DOI:
10.1039/C6RA02963A
(Paper)
RSC Adv., 2016,
6, 37731-37739
Construction of bimodal silsesquioxane-based porous materials from triphenylphosphine or triphenylphosphine oxide and their size-selective absorption for dye molecules†
Received
1st February 2016
, Accepted 8th April 2016
First published on 11th April 2016
Abstract
Two novel hybrid porous polymers were easily prepared by the Friedel–Crafts reaction of octavinylsilsesquioxane with triphenylphosphine and triphenylphosphine oxide, respectively. They possessed unique bimodal pores with uniform micropores and mesopores centered at 1.5 nm and 3.7 nm, respectively, high surface areas up to 1105 m2 g−1, high thermal stability and an excellent size-selective adsorption for dyes. These hybrid porous polymers are very promising in dye separation, water purification and treatment.
Introduction
Porous materials have attracted much attention during the last few decades because they show great potential applications in the fields of gas storage and separation,1–4 catalysis,5,6 optoelectronics,7,8 chemosensors,9–11 drug delivery12–15 and environment.16–18 Recently, porous materials formed by covalent bonded linkage are of great interest for their characteristic advantages: large specific surface areas, low skeletal density, high thermal and chemical stability, etc.19–21 Rational selection of suitable rigid building blocks with different geometries and advantageous functionalities will be helpful in the construction of porous materials with novel topology structures and properties.
Cubic octavinylsilsesquioxane (OVS) (Scheme 1), a versatile precursor, has been extensively explored as a starting material for nanohybrid synthesis.22–29 Recently, many hybrid porous polymers were prepared via the topology combination from cubic OVS with planar molecules,30 tetrahedral molecules,31,32 cubic molecules33–36 and polymer.37 By far, there was no report on hybrid porous polymers by the combination of trigonal pyramidal triphenylphosphine with cubic OVS.
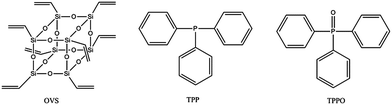 |
| Scheme 1 Structure of octavinylsilsesquioxane (OVS), triphenylphosphine (TPP) and triphenylphosphine oxide (TPPO). | |
Triphenylphosphine (denoted as TPP) is an important ligand for noble metals, and widely used in the cross-coupling reactions in modern synthesis chemistry.38–40 TPP and its derivative, triphenylphosphine oxide (denoted as TPPO), are usually rigid trigonal pyramidal molecules, and possess different steric crowding around the phosphorus atom, tended to take a more tetrahedral geometry (Scheme 1), which make them become two ideal building blocks for constructing functional porous polymers. Some phosphorous-containing porous polymers have been prepared by different methods, such as the Friedel–Crafts reaction,41 the Yamamoto reaction,42 and the Sonogashira–Hagihara coupling reaction.43 From a practical point of view, the use of commercial TPP or TPPO is highly desirable to prepare some novel cost-effective phosphorus-containing porous polymers in a large-scale via the Friedel–Crafts reaction.
In this paper, triphenylphosphine (TPP) and triphenylphosphine oxide (TPPO) were chosen to react with cubic octavinylsilsesquioxane (OVS) to prepare two novel parallel series of hybrid phosphorus-containing porous polymers (denoted as HP-TPPs and HP-TPPOs) via the Friedel–Crafts reaction, respectively. These hybrid porous polymers possessed high surface areas, unique bimodal pores with uniform micropores and mesopores centered at 1.5 nm and 3.7 nm, respectively, and an excellent size-selective adsorption for dyes. They displayed an outstanding adsorption ability for rhodamine B (RB) with maximum equilibrium adsorption capacities of 828.6 mg g−1 and could rapidly remove more than 90% RB within 3 minutes when the initial concentration of the RB solution was 50 ppm.
Experimental section
Materials
Unless otherwise noted, all reagents were obtained from commercial suppliers and used without further purification. Octavinylsilsesquioxane (OVS) was synthesized from the previous reports.44,45 Triphenylphosphine oxide (TPPO) was prepared according to the previous report.46 1,2-Dichloroethane was dried by distillation over calcium hydride under reflux prior to use.
Methods
FTIR spectra were measured within a 4000 cm−1 to 400 cm−1 regions on a Bruker TENSOR-27 infrared spectrophotometer (KBr pellet). Solution-state 1H NMR spectra were measured with a Bruker Avance 300 spectrometer in CDCl3 with tetramethylsilane as the internal standard. Solid-state 13C cross polarization (CP) magic angle spinning (MAS) NMR, 29Si MAS NMR and 31P high power decoupling (HPDEC) MAS NMR spectra were carried out on a Bruker Avance-500 NMR spectrometer operating under a magnetic field strength of 9.4 T. A chemagnetics of 5 mm triple-resonance MAS probe was used to acquire 13C, 29Si and 31P NMR spectra. 29Si MAS NMR spectra with high power proton decoupling were recorded using a π/2 pulse length of 5 μs, a recycle delay of 120 s and a spinning rate of 5 kHz. The 13C chemical shifts were referenced externally via the resonance of tetramethylsilane (TMS) of 0 ppm. The 31P chemical shifts were referenced externally via the resonance of NH4H2PO4 at an isotropic chemical shift of 1.058 ppm. Elemental analysis was performed by using an Elementarvario EL III elemental analyzer. Nitrogen sorption isotherm measurements were performed by using a Micro Meritics surface area and pore size analyzer. The samples were degassed for 12 h at 150 °C prior to measurement. A sample of ca. 100 mg and a UHP-grade nitrogen (99.999%) gas source were chosen in the nitrogen sorption measurements at 77 K and collected on a Quantachrome Quadrasorb apparatus. BET surface areas were evaluated over a P/P0 range from 0.02 to 0.20. Nonlocal density functional theory (NL-DFT) pore size distributions were confirmed using the carbon/slit-cylindrical pore mode of the Quadrawin software. Thermal gravimetric analysis (TGA) was conducted on a Mettler-Toledo TGA/DSC system under a N2 atmosphere at a heating rate of 10 °C min−1 from room temperature to 800 °C. Powder X-ray diffraction (PXRD) were measured with a Rigaku D/MAX 2550 diffractometer with Cu-Kα radiation, 40 kV, 200 mA with a 2θ range of 5–80° (scanning rate of 10° min−1) at room temperature. The field-emission scanning electron microscopy (FE-SEM) experiments were recorded by using a HITACHI S4800 spectrometer. The high-resolution transmission electron microscopy (HR-TEM) experiments were characterized by using a JEM 2100 electron microscope (JEOL, Japan) with an acceleration voltage of 200 kV. CO2 adsorption capacity was measured at 273.0 K at 1.0 bar with a TriStar II 3020 V1.04. Before measurement, the samples were degassed at 150 °C under vacuum for about 15 h. UV-vis absorption spectra of organic dyes were collected on an a TU-1901 double beam UV-vis spectrophotometer.
