Polym. Bull., 2015, 72, 2843–2855 CrossRef.
- Y. X. Zhang and G. C. Rutledge, Electrical Conductivity of Electrospun Polyaniline and Polyaniline-Blend Fibers and Mats, Macromolecules, 2012, 45, 4238–4246 CrossRef CAS.
- J. B. Ballengee and P. N. Pintauro, Morphological Control of Electrospun Nafion Nanofiber Mats, J. Electrochem. Soc., 2011, 158, B568–B572 CrossRef CAS.
- H. X. Peng, B. Cui, G. M. Li, Y. S. Wang, N. N. Li, Z. G. Chang and Y. Y. Wang, A multifunctional β-CD-modified Fe3O4@ZnO: Er3+,Yb3+ nanocarrier for antitumor drug delivery and microwave-triggered drug release, Mater. Sci. Eng., C, 2015, 46, 253–263 CrossRef CAS PubMed.
- Y. J. Lu, Y. Zheng, S. S. You, F. Wang, Z. Gao, J. Shen, W. T. Yang and M. Z. Yin, Bifunctional Magnetic-Fluorescent Nanoparticles: Synthesis, Characterization, and Cell Imaging, ACS Appl. Mater. Interfaces, 2015, 7, 5226–5232 CAS.
- Y. Gong, J. W. Dai, H. Li, X. Wang, H. R. Xiong, Q. Y. Zhang, P. H. Li, C. F. Yi, Z. S. Xu, H. B. Xu and P. K. Chu, Magnetic, Fluorescent, and Thermo-Responsive Poly(MMA-NIPAM-Tb(AA)3Phen)/Fe3O4 Multifunctional Nanospheres Prepared by Emulsifier-Free Emulsion Polymerization, J. Biomater. Appl., 2015, 159, 159–163 Search PubMed.
- X. Xi, Q. L. Ma, M. Yang, X. T. Dong, J. X. Wang, W. S. Yu and G. X. Liu, Janus nanofiber: a new strategy to achieve simultaneous enhanced magnetic-photoluminescent bifunction, J. Mater. Sci.: Mater. Electron., 2014, 25, 4024–4032 CrossRef CAS.
- N. Lv, X. T. Dong, Q. L. Ma, J. X. Wang, W. S. Yu and G. X. Liu, Parallel spinnerets electrospinning construct and properties of electrical-luminescent bifunctional bistrand-aligned nanobundles, J. Mater. Sci., 2014, 49, 2171–2179 CrossRef CAS.
- K. Lun, Q. L. Ma, X. T. Dong, W. S. Yu, J. X. Wang and G. X. Liu, A single flexible nanofiber to obtain simultaneous tunable color-electricity bifunctionality, J. Mater. Sci.: Mater. Electron., 2014, 25, 5395–5402 CrossRef CAS.
- F. L. Zhang, Q. Zhao, C. W. Lan, X. He, W. H. Zhang, J. Zhou and K. P. Qiu, Magnetically coupled electromagnetically induced transparency analogy of dielectric metamaterial, Appl. Phys. Lett., 2014, 104, 131907 CrossRef.
- Y. Yong, Y. Yang, X. Wen and D. Jun, Microwave electromagnetic and absorption properties of magnetite hollow nanostructures, J. Appl. Phys., 2014, 115, 17A521 CrossRef.
- A. P. Singh, M. Mishra, D. P. Hashim, T. N. Narayanan, M. G. Hahm, P. Kumar, J. Dwivedi, G. Kedawat, A. Gupta, B. P. Singh, A. Chandra, R. Vajtai, S. K. Dhawan, P. M. Ajayan and B. K. Gupta, Probing the engineered sandwich network of vertically aligned carbon nanotube–reduced graphene oxide composites for high performance
electromagnetic interference shielding applications, Carbon, 2015, 85, 79–88 CrossRef CAS.
- C. K. Cui, Y. C. Du, T. H. Li, X. Y. Zheng, X. H. Wang, X. J. Han and P. Xu, Synthesis of Electromagnetic Functionalized Fe3O4 Microspheres/Polyaniline Composites by Two-Step Oxidative Polymerization, J. Phys. Chem. B, 2012, 116, 9523–9531 CrossRef CAS PubMed.
- S. J. Sheng, Q. L. Ma, X. T. Dong, N. Lv, J. X. Wang, W. S. Yu and G. X. Liu, A single nanobelt to achieve simultaneous photoluminescence–electricity–magnetism trifunction, J. Mater. Sci.: Mater. Electron., 2014, 25, 2279–2286 CrossRef CAS.
- A. Walther and A. H. E. Muller, Janus particles, Soft Matter, 2008, 4, 663–668 RSC.
- J. Yan, S. C. Bae and S. Granick, Colloidal Superstructures Programmed into Magnetic Janus Particles, Adv. Mater., 2015, 27, 874–879 CrossRef CAS PubMed.
- T. Wang, S. R. Chen, F. Jin, J. H. Cai, L. Y. Cui, Y. M. Zheng, J. X. Wang, Y. L. Song and L. Jiang, Droplet-assisted fabrication of colloidal crystals from flower-shaped porphyrin Janus particles, Chem. Commun., 2015, 51, 1367–1370 RSC.
- S. Meshkova, The Dependence of the Luminescence Intensity of Lanthanide Complexes with β-Diketones on the Ligand Form, J. Fluoresc., 2000, 10, 333–337 CrossRef CAS.
- Q. L. Ma, J. X. Wang, X. T. Dong, W. S. Yu and G. X. Liu, Magnetic-upconversion luminescent bifunctional flexible coaxial nanoribbon and Janus nanoribbon: One-pot electrospinning preparation, structure and enhanced upconversion luminescent characteristics, Chem. Eng. J., 2015, 260, 222–230 CrossRef CAS.
- Q. L. Ma, W. S. Yu, X. T. Dong, J. X. Wang and G. X. Liu, Janus nanobelts: fabrication, structure and enhanced magnetic-fluorescent bifunctional performance, Nanoscale, 2014, 6, 2945–2952 RSC.
Footnote |
† Electronic supplementary information (ESI) available. See DOI: 10.1039/c6ra04192b |
|
This journal is © The Royal Society of Chemistry 2016 |