DOI:
10.1039/C6RA02697D
(Paper)
RSC Adv., 2016,
6, 30320-30329
Rheological properties and crystallization behavior of comb-like graft poly(L-lactide): influences of graft length and graft density†
Received
29th January 2016
, Accepted 8th March 2016
First published on 11th March 2016
Abstract
Three series of narrowly dispersed comb-like graft poly(L-lactide) (PLLA) with controlled graft length and graft density have been facilely synthesized and characterized. The structural information was determined by means of 1H NMR, GPC, and viscometry, demonstrating the well-defined grafted structure. The effects of grafting parameters on rheological properties, thermal and crystallization behaviors were investigated systematically to illustrate the structure–property relationships. By the analysis of the rheological behavior, the increase of graft length and graft density can both lead to an elevated value of zero-viscosity (η0), enhanced complex modulus (G*) and increased complex viscosity (η*). Comparatively, graft density contributes more to the improvement of rheological properties than graft length does. For thermal properties, DSC results show that the glass transition temperature (Tg), the melting temperature (Tm) and the crystallization enthalpy (ΔHm) of graft PLLA increase with the chain length and decrease with the graft density. Furthermore, spherulite morphologies and growth rates were studied by polarized microscopy (POM), and the observation revealed a remarkable improvement of radius growth rate of the spherulites (G) when graft density increased. The results indicate that G depends more on the molecular weight of each arm (Mn,arm) than the total Mn.
Introduction
Poly(L-lactide) (PLLA) is a biodegradable and biocompatible polymer. It combines both convenient accessibility from renewable resources and well-understood synthesis.1 Therefore, PLLA has been studied intensely in recent years, due to its high mechanical strength, and excellent shaping and molding properties. Despite these obvious advantages, however, PLLA shows some disadvantages such as low glass transition temperature (Tg), slow crystallization, poor stability, as well as its brittle nature. To tailor the properties of PLLA for more suitable applications, many methods are performed as copolymerization, cross-linking, chain-extension, branching, stereocomplexation, blending etc. One of the promising methods is to introduce branched or grafted structure into PLLA.2 By varying the molecular architecture, PLA could be useful for more applications such as surfactants,3 compatibilizer,4 and also as carriers for drug delivery.5
Up to now, different architectures such as star-like,6 comb-like,7 and other branched PLA8 have been successfully synthesized, with the investigation on their properties originated from their molecular architectures. As noted, the branching strongly affects the chain motion in polymer melt and determines rheological properties of polymer. The branched polymer were found to exhibit stronger shear thinning, higher Newtonian viscosity, enhanced storage modulus compared with the linear counterpart.9 For the crystallization behavior, some of the studies elucidated that the crystallization kinetics of the branched PLLA are inferred to be faster than the linear analog.2a,10 C. B. Park et al. systematically explored the effect of chain branching on PLLA's crystallization kinetics.10b The branched PLLA with up to 0.7 wt% of the given chain extender performed enhanced the degree of crystallization. Mihai et al. also reported that addition chain extender improved the crystallization and the foaming behavior of PLLA samples.11 However, Hideto Tsuji et al. reported that the crystallinity (Xc) and radius growth rate of the spherulites (G) for the branched PLLA were lower than its linear counterpart.12 Since the branched PLA have been reported with different crystallization behavior from its linear one, the effect of the branching parameters on PLA's properties is still not well-understood.
Comparatively, the case of the comb-like PLA is more complicated, as both the main chain architecture and the side chain parameters are related to PLA's performance. Zhao et al. prepared comb-like PLA using poly(2-hydroxythyl methacrylate) (PHEMA) as the backbone.13 Since there are numbers of hydroxyl groups on the PHEMA, it is hard to control the graft density of PLA side chains. Hillmyer and co-workers were able to synthesize a graft PLA with poly(1,5-cyclooctadiene-co-5-norbornene-2-methanol) (PCN) as the backbone.14 However, both the PCN and the resulting PLA showed broad polymer disperse index (PDIPCN = 1.69, PDIPLA = 1.88). The comb-like PLA is always with broadened molecular weight distributions and very uncontrollable topological structures. Due to the difficulty of preparing well-defined structure, relatively few literatures reported the effect of branching on the rheological property and crystallization behavior of graft PLLA. A systematic relationship between the main chain structure, the grafting parameters and the properties of the PLLA is unclear.
In this regard, we have recently reported the synthesis of comb-like PLLA with different backbone structures by using ‘graft-from’ technique.15 This technique requires a preliminary synthesis of linear or star-shaped polybutadiene (PB) backbone containing multiple initiator sites. By comparing the properties of linear PLLA with linear-comb and star-comb PLLA, the influence of main chain structure was well illustrated in the previous paper. However, the effect of side chain parameters was not considered in that initial study. This work aims to investigate the correlations between side chain architectures and properties of comb-like polymer. On the basis of three series of narrow dispersed comb-like PLLA with various graft length and graft density, this report focus on the effects of the side chain parameters (including graft length and graft density) on the intrinsic viscosity, rheological property, thermal and crystallization behaviors.
Experimental section
Materials
Butadiene (Yanshan Petrochem. Co., polymerization grade) was treated with little of n-butyllithium (n-BuLi) to remove the moisture and inhibitor. n-BuLi (J&K Chemical, 2.5 M solution in n-hexane), was determined by Gilman–Haubein double titration and stored under 0 °C.16 Dichloromethane (CH2Cl2, Aladdin), and cyclohexane were distilled from CaH2 under nitrogen. Tetrahydrofuran was dried over sodium benzophenone ketyl under nitrogen and freshly distilled. The terminating agent 2-propanol was degassed via three freezing–evacuation–thawing cycles. L-Lactide (Jinan Daigangbio. Co., 99%) were triple recrystallized from ethyl acetate respectively, then dried and stored in a glovebox. 1,8-Diazabicyclo[5,4,0]undec-7-ene (DBU, Sigma-Aldrich, 98%) was dried over calcium hydride, distilled under reduced pressure and stored in a glovebox. Trifluoromethanesulfonic acid (TfOH, Aladdin, 98%), formic acid (HCOOH, Aladdin, 88%), hydrogen peroxide (H2O2, 30%), and all other reagents were used as received from the commercial source without further purification.