Synthesis of OVS
OVS was synthesized from the previous reports.44,45 675 mL of acetone, 66.8 (0.45 mol) g of vinyltrimethoxysilane, 112.5 mL of concentrated hydrochloric acid and 129.6 mL of deionized water were charged into a 1 L flask equipped with a magnetic stirrer, refluxed at 40 °C for 48 h. A brown mixture solution was obtained. The white power could be obtained by filtering, washing with ethanol and recrystallizing from the mixed solutions of dichloromethane and acetone to afford 11.9 g of OVS with 33% yield. FTIR (KBr, cm−1): 3043 (ν C–H), 1605 (ν C
C), 1410, 1276 (δ C–H), 1112, 463 (ν Si–O–Si), 584 (δ Si–O–Si), 778 (ν Si–C) (Fig. S1†); 1H NMR (CDCl3): δ 5.89–6.16 (m, H2C
CH–, 24H) (Fig. S2†).
Synthesis of TPPO
TPPO was prepared according to the previous report.46 2.65 g (10.1 mmol) triphenylphosphine (TPP), 50 mL tetrahydrofuran (THF) and 30% hydrogen peroxide (30 mL) were charged into a 150 mL flask with a magnetic stirrer and stirred over night at room temperature. The mixture was evaporated and purified to give a white solid (2.75 g). Yield: 98%. FTIR (KBr, cm−1): 3070, 3027 (ν C–H), 1581 (ν C
C), 1438, 1263 (δ C–H), 1189, 1116 (ν P–O), 724 (ν P–C) (Fig. S3†); 1H NMR (CDCl3): δ 7.70–7.64 (m, 6H), 7.58–7.52 (m, 3H), 7.49–7.43 (m, 6H) (Fig. S4†).
Synthesis of hybrid porous polymers
A typical procedure for HP-TPP-1 was given in detail as follows. OVS (0.95 g, 1.5 mmol), TPP (0.35 g, 1.3 mmol), anhydrous aluminum chloride (0.40 g, 3.0 mmol) and 1,2-dichloroethane (40 mL) were charged in an oven-dried flask. The mixture was stirred at room temperature for 0.5 h and then under reflux for 24 h. After cooling to room temperature, the mixture was filtered and washed with THF, methanol, acetone, chloroform, sequentially, in order to remove any unreacted monomers or residuary catalyst. The product was further purified under the Soxhlet extractor with THF for 24 h and methanol for 24 h, respectively, and then dried in vacuum at 70 °C for 48 h to obtain a yellow power (0.89 g, 69%). HP-TPP-2, HP-TPP-3, HP-TPPO-1, HP-TPPO-2 and HP-TPPO-3 were synthesized as same as the procedure of HP-TPP-1. Elemental analysis of HP-TPPs and HP-TPPOs were shown in Table S1.†
Dye adsorption experiments
The batch mode adsorption studies were done by taking dye molecules as probes, including rhodamine B (RB), methylene blue (MB), and methyl orange (MO) (Scheme 2). Dye solutions were prepared by deionized water with known amounts of RB, MB and MO. The adsorption isotherm was obtained by the addition of 10 mg HP-TPP-3 or HP-TPPO-3 into 30 mL dye solutions of different initial concentrations. The mixture was stirred for several hours (typically 24 h) at room temperature to reach adsorption equilibrium. Subsequently, the adsorbents were removed by filtering. And the collected solutions were reserved to be analyzed by UV-vis spectrophotometer. The wavelengths for the detection are 554, 665, and 466 nm for RB, MB, and MO, respectively.
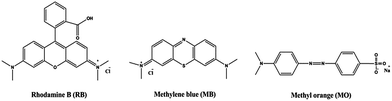 |
| Scheme 2 Structure of rhodamine B (RB), methylene blue (MB), methyl orange (MO). | |
The RB adsorption rate test was done by adding 10 mg HP-TPP-3 or HP-TPPO-3 into 15 mL aqueous RB solution of same initial concentration (50 ppm). The mixture was stirred and remained in different time at room temperature. The next procedure was the same as the batch mode.