Instrumentation
The molecular weight and PDI of the polymers were determined by GPC using a Waters 1515 HPLC pump, a Waters 2414 refractive index detector, and PS columns (one PL gel 5 μm 10E4A and one Shodex KF805) in THF as eluent at a flow rate of 0.6 mL min−1 at 35 °C calibration based on PS standards (Shodex PS STD SM-105). 1H NMR spectra were record on a Bruker Avance 400 MHz spectrometer in CDCl3 at 25–30 °C at a concentration of 4% w/v. The specific optical rotation, [a] of the polymer was measured in chloroform at a concentration of 1 g dL−1 at 25 °C using a JASCO J-810 Polari meter at a wavelength of 589 nm. Intrinsic viscosity. The intrinsic viscosities ([η]) of PLLA were determined in CHCl3 by a 0.4 mm Schott Ubbelohde viscometer at 30.0 °C. All samples were filtered before test. Flow time of the solvent and that of each of polymer solutions with four different concentrations below the overlap concentration c* were measured to determine [η]. [η] was estimated via the extrapolation of the relative viscosities (ηr) vs. polymer concentration plot for the synthesized polymer. The thermal behavior of the polymers were measured on differential scanning calorimeter (DSC, TA, Q520). Each sample was heated to 200 °C at a heating rate of 10 °C min−1 in aluminum pans under nitrogen atmosphere. Thermal history was removed by keeping the samples at 200 °C for 3 min. Then samples were rapidly cooled to 100 °C and isothermally crystallized for 30 min, followed by cooling to 20 °C and heated to 200 °C at 10 °C min−1. The crystalline morphology of the polymer was observed using a Leica DM4500P polarized optical microscopy (POM) equipped with a Linkam THMS420 hot stage. The PLLA samples were melted at 200 °C for 3 min to eliminate thermal history and then cooled to the isothermal crystallization temperature at a cooling rate of 40 °C min−1. Then take the optical micrographs at appropriate times during the crystallization to examine the growth rate of PLLA spherulites. Rheological measurements were performed on a stress-controlled Rheometer AR2000 (TA Instruments Ltd.) equipped with parallel-plate geometry (diameter of 25 mm) and a gap of 1 mm at constant temperature 190 °C. Dynamic frequency sweep measurements were carried out in an oscillatory shear mode from 10 to 0.01 rad s−1.
Preparation of comb-like PLLA
Synthesis of macroinitiator. Polybutadiene (PB) of defined molecular weight was synthesized by anionic polymerization of butadiene with BuLi in cyclohexane with a small amount of THF as a modifier in a flask at 50 °C for 3 h, quenched by the addition of excess methanol, and dried under vacuum. The PB product was then epoxidized and then hydroxylated. In the epoxidation step, different molar ratio of formic acid and H2O2 ([1,4-butadiene]0/[HCOOH]0/[H2O2]0 = 1/0.3/0.3, 1/0.4/0.4 and 1/0.5/0.5) were used in order to produce PB with various epoxidation degree. In the hydroxylation step, epoxidized PB (PBE) in THF solution was treated with TfOH and H2O to produce multi-functional initiator PB-OH ([epoxy group]0/[TfOH]0/[H2O]0/[THF]0 = 1/1.5/15/30). The detailed information about the synthesis and characterization of the PB backbone could be found in our previous work.15
Synthesis of comb-like PLLA. The observed PB-OH was then used as a macroinitiator in the ring-opening polymerization (ROP) of L-lactide in CH2Cl2 with 1,8-diazabicyclo[5,4,0]undec-7-ene (DBU) as a catalyst to form graft PLLA. The polymerization proceeded at room temperature within 1 h and was quenched by the addition of benzoic acid. The length of PLLA grafts was simply controlled by varying the feeding ratio ([LA]0/[OH]0 = 10, 30, 50, 70, and 100). The 1H NMR and GPC spectrums are shown in ESI Fig. S1 and S2.† The graft PLLA is denoted by PBxPLLAy, where the x and y represent the calculated number of arms on the PB backbone and the lactide units per arm, respectively.
Results and discussion
Synthesis and characterization of comb-like PLLA
In this study, three series of linear-comb PLLA with different graft density were synthesized through ring-opening polymerization (ROP) of L-lactide, by using 1,8-diazabicyclo[5,4,0]undec-7-ene (DBU) as the catalyst, hydroxylated polybutadiene (PB-OH) as the macroinitiator.
Synthesis of macroinitiator. The polymerization of butadiene in cyclohexane with a small amount of THF as a modifier yielded a polybutadiene (PB) with high 1,4-content (90%).17 The produced PB was partially epoxied only at the 1,4-PB sites.18 Double bond with higher electron density favors the epoxidation, which is relatively less sensitive to steric effect. The ratio of [1,4-butadiene]0/[HCOOH]0/[H2O2]0 was added as 1/0.3/0.3, 1/0.4/0.4 and 1/0.5/0.5, respectively, to produce PB with different epoxidation degree. Accordingly, epoxidized PB with different epoxidation degree were successfully obtained by controlling the addition of H2O2 with proper stirring rate. After hydroxylation step, which was a 100% reaction of the epoxidation groups, three types of PB-OH macroinitiators with designed hydroxylation degree (10.4%, 11.7% and 16.4%, determined by 1H NMR) were prepared. The hydroxylated PB were named PB-OH13, PB-OH18 and PB-OH25, respectively, by the number of the hydroxyl groups and listed in Table 1. The merit of this linear backbone based on anionic polymerization is its narrow molecular weight distribution (PDI ≈ 1.1) and the evenly dispersed hydroxyl groups. Graft PLLA from other linear backbone, for instance, based on linear polyglycerols or PCN, usually has broad PDI (>1.5) and uncontrollable graft density.6a,14
Table 1 Characterization data of macroinitiators
Macroinitiator |
Mna (g mol−1) |
PDIa |
Hydroxylation degreeb |
No.OHc |
Determined by GPC analysis with polystyrene standards. Determined by the 1H NMR. The number of the hydroxyl groups on each polybutadiene chain: [No.OH] = e.g. (4200/58 × 90% × 10.4% × 2), where 58 g mol−1 was calculated as the average molecular weight of each backbone unit. Sample data from ref. 15. |
PB13-OHd |
4200 |
1.1 |
10.4% |
13.4 |
PB18-OH |
4500 |
1.1 |
13.1% |
18.3 |
PB25-OH |
4850 |
1.1 |
16.4% |
24.7 |
Synthesis of comb-like PLLA. The multi-functional PB-OH were then used as macroinitiators in the ROP of L-lactide with DBU as a catalyst to produce graft PLLA. The length of PLLA grafts was simply controlled by varying the feeding ratio ([LA]/[OH] = 10, 30, 50, 70, and 100). The characterization data are summarized in Table 2.