Results and discussion
Synthesis and characterization of hybrid porous polymers
Octavinylsilsesquioxane (OVS) reacted with TPP and TPPO to prepare two novel parallel series of hybrid porous polymers, i.e. HP-TPPs and HP-TPPOs, respectively, as shown in Scheme 3. The resulting products were yellow powders, had a low density, and were not soluble in any common organic solvents. These hybrid porous polymers were first characterized by FTIR spectroscopy. We took HP-TPPOs as samples to confirm the success of the Friedel–Crafts reaction. Compared with the FTIR pattern of OVS, the intensities of the peaks at about 3023, 1609, 1412, and 1277 cm−1 decreased to different degrees (Fig. 1), which indicated that the cross-linking reaction occurred in varying degrees in HP-TPPOs. The decrease of C
C (1609 cm−1) vibrations and unsaturation C–H (3023 cm−1) vibrations of the OVS demonstrated the successful synthesis of the hybrid porous polymers. And the band of approximate 2963 cm−1 confirmed the presence of methylene groups (Si–CH2–) in HP-TPPOs, which also indicated that the Friedel–Crafts reaction was successful. Compared with the typical Si–O–Si stretching vibration for OVS at ca. 1111 cm−1 (Fig. 1), those for the porous networks became broader, indicating the presence of cross-linking networks in the hybrid porous polymers.30,32,33 The symmetric stretching vibrations of P
O at 1187 cm−1 (Fig. 1) was not observed in HP-TPPOs, which could be attributed to the overlap of P
O and Si–O–Si. The FTIR spectra of HP-TPPs were shown in Fig. S5.†
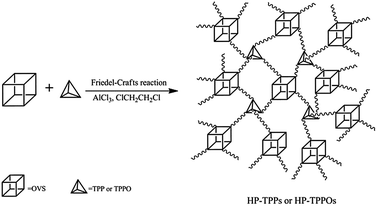 |
| Scheme 3 Synthesis of hybrid porous polymers. | |
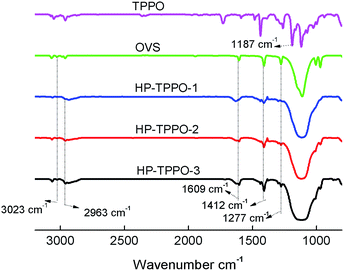 |
| Fig. 1 FTIR spectra of HP-TPPOs, TPPO and OVS. | |
Solid-state 13C CP/MAS, 29Si MAS and 31P HPDEC/MAS NMR were also used to further confirm the structures of these hybrid crosslinked porous polymers. Considering their similar spectra, HP-TPPO-3 was selected as a representative sample to analyze in HP-TPPO series. As shown in Fig. 2, the signals of the carbon atoms of the bridging phenyl units and of the unreacted double bonds from TPPO and OVS were observed in the range of 127–151 ppm. The resonance peaks at 19.6 ppm and 23.4 ppm were mainly ascribed to the methylene carbon atoms (Si–CH2–CH2–Ar–P
O and Si–CH2–CH2–Ar–P
O), which confirmed the formation of single C–C bonds derived from the vinyl units after the Friedel–Crafts reaction.30,32 The signal at δ = 46 ppm might be assigned to the carbon atom from methanol. Similar result was also observed in the solid-state 13C CP/MAS NMR spectrum of HP-TPP-3 (Fig. S6†).
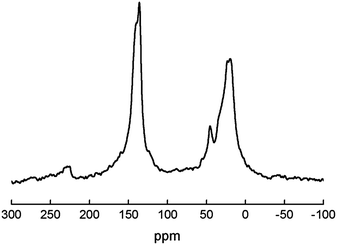 |
| Fig. 2 Solid-state 13C CP/MAS NMR spectrum of HP-TPPO-3. | |
Fig. 3 showed the solid-state 29Si MAS NMR spectrum of HP-TPPO-3. There were two main signals at ca. −68.4 and −80.2 ppm in Fig. 3, which could be assigned to the silicon atoms of the T3 units (Tn: CSi(OSi)n(OH)3−n) from the Si–CH2–CH2– units formed after the Friedel–Crafts reaction and the unreacted Si–CH
CH2 units, respectively. The signal of Si–CH2–CH2– units further confirmed the success of the Friedel–Crafts reaction. Similar phenomenon was also observed in the solid-state 29Si MAS NMR spectrum of HP-TPP-3 (Fig. S7†).
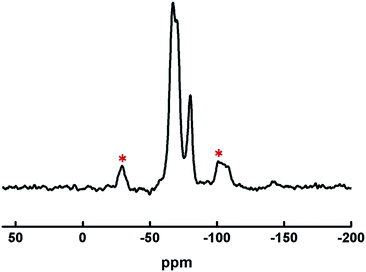 |
| Fig. 3 Solid-state 29Si MAS NMR spectrum of HP-TPPO-3 (‘*’ meant satellite peaks). | |
Fig. 4 showed the solid-state 31P HPDEC/MAS NMR spectrum of HP-TPPO-3. A single broad peak in the range of 50–100 ppm was assigned to phosphorus in phosphine oxide units in HP-TPPO-3. The solid-state 31P HPDEC/MAS NMR spectrum of HP-TPP-3 (Fig. S8†) exhibited two main signals. The signal at 22.6 ppm corresponded to phosphorus in triphenylphosphine units in HP-TPP-3; another signal at 65.1 ppm was assigned to phosphorus in phosphine oxide units in HP-TPP-3, which originated from aerobic oxidation of PIII.41,47
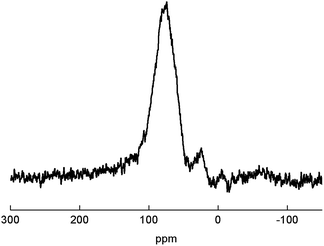 |
| Fig. 4 Solid-state 31P HPDEC/MAS NMR spectrum of HP-TPPO-3. | |
The Brunauer–Emmet–Teller (BET) specific surface area (SBET) and pore structures of hybrid porous polymers were characterized by nitrogen adsorption and desorption isotherms experiments at 77 K. All of the samples showed the type IV shape of nitrogen adsorption isotherms (Fig. S9†), a sharp uptake at low relative pressures and a gradually increasing uptake at higher relative pressures with a hysteresis, indicating that the synthesized hybrid porous polymers contained both micropores and mesopores.32,33 The detailed porosity data of these hybrid porous polymers were compiled in Table 1. The SBET of HP-TPP-1, HP-TPP-2, HP-TPP-3, HP-TPPO-1, HP-TPPO-2 and HP-TPPO-3 was calculated as 966, 1018, 1105, 887, 920 and 976 m2 g−1, respectively. The respective micropore surface area (Smicro) was calculated to be 375, 277, 260, 189, 172 and 78 m2 g−1 by using the t-plot method. With the increasing mole ratio of OVS to TPP or TPPO, the SBET of the hybrid porous polymers increased and approached a maximum SBET of 1015 and 976 m2 g−1 when the molar ratio was 1.5
:
0.8 (i.e. HP-TPP-3 and HP-TPPO-3), respectively; however, the Smicro of the hybrid polymers decreased.
Table 1 Porosity data of HP-TPPs and HP-TPPOs
Sample |
Molar ratio of OVS to TPP/TPPO |
SBET/m2 g−1 |
Smicro/m2 g−1 |
Vtotal/cm3 g−1 |
Vmicro/cm3 g−1 |
Vmicro/Vtotal |
HP-TPP-1 |
1.5 : 1.3 |
966 |
375 |
0.67 |
0.18 |
0.28 |
HP-TPP-2 |
1.5 : 1.0 |
1018 |
277 |
0.75 |
0.12 |
0.16 |
HP-TPP-3 |
1.5 : 0.8 |
1105 |
260 |
0.82 |
0.11 |
0.13 |
HP-TPPO-1 |
1.5 : 1.3 |
887 |
189 |
0.58 |
0.08 |
0.14 |
HP-TPPO-2 |
1.5 : 1.0 |
920 |
172 |
0.66 |
0.07 |
0.11 |
HP-TPPO-3 |
1.5 : 0.8 |
976 |
78 |
0.72 |
0.02 |
0.03 |
Pore-size distributions (PSD) of the hybrid porous polymers were evaluated from the nonlocal density functional theory (NL-DFT). The results were in accordance with the patterns of the N2 sorption isotherms and suggested the presence of micropores and mesopores within the hybrid porous polymers. As shown in Fig. 5, the polymers showed similar PSD curves with unique bimodal pores, which indicated the uniformity of micro-mesopores in the networks. All the samples showed uniform micropores with an average diameter located at around 1.5 nm and uniform mesopores with an average diameter centered at about 3.7 nm. And the ratio of the micropore volume (Vmicro) to Vtotal for HP-TPP-1 to HP-TPPO-3 was 0.28, 0.16, 0.13, 0.14, 0.11 and 0.03, respectively (Table 1). According to the IUPAC classification, these hybrid porous polymers could be regarded as mesoporous polymers because of the low Vmicro/Vtotal ratio.
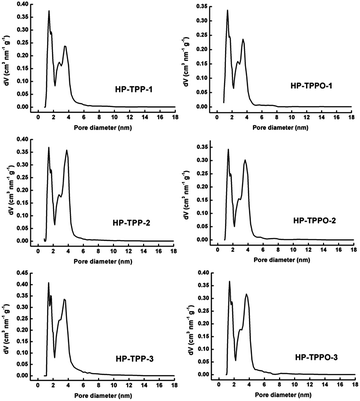 |
| Fig. 5 NL-DFT pore-size distributions of HP-TPPs and HP-TPPOs. | |
It was interesting that SBET and Vtotal of the hybrid porous polymers increased monotonically with the increasing molar ratio of OVS to TPP or TPPO; while Smicro, Vmicro and Vmicro/Vtotal decreased. The increasing molar ratio of OVS to TPP or TPPO meant the increasing reactive sites of the phenyl groups in the TPP or TPPO and the increasing locally crosslinked density, which favored mesopores.32 Different from previous phosphorus-containing microporous polymers,42,43 two kind of novel hybrid polymers with unique bimodal pores, i.e. uniform micropores and mesopores centered at 1.5 nm and 3.7 nm, respectively, were easily prepared through the topology combination of TPP or TPPO with OVS via the Friedel–Crafts reaction.
Thermal stability and morphology
TGA was used to evaluate the thermal stability of the hybrid porous polymers. These porous polymers possessed good thermal stability and exhibited high thermal decomposition temperature. The decomposition temperatures (Td at 5 wt%) of HP-TPPOs and HP-TPPs were above 500 °C (Fig. 6), which were significantly higher than those of OVS, TPP and TPPO. This result could be predominantly ascribed to the formation of highly crosslinked network after the Friedel–Crafts reaction;30,33 in addition, the inorganic silica-like cages and phosphorus elements containing in the hybrid polymers also contributed to the thermal staibility.32,37,48 The crystallographic order of these hybrid porous polymers was investigated by powder X-ray diffraction (PXRD) analysis. Although OVS, TPP and TPPO were highly crystalline, the resulted hybrid porous polymers HP-TPP-3 and HP-TPPO-3 were amorphous and showed a broad diffraction peak at 2θ ≈ 22° (Fig. S10†), which were evidently associated with the Si–O–Si linkages.32,33 The particle size and morphology could be observed by FE-SEM (Fig. S11†). HP-TPPO-3 was selected as a representative sample to analyze. The sample aggregated into interlinking irregular shapes looked like cloud with nanostructure appearance and a wide range of diameters from 500 nm to several micrometers.30,32,33 The worm-like texture showed in translucent HR-TEM images (Fig. S12†) indicated a porous and not dense structure of the materials with relatively uniform pore diameters but no evidence of long-range ordering. The results were consistent with other OVS-based porous materials.30–33
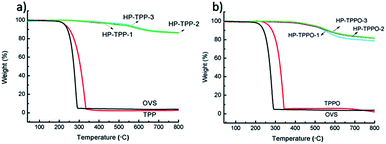 |
| Fig. 6 (a) TGA curves of HP-TPPs, OVS and TPP in N2; (b) TGA curves of HP-TPPOs, OVS and TPPO in N2. | |
CO2 storage of HP-TPP-3 and HP-TPPO-3
Gas storage is one of the most important potential applications for porous materials,49,50 especially in storing CO2. HP-TPP-3 and HP-TPPO-3 possessed the same molar content of phosphorus and the highest SBET in HP-TPPs and HP-TPPOs, respectively; therefore, they were selected to compare their CO2 capture properties. The CO2 capture capacities for HP-TPP-3 and HP-TPPO-3 were 3.97 wt% (0.90 mmol g−1) and 4.63 wt% (1.05 mmol g−1) at 273.0 K/101 kPa, respectively (Fig. 7). The result showed that the introduction of P
O bond into hybrid network indeed improved CO2 adsorption, agreeing with previous reports.42,43
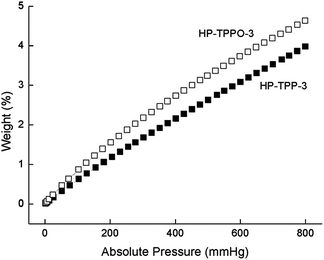 |
| Fig. 7 CO2 adsorption isotherms of HP-TPP-3 and HP-TPPO-3 at 273 K. | |
Dye adsorption of HP-TPP-3 and HP-TPPO-3
Water contamination remains a serious environmental and public problem, especially by toxic organic dyes. Rapid and convenient removal of organic dyes from wastewater by various techniques has been studied in the past, among which adsorption is the simplest and widely adopted technology.51,52 The same reason with CO2 capture, HP-TPP-3 and HP-TPPO-3 were also selected to compare their adsorption efficiency of different kinds of dyes. A UV-vis spectrophotometer was employed to trace the adsorption behaviors of HP-TPP-3 and HP-TPPO-3 according to changes in the intensities of the maximum absorption wavelengths of rhodamine B (RB), methylene blue (MB), and methyl orange (MO) at 554, 665, and 466 nm, respectively.