Table 2 Characterization data of comb-like PLLA
Sample |
[OH]a/[L-LA]/[DBU] |
Mn,theob (kg mol−1) |
Mn,NMRc (kg mol−1) |
Mn,GPCd (kg mol−1) |
PDId |
[η]e (dL g−1) |
[η ]theof (dL g−1) |
g′g |
The mole of hydroxyl groups of the PB-OH. Mn,theo = [L-LA]/[OH] × Mn,LA × No.OH + Mn,PB-OH, where Mn,LA is the molecular weight of LA and Mn,PB-OH is the molecular weight of the corresponding PB-OH. Determined by 1H NMR. Determined by GPC analysis with polystyrene standards. Measured in CHCl3 by a 0.4 mm Schott Ubbelohde viscometer at 30 °C. Values of ηtheo were calculated by the eqn (1),19 where K = 5.45, α = 0.73 the Mark–Houwink parameters. Calculated by the eqn (3). Sample data from ref. 15. |
PB13PLLA10h |
1/10/0.1 |
23.9 |
24.4 |
25.0 |
1.19 |
0.35 |
0.57 |
0.61 |
PB13PLLA30h |
1/30/0.3 |
62.8 |
61.2 |
58.4 |
1.15 |
0.58 |
1.06 |
0.55 |
PB13PLLA50 |
1/50/0.5 |
100.7 |
98.3 |
97.6 |
1.13 |
0.74 |
1.54 |
0.48 |
PB13PLLA70h |
1/70/0.5 |
140.6 |
139.6 |
137.1 |
1.14 |
0.97 |
1.98 |
0.49 |
PB13PLLA100h |
1/100/0.5 |
198.9 |
191.5 |
198.6 |
1.16 |
1.20 |
2.59 |
0.46 |
PB18PLLA10 |
1/10/0.1 |
30.4 |
31.4 |
31.3 |
1.23 |
0.32 |
0.67 |
0.48 |
PB18PLLA30 |
1/30/0.3 |
82.3 |
78.3 |
76.2 |
1.22 |
0.59 |
1.29 |
0.46 |
PB18PLLA50 |
1/50/0.5 |
134.1 |
128.3 |
125.7 |
1.16 |
0.75 |
1.86 |
0.41 |
PB18PLLA70 |
1/70/0.5 |
185.9 |
185.1 |
184.0 |
1.17 |
1.01 |
2.45 |
0.41 |
PB25PLLA10 |
1/10/0.1 |
40.8 |
41.6 |
41.6 |
1.23 |
0.33 |
0.82 |
0.40 |
PB25PLLA30 |
1/30/0.3 |
112.8 |
107.1 |
104.2 |
1.22 |
0.64 |
1.62 |
0.40 |
PB25PLLA50 |
1/50/0.5 |
184.8 |
170.8 |
166.1 |
1.21 |
0.83 |
2.28 |
0.36 |
PB25PLLA70 |
1/70/0.5 |
256.8 |
251.5 |
248.6 |
1.19 |
1.12 |
3.05 |
0.37 |
The 1H NMR, GPC equipment were employed to elucidate their fine structures (Fig. S1 and S2†). As seen in the 1H NMR spectrum of graft PLLA with assignments, the resonance of PB main chain (about 5.3–5.4 ppm) could be obviously detected when the molar ratios of LA were low. Moreover, the GPC traces exhibited narrow (PDI ≤ 1.23) and symmetrical elution peaks for all PLLA samples prepared with different graft density and side chain length. Our group recently proved that the kinetics study of the DBU catalyzed ROP reaction in this multi-functional initiator system indicated a first-order kinetic in monomer concentration.15 Thus, these 1H NMR and GPC results unambiguously confirm the efficient initiation of the hydroxyl functional groups on the main chain.
The impact of the chain topology on the solution behavior was investigated by intrinsic viscosities measurement (Fig. S3†). Table 2 summarized the tested intrinsic viscosity ([η]) and the branching factor (g′) for the graft PLLA with different graft degree and various side chain lengths. The [η] of the graft PLLA decreased in the following order: PB13PLLA50 (0.48) ≥ PB18PLLA50 (0.41) ≥ PB25PLLA50 (0.36). The total molecular weight of the graft PLLA with same side chain length increases with the graft density increases, but exhibits a decreased intrinsic viscosity. In CHCl3 solution, linear PLLA behaves more rod-like, graft PLLA exhibits much smaller hydrodynamic volumes.20 For comb-like PLLA, both g′ and [η] further decreased with the graft degree increased. This is consistent with a more compact structure in the highly branched star-like PLLA and in the long chain branched (LCB) PLA.21 The PB25PLLA with the highest graft density exhibited the lowest g′ (≤0.4), which remarkably reduced the intrinsic viscosity of PLLA.
Rheological property
All the rheological measurements were carried out at 190 °C. The scale of the frequency was from 0.01 to 10 Hz. The influences of grafting parameters (graft length and graft density) on the complex modulus (G*) and complex viscosity (η*) were investigated, as defined in eqn (1) and (2): |
G* = [(G′)2 + (G′′)2]1/2
| (1) |
|
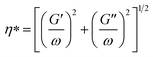 | (2) |
where G′ is storage modulus, G′′ is loss modulus and ω is angular frequency. The influences of grafting parameters on the rheology were detailed studied in the following sections.