The specific amount adsorbed onto adsorbents was calculated based on the following formula:53
|
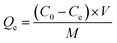 | (1) |
where
Qe (mg g
−1) is the equilibrium adsorption capacities,
C0 (mg L
−1) is the initial concentration,
Ce (mg L
−1) is the equilibrium concentration,
V (L) is the volume of the aqueous solution and
M (g) is the mass of the adsorbent used.
The molecular size of RB, MB and MO molecule, estimated by Newtonian ball and spring model, was in the order of RB > MB > MO, listed in Table 2.53 It was interesting to observe that they showed distinctly different equilibrium adsorption capacities (Qe) for three dye molecules; RB possessed the maximum Qe of 828.6 mg g−1 and MO possessed the minimum Qe of 25.9 mg g−1. This result suggested that these porous polymers possessed an excellent size-selective adsorption for dyes. As mentioned above, the hybrid porous polymers showed unique bimodal pores with micropores centered at around 1.5 nm and mesopores centered at about 3.7 nm, respectively. Adsorption is a complex process. Adsorption capacity of dyes in porous materials is a comprehensive result of various factors, among which pore structure plays an important role in the adsorption process. For these hybrid materials in this work, most pores should be open and interconnecting; and micropore sizes centered at 1.5 nm, which was larger than that of MB and MO. Although mass transfer of MB and MO was more efficient in the porous materials, they could rapidly enter into pores; at the same time, they also easily leave from pores and led to low adsorption capacities in the porous polymers because of small spatial hindrance. However, the diameter of RB was larger than 1.5 nm, the mass transportation in the small-size pores was seriously inhibited; thus, vast RB molecules could block the orifice of micropores or bind in the mesopores.53,54 Therefore, the adsorption capacities of RB was much larger than those of MB and MO. This unique bimodal pores structure made these porous polymers prefer to absorb bulky dye molecules. This was supported by the adsorption equilibrium experiment, which showed that the adsorption equilibrium time of MB and MO nearly needed 24 hours; however, RB almost achieve adsorption equilibrium within 30 minutes. It was interesting that MO showed lower adsorption capacity compared to MB although they both possessed smaller sizes. This was probably related with the nature of porous materials and dyes. As we know, these hybrid porous materials were prepared from cubic silsesquioxane and triphenylphosphine or triphenylphosphine oxide; therefore, the content of O and P were higher, which possessed partially negative charges. MB was a kind of cationic dye, which had stronger electrostatic attraction interactions with the hybrid porous polymers. However, MO was a kind of anionic dye, which had electrostatic repulsion interactions with the hybrid porous polymers. So the adsorption capacity of MO was lower than that of MB.
Table 2 Adsorption capacities for three dye molecules onto adsorbents
Dye |
Molecular size (nm) |
Molecular weight (g mol−1) |
Qe (mg g−1) |
HP-TPP-3 |
HP-TPPO-3 |
Rhodamine B (RB) |
1.59 × 1.18 × 0.56 |
478 |
674.6 |
828.6 |
Methylene blue (MB) |
1.26 × 0.77 × 0.65 |
320 |
154.0 |
145.0 |
Methyl orange (MO) |
1.31 × 0.55 × 0.18 |
327 |
25.9 |
64.6 |
In view of the maximum Qe of RB, we further investigate its adsorption behaviors. Three well-known isotherm equations, Langmuir isotherm,55–58 Freundlich isotherm58–60 and Dubinin–Radushkevich isotherm,61,62 were applied to evaluate the data of RB adsorption (the three isotherm equations see ESI†).
The adsorption isotherms of RB (Fig. 8) demonstrated a positive relationship between the adsorption capacity of the hybrid porous polymers and the equilibrium concentration. The detailed fitting isotherm parameters were listed in Table 3. For HP-TPP-3, the correlation coefficient (RL2, 0.9909) of the linearized Langmuir equation was higher than those of Freundlich equation (RF2, 0.9267) and Dubinin–Radushkevich equation (RD2, 0.8955), indicated that the adsorption behaviors of RB onto HP-TPP-3 could better fit to Langmuir model. However, the adsorption behaviors of RB onto HP-TPPO-3 followed Dubinin–Radushkevich isotherm more satisfactorily than other two isotherms. From the Langmuir plot (Fig. S18†), Qmax for HP-TPP-3 was calculated to be 847.46 mg g−1 and higher than the experimental Qe of 674.6 mg g−1, probably due to the adsorption of solvent onto adsorbents. From the Dubinin–Radushkevich plot (Fig. S20†), Qmax for HP-TPPO-3 was calculated to be 758.26 mg g−1, and lower than the experimental Qe of 828.6 mg g−1. This experimental Qe was significantly higher than those for other porous materials, such as active carbon,63 mesoporous carbons,53 et al. (Table S2†). And the E values of RB adsorption onto HP-TPPO-3 or HP-TPP-3 were lower 8 kJ mol−1; thus, the adsorption behavior of RB belonged to physical adsorption.58
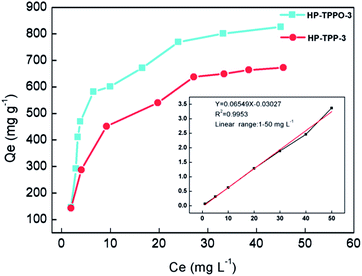 |
| Fig. 8 Rhodamine B adsorption isotherms of HP-TPP-3 and HP-TPPO-3. The inset shows the low-limit of detection with a linear fit in the linear concentration range. | |
Table 3 Parameters of isotherms for rhodamine B adsorption onto adsorbents
Model |
Parameters |
Adsorbent |
HP-TPP-3 |
HP-TPPO-3 |
Langmuir isotherm |
Qmax (mg g−1) |
847.46 |
1627.34 |
KL (L mg−1) |
0.111 |
0.065 |
RL2 |
0.9909 |
0.8099 |
Freundlich isotherm |
n |
2.191 |
2.331 |
KF (mg1−1/n L1/n g−1) |
133.903 |
196.549 |
RF2 |
0.9267 |
0.7354 |
Dubinin–Radushkevich isotherm |
KD (mol2 J−2) |
3.141 × 10−6 |
3.629 × 10−6 |
E (kJ mol−1) |
0.399 |
0.371 |
RD2 |
0.8955 |
0.9803 |
Qmax (mg g−1) |
590.41 |
758.26 |
As shown in Fig. 9, it showed the absorption spectra at different time intervals of RB aqueous solutions after they were treated with HP-TPP-3 and HP-TPPO-3, respectively. It could be observed that the characteristic bands of RB became increasingly weaker with the increasing treatment time, and both of the bands were completely removed after being treated for 3 h; more than 90% of the dyes were rapidly removed by HP-TPP-3 within 6 minutes and by HP-TPPO-3 within 3 minutes, respectively. The inset figures showed the corresponding camera images of the dye solutions over time. The colors of the dyes became increasingly lighter and finally faded after 3 h.
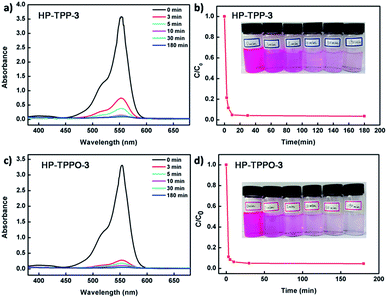 |
| Fig. 9 Dye adsorption properties of HP-TPP-3 and HP-TPPO-3. (a and c) UV-vis adsorption spectra of the RB solutions after being treated with HP-TPP-3 and HP-TPPO-3 at different time intervals. The initial concentration of the RB solution is 50 ppm; (b and d) the RB adsorption rate of HP-TPP-3 and HP-TPPO-3. The insets show the corresponding camera images. | |
In order to further examine the adsorption process of RB, pseudo-first-order (eqn (2)),64 pseudo-second-order (eqn (3))65 and intraparticle diffusion (eqn (4))66 models were used to test the experimental data.
|
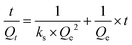 | (2) |
|
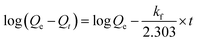 | (3) |
where
Qt (mg g
−1) and
Qe (mg g
−1) are amount of dye adsorbed at each time and equilibrium, respectively. And
kf (min
−1),
ks (g mg
−1 min
−1), and
ki (mg g
−1 min
−0.5) are the rate constants of pseudo-first-order, pseudo-second-order, and intraparticle diffusion models, respectively. The fitting results of RB onto adsorbents HP-TPP-3 and HP-TPPO-3 were exhibited in Fig. S22–S24.
† It could be easily observed that linear relation of pseudo-second-order kinetic fitting curve was much better than those of pseudo-first-order kinetic fitting curve and intraparticle diffusion kinetic fitting curve. The kinetic parameters were all listed in
Table 4. It suggested that pseudo-second-order model was more suitable in explaining this adsorption process. In addition, the values of
Qe calculated by pseudo-first-order kinetic model largely deviated the experimental values, which further indicated that pseudo-second-order model was the optimal candidate in explaining RB onto adsorbents HP-TPP-3 and HP-TPPO-3.
Table 4 Kinetic parameters for the adsorption of rhodamine B adsorption on adsorbents
Model |
Parameters |
Adsorbent |
HP-TPP-3 |
HP-TPPO-3 |
Pseudo-first-order |
Qe,exp (mg g−1) |
79.29 |
94.37 |
kf (min−1) |
0.1243 |
0.1402 |
Rf2 |
0.9753 |
0.5982 |
Qe,cal (mg g−1) |
15.30 |
12.85 |
Pseudo-second-order |
ks (g mg−1 min−1) |
0.0242 |
0.0542 |
Qe,cal (mg g−1) |
79.55 |
94.43 |
Rs2 |
1.0000 |
1.0000 |
Intraparticle diffusion |
RI2 |
0.1955 |
0.1165 |
It was interesting that the equilibrium adsorption capacities and adsorption rates for RB onto HP-TPPO-3 were higher than those for RB onto HP-TPP-3 by the introduction of the P
O bond (Fig. 8 and 9). As we all know, the cubic OVS and trigonal pyramidal TPP molecule are nonpolar compounds,67–69 which make the HP-TPP-3 hydrophobic. However, the introduction of P
O bond caused HP-TPPO-3 to possess certain hydrophilicity compared with HP-TPP-3. What's more, P
O bond is a kind of hydrogen bond acceptor,70 making the interaction force between HP-TPPO-3 and water molecules stronger. This two factors mentioned above, make HP-TPPO-3 easier to form a uniform dispersion in RB aqueous solution. Thus, the RB in water could fully contact with the hybrid porous polymers and easily enter into the pores of the hybrid polymers. In addition, the oxygen-rich units (TPPO) make HP-TPPO-3 possess partially negative charges, which allow additionally strong electrostatic interactions with cationic dye molecules (RB).71,72 This also explained the adsorption behaviors of HP-TPPO-3 could better fit Dubinin–Radushkevich model. The last but not least, the adsorption amount of bulky dye molecules (such as RB) onto porous materials increased with the increasing mesopore volumes.73,74 Although SBET of HP-TPP-3 was higher than that of HP-TPPO-3, HP-TPPO-3 had more mesopores than HP-TPP-3, which made the Qe of HP-TPPO-3 larger than HP-TPP-3.
After these porous materials adsorbed dyes, they could be regenerated by Soxhlet extraction with methanol and used again. In addition, dye molecules could also be recycled after methanol was removed by vacuum.
Conclusions
Phosphorus-centered trigonal pyramidal molecule triphenylphosphine and triphenylphosphine oxide, and cubic octavinylsilsesquioxane, were simultaneously used as building blocks to easily synthesize two novel parallel series of hybrid porous polymers via the Friedel–Crafts reaction. The resulting materials possessed unique bimodal pores with uniform micropore centered at 1.5 nm and mesopore centered at 3.7 nm, high surface areas up to 1105 m2 g−1, and high thermal stability. More importantly, they possessed an excellent size-selective adsorption performance for different molecular size dyes and could rapidly remove bulky rhodamine B. Simple synthetic procedure, commercial raw materials, and inexpensive catalyst make these hybrid porous polymers very promising to be industrialized and be of great use in the field of dyes separation and wastewater treatment.