Effect of graft length on the rheology. Take PB18PLLA as an example, Fig. 1 shows the variation of G* (Fig. 1a) and η* (Fig. 1b) as a function of the frequency plots of PB18PLLA with varied side chain length. The complex modulus of graft PB18PLLA increase with the length of side chain, or with the total molecular weight. Besides G*, η* is also very sensitive to the side chain length. To fit the viscosity data by the cross equation written as:22 |
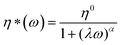 | (3) |
where η0 is the zero-shear viscosity, λ is a relaxation time which inversely accounts for the onset of shear-thinning region, and α is a shear-thinning index. For all the graft PLLA samples, a continuous shear-thinning region can be observed in the measured frequency range, without any symptom of leveling off at low shear frequencies. Therefore, the value of η0 can hardly be related to the Newtonian viscosity of the general linear viscoelastic model. In order to correlate the rheological property with topological structure PLLA, η0 can be approximated with the η* obtained at the frequency of 0.01 Hz, which is listed in Table 2. As shown in Table 2, the value of η0 increases from 0.79 Pa s (PB18PLLA10) to 6.37 Pa s (PB18PLLA70). The above results indicate that the increase of side chain length remarkably enhances the η* and strengthen the G* of PB18PLLA samples. Similar trends could also be observed in the PB13PLLA and PB25PLLA samples.
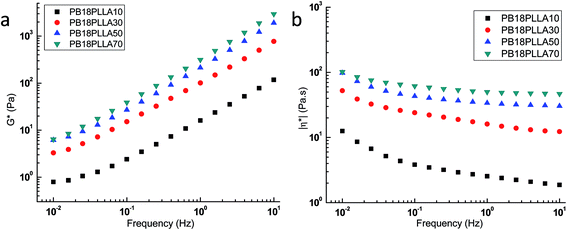 |
| Fig. 1 Variation of modulus (a) and complex viscosity (b) as a function of the frequency plots of PB18PLLA with different side chain length at 190 °C. | |
Effect of graft density on the rheology. Fig. 2 shows the variation of G* (Fig. 2a) and η* (Fig. 2b) as a function of the frequency plots of graft PLLA with similar side chain length but varied graft density. The sample PB25PLLA30 with highest graft density exhibited the highest η0 (8.57 Pa s for PB25PLLA30, 0.69 Pa s for PB18PLLA30, 3.28 Pa s for PB13PLLA30, respectively, in Table 2) and more enhanced G* compared with PB18PLLA30 and PB13PLLA30. Similar results can also be obtained from the comparison between PB13PLLA10, PB18PLLA10 and PB25PLLA10. Therefore, the rheological property of graft PLLA samples with same side chain length can be enhanced through increasing the graft density.
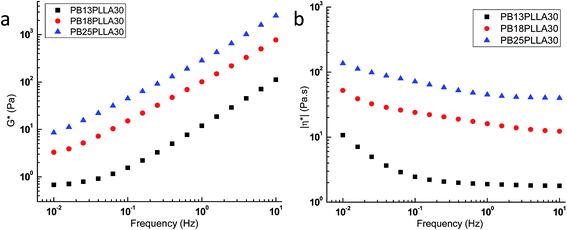 |
| Fig. 2 Variation of modulus (a) and complex viscosity (b) as a function of the frequency plots of different graft density PLLA with same side chain length. | |
Comparison of the effects of graft length and graft density on the rheology. Due to the above experimental results, the increase of graft length or graft density can lead to an enhancement of rheological properties. Other copolymers with similar graft structure exhibited the same regulation.2b,21b,23 However, the comparison of the effects of the two grafting parameters on the rheology was seldom studied, which was ascribed to the difficulty of preparing narrow dispersed comb-like PLLA samples. To further distinguish the influence of grafting parameters of comb-like PLLA, well-defined samples were synthesized and rheological measured. Fig. 3 shows the variation of G* (Fig. 3a) and η* (Fig. 3b) as a function of the frequency plots of graft PLLA with similar molecular weight but apparently different graft density and graft length. The graft density of PB25PLLA50 is about 2 times as that of PB13PLLA100, and the side chain length of the former is only half of that of the latter. As shown in Table 2, PB25PLLA50 exhibits a three times higher value of η0 than PB13PLLA100 (11.57 Pa s for PB25PLLA50 and 3.07 Pa s for PB13PLLA100). Besides, the G* and η* values of the samples followed the order PB13PLLA100 < PB18PLLA70 < PB25PLLA50 through all frequencies, in parallel with their graft densities, which indicates an increased rheological property. This result reveals that the graft density has more significant influence on rheological property of the graft PLLA compared to the graft length in this system.
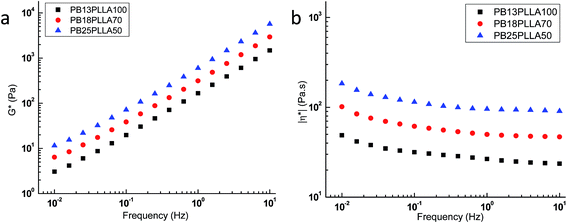 |
| Fig. 3 Variation of modulus (a) and complex viscosity (b) as a function of the frequency plots of different graft density PLLA with similar molecular weight. | |
Crystallization behavior
Thermal property. Due to the slow crystallization of PLLA, no crystallization peak can be observed during the nonisothermal quenching process (under 10 °C min−1 cooling rate, Fig. S4†). To better study the graft density effect on the thermal behavior of graft PLLA, after removing thermal history at 200 °C, samples were rapidly cooled to 100 °C and isothermally crystallized for 30 min. Fig. 4 shows the second heating DSC curves of similar molecular weight comb-like PLLA with different graft density. From the data summarized in Table 3, Tg decreases as the following order: PB13PLLA70 (51.2 °C) > PB18PLLA50 (49.4 °C) > PB25PLLA30 (46.8 °C). There are many parameters that influence glass transition temperature when chain structure changed.10c By changing the graft density of the comb-like PLLA, two factors are altered. One is the free volume, which increases by the higher graft density gives more flexible chain ends, contributes to decrease in Tg. The other is the segmental mobility, which decreases by chains are much closer and restricted in movement, contributes to increase in Tg. Both directions in Tg between branched PLA and linear PLA were reported by Sakamoto and Tsuji.24 Therefore, Tg may changes with the degree of graft changes depending on which of the two counteracting parameters controls. Here, the Tg decreases with graft density increases. The behavior of graft polymer is consistent with additional free volume from the increase in chain end.