Acknowledgements
This research was supported by the National Natural Science Foundation of China (NSFC) (grant number 21274081 and 21574075).
References
- R. J. White, V. Budarin, R. Luque, J. H. Clark and D. J. Macquarrie, Chem. Soc. Rev., 2009, 38, 3401–3418 RSC
. - J. Germain, J. M. Frechet and F. Svec, Small, 2009, 5, 1098–1111 CrossRef CAS PubMed
. - H. Furukawa and O. M. Yaghi, J. Am. Chem. Soc., 2009, 131, 8875–8883 CrossRef CAS PubMed
. - S.-Y. Ding and W. Wang, Chem. Soc. Rev., 2013, 42, 548–568 RSC
. - J. S. Seo, D. Whang, H. Lee, S. Im Jun, J. Oh, Y. J. Jeon and K. Kim, Nature, 2000, 404, 982–986 CrossRef CAS PubMed
. - C. H. Christensen, K. Johannsen, I. Schmidt and C. H. Christensen, J. Am. Chem. Soc., 2003, 125, 13370–13371 CrossRef CAS PubMed
. - A. Patra and U. Scherf, Chem.–Eur. J., 2012, 18, 10074–10080 CrossRef CAS PubMed
. - V. S.-Y. Lin, K. Motesharei, K.-P. S. Dancil, M. J. Sailor and M. R. Ghadiri, Science, 1997, 278, 840–843 CrossRef CAS PubMed
. - P. Wu, J. Wang, Y. Li, C. He, Z. Xie and C. Duan, Adv. Funct. Mater., 2011, 21, 2788–2794 CrossRef CAS
. - P. Wu, J. Wang, C. He, X. Zhang, Y. Wang, T. Liu and C. Duan, Adv. Funct. Mater., 2012, 22, 1698–1703 CrossRef CAS
. - T. Fei, K. Jiang, S. Liu and T. Zhang, RSC Adv., 2014, 4, 21429–21434 RSC
. - D. A. Edwards, J. Hanes, G. Caponetti, J. Hrkach, A. Ben-Jebria, M. L. Eskew, J. Mintzes, D. Deaver, N. Lotan and R. Langer, Science, 1997, 276, 1868–1872 CrossRef CAS PubMed
. - C.-Y. Lai, B. G. Trewyn, D. M. Jeftinija, K. Jeftinija, S. Xu, S. Jeftinija and V. S.-Y. Lin, J. Am. Chem. Soc., 2003, 125, 4451–4459 CrossRef CAS PubMed
. - P. Horcajada, C. Serre, M. Vallet-Regí, M. Sebban, F. Taulelle and G. Férey, Angew. Chem., 2006, 118, 6120–6124 CrossRef
. - P. Horcajada, T. Chalati, C. Serre, B. Gillet, C. Sebrie, T. Baati, J. F. Eubank, D. Heurtaux, P. Clayette and C. Kreuz, Nat. Mater., 2010, 9, 172–178 CrossRef CAS PubMed
. - M. Hartmann, S. Kullmann and H. Keller, J. Mater. Chem., 2010, 20, 9002–9017 RSC
. - Q. Liu, L. Wang, A. Xiao, J. Gao, W. Ding, H. Yu, J. Huo and M. Ericson, J. Hazard. Mater., 2010, 181, 586–592 CrossRef CAS PubMed
. - F.-H. Wang, W. Jiang, Y. Fang and C.-W. Cheng, Chem. Eng. J., 2015, 259, 827–836 CrossRef CAS
. - D. Wu, F. Xu, B. Sun, R. Fu, H. He and K. Matyjaszewski, Chem. Rev., 2012, 112, 3959–4015 CrossRef CAS PubMed
. - A. Thomas, Angew. Chem., Int. Ed., 2010, 49, 8328–8344 CrossRef CAS PubMed
. - A. I. Cooper, Adv. Mater., 2009, 21, 1291–1295 CrossRef CAS
. - G. Cheng, N. R. Vautravers, R. E. Morris and D. J. Cole-Hamilton, Org. Biomol. Chem., 2008, 6, 4662–4667 CAS
. - A. Sellinger and R. M. Laine, Chem. Mater., 1996, 8, 1592–1593 CrossRef CAS
. - M. Asuncion and R. M. Laine, J. Am. Chem. Soc., 2010, 132, 3723–3736 CrossRef CAS PubMed
. - H. Liu, M. Puchberger and U. Schubert, Chem.–Eur. J., 2011, 17, 5019–5023 CrossRef CAS PubMed
. - M. F. Roll, J. W. Kampf and R. M. Laine, Macromolecules, 2011, 44, 3425–3435 CrossRef CAS
. - J. C. Furgal, J. H. Jung, T. Goodson III and R. M. Laine, J. Am. Chem. Soc., 2013, 135, 12259–12269 CrossRef CAS PubMed
. - L. Li and H. Liu, RSC Adv., 2014, 4, 46710–46717 RSC
. - Y. Liu, W. Yang and H. Liu, Chem.–Eur. J., 2015, 21, 4731–4738 CrossRef CAS PubMed
. - Y. Wu, D. Wang, L. Li, W. Yang, S. Feng and H. Liu, J. Mater. Chem. A, 2014, 2, 2160–2167 CAS
. - D. Wang, W. Yang, L. Li, X. Zhao, S. Feng and H. Liu, J. Mater. Chem. A, 2013, 1, 13549–13558 CAS
. - W. Yang, D. Wang, L. Li and H. Liu, Eur. J. Inorg. Chem., 2014, 2014, 2976–2982 CrossRef CAS
. - D. Wang, L. Xue, L. Li, B. Deng, S. Feng, H. Liu and X. Zhao, Macromol. Rapid Commun., 2013, 34, 861–866 CrossRef CAS PubMed
. - I. Nischang, O. Bruggemann and I. Teasdale, Angew. Chem., Int. Ed., 2011, 50, 4592–4596 CrossRef CAS PubMed
. - C. Zhang, F. Babonneau, C. Bonhomme, R. M. Laine, C. L. Soles, H. A. Hristov and A. F. Yee, J. Am. Chem. Soc., 1998, 120, 8380–8391 CrossRef CAS