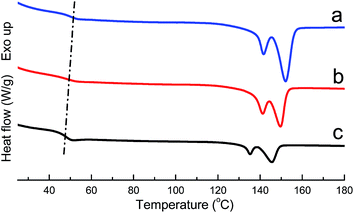 |
| Fig. 4 DSC curves of similar molecular weight comb-like PLLA with different graft density: (a) PB13PLLA70, (b) PB18PLLA50, and (c) PB25PLLA30. | |
Table 3 Thermal properties of comb-like PLLA
Sample |
Tg (°C) |
Tm (°C) |
ΔHma (J g−1) |
Xca (%) |
G120 °Cb (μm min−1) |
Determined from the DSC curve for the second heating. Xc (%) = ΔHm/ΔHm0 × 100, the fusion enthalpy of 100% PLLA crystalline (ΔHm0) was assumed to be 93.7 J g−1. G radius growth rate of spherulites: measured by POM at 120 °C. |
PB13PLLA70 |
51.2 |
151.9 |
39.8 |
42.5 |
0.71 |
PB18PLLA50 |
49.4 |
149.6 |
33.4 |
35.6 |
0.97 |
PB25PLLA30 |
46.8 |
145.5 |
25.4 |
27.1 |
1.31 |
It is noticed in Fig. 4, samples displayed double melting peaks, which is due to the melt-reorganization.25 The melting temperature (Tm), crystallization enthalpy (ΔHm) and the relative degree of crystallinity (Xc) were summarized in Table 3. Tm and ΔHm of the graft PLLA decrease with the graft density increases (PB13PLLA70 > PB18PLLA50 > PB25PLLA30). Similar trend has been reported in some other graft polymers.2b,21a,26 The branching and grafting architecture usually delay and disturb the crystallization of polymer, due to the lower degree of molecular symmetry.24,27 Moreover, the mobility and reputation ability of PLA chains are restrained by the graft structure, which hinders the crystallization process and makes a contribution to the imperfection. As a result, the Tg, Tm, ΔHm and Xc of graft PLLA all decrease with the graft density increases.
Spherulitic morphology and growth rate. Spherulitic morphology and spherulite growth rate (G) of comb-like PLLAs were investigated by optical microscopy under cross-polar conditions in isothermal mode. In the typical polarization photomicrographs of graft PLLA (Fig. 5 and 6), normal spherulites were formed in order with typical Maltese-cross patterns. Therefore, the graft structure does not cause obvious structural defects during the crystal growth in this system.
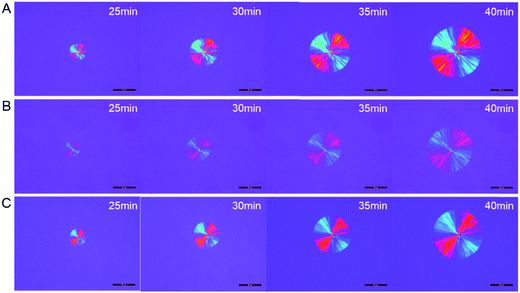 |
| Fig. 5 Selected POM micrographs during isothermal crystallization at 125 °C. (A) PB13PLLA10, (B) PB18PLLA10, (C) PB25PLLA10. The scale bar in the bottom micrograph of panel A represents 50 μm and applies to all the micrographs. | |
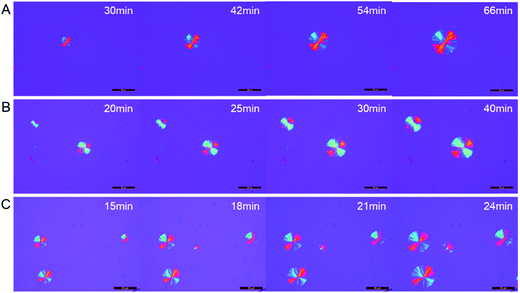 |
| Fig. 6 Selected POM micrographs during isothermal crystallization at 120 °C. (A) PB13PLLA70, (B) PB18PLLA50, (C) PB25PLLA30. | |
Effect of graft length on the spherulite growth rate. The G values of PB13PLLA, PB18PLLA and PB25PLLA plotted as a function of crystallization temperature (Tc) in Fig. 7a–c, respectively. Since the crystallization of PLLA under lower Tc gives higher nucleation density, the significant impingement of spherulites occurs when temperature lower than 100 °C. G values of each graft PLLA were measured at Tc ≥ 100 °C, every point per 5 °C. The G value of PB13PLLA at 110 °C, for instance, the radius growth rate of the PB13PLLA spherulites decreased in the following order: PB13PLLA10 (2.71 μm min−1) ≥ PB13PLLA30 (1.30 μm min−1) ≥ PB13PLLA50 (0.97 μm min−) ≥ PB13PLLA70 (0.65 μm min−1) ≥ PB13PLLA50 (0.35 μm min−1). In Fig. 7, the spherulite growth rate, of all the three series of graft PLLA (PB13PLLA, PB18PLLA and PB25PLLA, Mn > 25.0 kg mol−1) at all tested temperature, decreases with the side chain length increases due to the weakened chain mobility. The decreasing G is very common among ordinary synthetic polymers,28 and also essentially consistent with the results reported by Pennings and co-workers.29 However, Pengju Pan et al. reported that increasing the chain length of the side chains of the graft PLLA resulted in a higher crystallization rate30 (DP of LA in the graft polymer was less than 105. The molecular weight of PLLA content was less than 15.1 kg mol−1). The very different results also occurred in linear PLLAs. Sheng Xiang et al. recently investigated the effect of Mn on the crystallization rate of the linear PLLA.31 They demonstrated that: when Mn ≤ 18.6 kg mol−1, G increased with Mn increased; when Mn ≥ 18.6 kg mol−1, G decreased with Mn increased. On this point, the interesting regulation between molecular weight and crystallization rate of PLLA is consistent for both linear PLLA and graft PLLA.
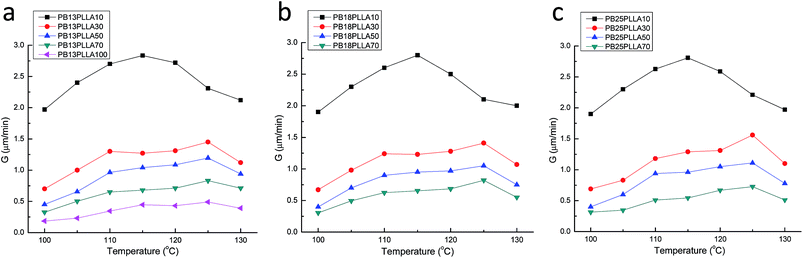 |
| Fig. 7 Radius growth rate of spherulites (G) versus crystallization temperature (Tc) for (a) PB13PLLA, (b) PB18PLLA, and (c) PB25PLLA. | |
Effect of graft density on the spherulite growth rate. The graft density of the polymer also plays a significant role in the process of crystallization. Take the isothermal crystallization of PB13PLLA10, PB18PLLA10, and PB25PLLA10 under 125 °C as an example (Fig. 5). The graft PLLA with different graft density but same molecular weight per arm (Mn,arm) exhibited the similar G values (around 2.2 μm min−1), almost the same spherulite size under the same time. The decreasing of spherulite growth by enhanced total molecular weight was balanced with the increased density of the terminal groups. As seen in Fig. 7, the G values of three series of PLLA with same side chain length ordered as the following: PB13PLLA10 ≈ PB18PLLA10 ≈ PB25PLLA10 ≥ PB13PLLA30 ≈ PB18PLLA30 ≈ PB25PLLA30 ≥ PB13PLLA50 ≈ PB18PLLA50 ≈ PB25PLLA50 ≥ PB13PLLA70 ≈ PB18PLLA70 ≈ PB25PLLA70. This finding may be ascribed to the fact that the main factor determining G is not Mn but Mn,arm.
Comparison of the effects of graft length and graft density on the spherulite growth rate. Fig. 6 shows graft PLLA with similar total molecular weight but different graft density under 120 °C. The G value of graft PLLA spherulites at 120 °C increased in the following order: PB13PLLA70 (0.71 μm min−1) ≤ PB18PLLA50 (0.97 μm min−1) ≤ PB25PLLA30 (1.31 μm min−1). The enhancement of spherulite growth could be explained by reduced side chain length through increased graft density. Under the similar molecular weight, more side chains means more chain ends and much shorter chain length. In some reports, PLAs with branch or graft structures can be appropriate for fast crystallization during cooling, because the branch or graft points can act as nucleating agents to accelerate crystallization, but the perfection of the resulting crystal would be disturbed as a consequence.10b,32 However, in this work the imperfection of the crystal did not appear on the graphs of spherulites. At least, there is no obvious difference between the 13–25 arms PLLAs. Hideto Tsuji et al. reported that, compared with 3 arms PLLA, the multi-arm PLLA showed different thermal stability against star-shaped PLLA.33 In such hyperbranched PLLA (16–21 arms PLLA), the effect of increased density of the terminal groups must be more remarkable compared with that in few-arm PLLA and the branching will not reduce the chain mobility.34
Conclusions
The well-defined narrow dispersed comb-like PLLA with various graft length and graft density has been achieved. The graft density was controlled through changing the oxidation degree of the PB backbone. The grafted side chain length was controlled by the ratio of monomer and initiator. All the initiation sites on the macroinitiator seem to react under room temperature during the ROP of L-lactide, which is strongly evidenced by 1H NMR and GPC results. Three sets of PLLA samples (Mn range from 25.0 kg mol−1 to 248.6 kg mol−1, PDI < 1.23) with different graft densities (13, 18 and 25 arms per main chain) and side chain length (10–100 lactide units per arm) were prepared efficiently. The correlations between the grafting parameters and the properties of the graft PLLA were systematically investigated. The increases of graft length and graft density both lead to an elevated value of zero-viscosity η0, enhanced complex modulus G* and increased complex viscosity η*. Comparatively, graft density contributes more to the improvement of rheological property than graft length does. As a result, the graft parameters have an obvious influence on the rheological property. DSC results indicated that the increase of graft density resulted in a lower Tg, and also the less perfect crystals that were responsible for the lower Tm and ΔHm values observed experimentally. From the isothermal crystallization, the increase of graft density exhibits an improvement of spherulitic growth rate G, while the increase of chain length reduces G. The value of G at 120 °C was found to increase from 0.71 μm min−1 for PB13PLLA70 with 13 arms to 1.31 μm min−1 for PB25PLLA30 with 25 arms. Moreover, the results indicate that the main factor determining G is not Mn but Mn,arm. In other words, rheological and crystallization properties of comb-like graft PLLA were correlated to the side chain structures. The information generated would help develop structure–property relationships, which could better illustrate the synthesis and the application of the comb-like PLLA.
Acknowledgements
This work was financially supported by National Program on Key Basic Research Program of China (973 Program No. 2015CB654700 (2015CB654701)) and National Science Foundation of China (No. 21174021).