. - W. Chaikittisilp, M. Kubo, T. Moteki, A. Sugawara-Narutaki, A. Shimojima and T. Okubo, J. Am. Chem. Soc., 2011, 133, 13832–13835 CrossRef CAS PubMed
. - Y. Wu, L. Li, W. Yang, S. Feng and H. Liu, RSC Adv., 2015, 5, 12987–12993 RSC
. - S. U. Son, Y. Jang, K. Y. Yoon, E. Kang and T. Hyeon, Nano Lett., 2004, 4, 1147–1151 CrossRef CAS
. - V. Farina and B. Krishnan, J. Am. Chem. Soc., 1991, 113, 9585–9595 CrossRef CAS
. - T. Ishiyama, M. Murata and N. Miyaura, J. Org. Chem., 1995, 60, 7508–7510 CrossRef CAS
. - B. Li, Z. Guan, W. Wang, X. Yang, J. Hu, B. Tan and T. Li, Adv. Mater., 2012, 24, 3390–3395 CrossRef CAS PubMed
. - Q. Zhang, Y. Yang and S. Zhang, Chem.–Eur. J., 2013, 19, 10024–10029 CrossRef CAS PubMed
. - S. Qiao, W. Huang, Z. Du, X. Chen, F.-K. Shieh and R. Yang, New J. Chem., 2015, 39, 136–141 RSC
. - J. F. Brown, L. H. Vogt and P. I. Prescott, J. Am. Chem. Soc., 1964, 86, 1120–1125 CrossRef CAS
. - D. Chen, S. Yi, W. Wu, Y. Zhong, J. Liao, C. Huang and W. Shi, Polymer, 2010, 51, 3867–3878 CrossRef CAS
. - C. Liu, Y. Li, Y. Li, C. Yang, H. Wu, J. Qin and Y. Cao, Chem. Mater., 2013, 25, 3320–3327 CrossRef CAS
. - W. He, F. Zhang and H. Li, Chem. Sci., 2011, 2, 961–966 RSC
. - K. C. Högström, H. Lundgren, S. Wilken, T. G. Zavalis, M. Behm, K. Edström, P. Jacobsson, P. Johansson and G. Lindbergh, J. Power Sources, 2014, 256, 430–439 CrossRef
. - F. Vilela, K. Zhang and M. Antonietti, Energy Environ. Sci., 2012, 5, 7819–7832 CAS
. - X. Zou, H. Ren and G. Zhu, Chem. Commun., 2013, 49, 3925–3936 RSC
. - C. Namasivayam and D. Sangeetha, J. Hazard. Mater., 2006, 135, 449–452 CrossRef CAS PubMed
. - A. Walcarius and L. Mercier, J. Mater. Chem., 2010, 20, 4478–4511 RSC
. - X. Zhuang, Y. Wan, C. Feng, Y. Shen and D. Zhao, Chem. Mater., 2009, 21, 706–716 CrossRef CAS
. - C. Zhang, P.-C. Zhu, L. Tan, J.-M. Liu, B. Tan, X.-L. Yang and H.-B. Xu, Macromolecules, 2015, 48, 8509–8514 CrossRef CAS
. - I. Langmuir, J. Am. Chem. Soc., 1918, 40, 1361–1403 CrossRef CAS
. - W. Shi, S. Tao, Y. Yu, Y. Wang and W. Ma, J. Mater. Chem., 2011, 21, 15567–15574 RSC
. - L. Zhou, C. Gao and W. Xu, ACS Appl. Mater. Interfaces, 2010, 2, 1483–1491 CAS
. - A. M. Isloor, S. S. Shenvi, A. F. Ismail, S. J. Shilton and D. A. Al-Ahmad, Ind. Eng. Chem. Res., 2015, 54, 4965–4975 CrossRef
. - M. D. LeVan and T. Vermeulen, J. Phys. Chem., 1981, 85, 3247–3250 CrossRef CAS
. - S. Tao, C. Wang, W. Ma, S. Wu and C. Meng, Microporous Mesoporous Mater., 2012, 147, 295–301 CrossRef
. - A. Gil and P. Grange, Colloids Surf., A, 1996, 113, 39–50 CrossRef CAS
. - C. Nguyen and D. Do, Carbon, 2001, 39, 1327–1336 CrossRef CAS
. - K. Kadirvelu, C. Karthika, N. Vennilamani and S. Pattabhi, Chemosphere, 2005, 60, 1009–1017 CrossRef CAS PubMed
. - Y. S. Ho and G. McKay, Water Res., 1999, 33, 578–584 CrossRef CAS
. - Y. S. Ho and G. Mckay, Trans. Inst. Chem. Eng., 1998, 76, 313–318 CAS
. - W. J. Weber and J. C. Morris, J. Sanit. Eng. Div., Am. Soc. Civ. Eng., 1963, 89, 31–60 Search PubMed
. - I. Nischang, O. Brüggemann and I. Teasdale, Angew. Chem., Int. Ed., 2011, 50, 4592–4596 CrossRef CAS PubMed
. - K. Tanaka and Y. Chujo, J. Mater. Chem., 2012, 22, 1733–1746 RSC
. - I. Romm, E. Guryanova, N. Rozanelskya and K. Kocheskov, Tetrahedron Lett., 1977, 18, 33–36 CrossRef
. - C. M. Whitaker, K. L. Kott and R. J. McMahon, J. Org. Chem., 1995, 60, 3499–3508 CrossRef CAS
. - C. e. N. e. R. Rao, A. e. K. Sood, K. e. S. Subrahmanyam and A. Govindaraj, Angew. Chem., Int. Ed., 2009, 48, 7752–7777 CrossRef CAS PubMed
. - X. Huang, Z. Yin, S. Wu, X. Qi, Q. He, Q. Zhang, Q. Yan, F. Boey and H. Zhang, Small, 2011, 7, 1876–1902 CrossRef CAS PubMed
. - C.-T. Hsieh and H. Teng, Carbon, 2000, 38, 863–869 CrossRef CAS
. - G. Walker and L. Weatherley, Chem. Eng. J., 2001, 83, 201–206 CrossRef CAS
.
Footnote |
† Electronic supplementary information (ESI) available. See DOI: 10.1039/c6ra02963a |
|
This journal is © The Royal Society of Chemistry 2016 |
Click here to see how this site uses Cookies. View our privacy policy here.