References
-
(a) K. A. Athanasiou, G. G. Niederauer and C. M. Agrawal, Sterilization, toxicity, biocompatibility and clinical applications of polylactic acid/polyglycolic acid copolymers, Biomaterials, 1996, 17(2), 93–102 CrossRef CAS PubMed;
(b) D. J. Sawyer, Bioprocessing – no longer a field of dreams, Macromol. Symp., 2003, 201(1), 271–282 CrossRef CAS;
(c) M. Okada, Chemical syntheses of biodegradable polymers, Prog. Polym. Sci., 2002, 27(1), 87–133 CrossRef CAS;
(d) A. P. Gupta and V. Kumar, New emerging trends in synthetic biodegradable polymers – polylactide: a critique, Eur. Polym. J., 2007, 43(10), 4053–4074 CrossRef CAS.
-
(a) J. Dorgan, H. Lehermeier and M. Mang, Thermal and Rheological Properties of Commercial-Grade Poly(Lactic Acid)s, J. Polym. Environ., 2000, 8(1), 1–9 CrossRef;
(b) S. Corneillie and M. Smet, PLA architectures: the role of branching, Polym. Chem., 2015, 6(6), 850–867 RSC.
-
(a) Y. Li, J. Zou, B. P. Das, M. Tsianou and C. Cheng, Well-Defined Amphiphilic Double-Brush Copolymers and Their Performance as Emulsion Surfactants, Macromolecules, 2012, 45(11), 4623–4629 CrossRef CAS;
(b) R. Fenyves, M. Schmutz, I. J. Horner, F. V. Bright and J. Rzayev, Aqueous Self-Assembly of Giant Bottlebrush Block Copolymer Surfactants as Shape-Tunable Building Blocks, J. Am. Chem. Soc., 2014, 136(21), 7762–7770 CrossRef CAS PubMed.
-
(a) Q. Cai, Y. Wan, J. Bei and S. Wang, Synthesis and characterization of biodegradable polylactide-grafted dextran and its application as compatilizer, Biomaterials, 2003, 24(20), 3555–3562 CrossRef CAS PubMed;
(b) C. Nouvel, J. Raynaud, E. Marie, E. Dellacherie, J. L. Six and A. Durand, Biodegradable nanoparticles made from polylactide-grafted dextran copolymers, J. Colloid Interface Sci., 2009, 330(2), 337–343 CrossRef CAS PubMed.
-
(a) Y. Wu, M. Li and H. Gao, Polymeric micelle composed of PLA and chitosan as a drug carrier, J. Polym. Res., 2009, 16(1), 11–18 CrossRef CAS;
(b) C. L. Lo, K. M. Lin, C. K. Huang and G. H. Hsiue, Self-Assembly of a Micelle Structure from Graft and Diblock Copolymers: An Example of Overcoming the Limitations of Polyions in Drug Delivery, Adv. Funct. Mater., 2006, 16(18), 2309–2316 CrossRef CAS;
(c) J.-M. Rabanel, J. Faivre, S. Tehrani, A. Lalloz, P. Hildgen and X. Banquy, Effect of polymer architecture on the structural and biophysical properties of PEG–PLA nanoparticles, ACS Appl. Mater. Interfaces, 2015, 7(19), 10374–10385 CrossRef CAS PubMed;
(d) M. H. Seo, B. O. Kim, I. J. Choi, M. S. Shim, et al., Biodegradable branched polylactide derivatives capable of forming polymeric micelles, and their preparation method and use, EP, US 8021652 B2, 2011.
-
(a) C. Gottschalk, F. Wolf and H. Frey, Multi-Arm Star Poly(L-Lactide) with Hyperbranched Polyglycerol Core, Macromol. Chem. Phys., 2007, 208(15), 1657–1665 CrossRef CAS;
(b) M. Adeli and R. Haag, Multiarm star nanocarriers containing a poly(ethylene imine) core and polylactide arms, J. Polym. Sci., Part A: Polym. Chem., 2006, 44(19), 5740–5749 CrossRef CAS;
(c) C. Hiemstra, Z. Zhong, L. Li, P. J. Dijkstra and J. Feijen, In situ Formation of Biodegradable Hydrogels by Stereocomplexation of PEG–(PLLA)8 and PEG–(PDLA)8 Star Block Copolymers, Biomacromolecules, 2006, 7(10), 2790–2795 CrossRef CAS PubMed;
(d) H. Tsuji and T. Hayashi, Hydrolytic degradation and crystallization behavior of linear 2-armed and star-shaped 4-armed poly(L-lactide)s: effects of branching architecture and crystallinity, J. Appl. Polym. Sci., 2015, 132(20), 41983 CrossRef.
-
(a) G. Theryo, F. Jing, L. M. Pitet and M. A. Hillmyer, Tough Polylactide Graft Copolymers, Macromolecules, 2010, 43(18), 7394–7397 CrossRef CAS;
(b) F. Tasaka, H. Miyazaki, Y. Ohya and T. Ouchi, Synthesis of Comb-Type Biodegradable Polylactide through Depsipeptide–Lactide Copolymer Containing Serine Residues, Macromolecules, 1999, 32(19), 6386–6389 CrossRef CAS.
-
(a) F. K. Wolf and H. Frey, Inimer-Promoted Synthesis of Branched and Hyperbranched Polylactide Copolymers, Macromolecules, 2009, 42(24), 9443–9456 CrossRef CAS;
(b) J. Liu, L. Lou, W. Yu, R. Liao, R. Li and C. Zhou, Long chain branching polylactide: structures and properties, Polymer, 2010, 51(22), 5186–5197 CrossRef CAS;
(c) J. Liu, S. Zhang, L. Zhang and Y. Bai, Preparation and rheological characterization of long chain branching polylactide, Polymer, 2014, 55(10), 2472–2480 CrossRef CAS.
- M. G. Mckee, S. Unal, G. L. Wilkes and T. E. Long, Branched polyesters: recent advances in synthesis and performance, Prog. Polym. Sci., 2005, 30(5), 507–539 CrossRef CAS.
-
(a) M. Nofar, W. Zhu and C. B. Park, Effect of dissolved CO2 on the crystallization behavior of linear and branched PLA., Polymer, 2012, 53(15), 3341–3353 CrossRef CAS;
(b) M. Nofar, W. Zhu, C. B. Park and J. Randall, Crystallization Kinetics of Linear and Long-Chain-Branched Polylactide, Ind. Eng. Chem. Res., 2011, 50(24), 13789–13798 CrossRef CAS;
(c) S. Nouri, C. Dubois and P. G. Lafleur, Synthesis and characterization of polylactides with different branched architectures, J. Polym. Sci., Part B: Polym. Phys., 2015, 53(7), 522–531 CrossRef CAS.
- M. Mihai, M. A. Huneault and B. D. Favis, Rheology and extrusion foaming of chain-branched poly(lactic acid), Polym. Eng. Sci., 2010, 50(3), 629–642 CAS.
- H. Tsuji, T. Miyase, Y. Tezuka and S. K. Saha, Physical properties, crystallization, and spherulite growth of linear and 3-arm poly(L-lactide)s, Biomacromolecules, 2005, 6(1), 244–254 CrossRef CAS PubMed.
- C. Zhao, D. Wu, N. Huang and H. Zhao, Crystallization and thermal properties of PLLA comb polymer, J. Polym. Sci., Part B: Polym. Phys., 2008, 46(6), 589–598 CrossRef CAS.
- G. Theryo, J. Feng, L. M. Pitet and M. A. Hillmyer, Tough Polylactide Graft Copolymers, Macromolecules, 2010, 43(18), 7394–7397 CrossRef CAS.
- X. Leng, Z. Wei, Y. Ren, Y. Li, Y. Wang and Q. Wang, Facile synthesis and comparative study of poly(L-lactide) with linear-comb and star-comb architecture, RSC Adv., 2015, 5(99), 81482–81491 RSC.
- H. Gilman and A. H. Haubein, The Quantitative Analysis of Alkyllithium Compounds1, J. Am. Chem. Soc., 1944, 66(9), 1515–1516 CrossRef CAS.
-
(a) G. D. Liang, J. T. Xu and Z. Q. Fan, Synthesis of polystyrene-b-poly(ethylene-co-butene) block copolymers by anionic living polymerization and subsequent noncatalytic hydrogenation, J. Appl. Polym. Sci., 2006, 102(3), 2632–2638 CrossRef CAS;
(b) H. Zhang, Y. Li, C. Zhang, Z. Li, X. Li and Y. Wang, Synthesis of Dendrigraft Star–Comb Polybutadienes by Anionic Polymerization and Grafting-onto Methodology, Macromolecules, 2009, 42(14), 5073–5079 CrossRef CAS.
- D. V. Deubel, Are Peroxyformic Acid and Dioxirane Electrophilic or Nucleophilic Oxidants?, J. Org. Chem., 2001, 66(11), 3790–3796 CrossRef CAS PubMed.
- J. E. Mark, Polymer Data Handbook, Oxford University Press, 1999 Search PubMed.
- A. Breitenbach and T. Kissel, Biodegradable comb polyesters: part 1 synthesis, characterization and structural analysis of poly(lactide) and poly(lactide-coglycolide) grafted onto water-soluble poly(vinyl alcohol) as backbone, Polymer, 1998, 39(14), 3261–3271 CrossRef CAS.
-
(a) Q. Hao, F. Li, Q. Li, Y. Li, L. Jia, J. Yang, Q. Fang and A. Cao, Preparation and crystallization kinetics of new structurally well-defined star-shaped biodegradable poly(L-lactide)s initiated with diverse natural sugar alcohols, Biomacromolecules, 2005, 6(4), 2236–2247 CrossRef CAS PubMed;
(b) L. Wang, X. Jing, H. Cheng, X. Hu, L. Yang and Y. Huang, Rheology and Crystallization of Long-Chain Branched Poly(L-Lactide)s with Controlled Branch Length, Ind. Eng. Chem. Res., 2012, 51(33), 10731–10741 CrossRef CAS.
- M. M. Cross, Rheology of non-Newtonian fluids: a new flow equation for pseudoplastic systems, J. Colloid Sci., 1965, 20(5), 417–437 CrossRef CAS.
-
(a) S. J. Dalsin, M. A. Hillmyer and F. S. Bates, Linear Rheology of Polyolefin-Based Bottlebrush Polymers, Macromolecules, 2015, 48(13), 4680–4691 CrossRef CAS;
(b) J. De la Fuente, M. Fernández-García, M. Cerrada, H. W. Spiess and M. Wilhelm, Small-angle X-ray scattering and linear melt rheologyof poly(tert-butyl acrylate-g-styrene) graft copolymers, Polymer, 2006, 47(5), 1487–1495 CrossRef CAS;
(c) Q. Ran, P. Somasundaran, C. Miao, J. Liu, S. Wu and J. Shen, Effect of the length of the side chains of comb-like copolymer dispersants on dispersion and rheological properties of concentrated cement suspensions, J. Colloid Interface Sci., 2009, 336(2), 624–633 CrossRef CAS PubMed;
(d) Y. Lin, J. Zheng, K. Yao, H. Tan, G. Zhang, J. Gong, T. Tang and D. Xu, Synthesis and linear rheological property of comb-like styrene-based polymers with a high degree of branch chain, Polymer, 2015, 59, 252–259 CrossRef CAS.
- Y. Sakamoto and H. Tsuji, Crystallization behavior and physical properties of linear 2-arm and branched 4-arm poly(L-lactide)s: effects of branching, Polymer, 2013, 54(9), 2422–2434 CrossRef CAS.
- Y.-T. Shieh and G.-L. Liu, Effects of carbon nanotubes on crystallization and melting behavior of poly(L-lactide) via DSC and TMDSC studies, J. Polym. Sci., Part B: Polym. Phys., 2007, 45(14), 1870–1881 CrossRef CAS.
- W. Yuan, J. Yuan, F. Zhang, X. Xie and C. Pan, Synthesis, characterization, crystalline morphologies, and hydrophilicity of brush copolymers with double crystallizable side chains, Macromolecules, 2007, 40(25), 9094–9102 CrossRef CAS.
- Q. Hao, F. Li, Q. Li, Y. Li, L. Jia, J. Yang, Q. Fang and A. Cao, Preparation and Crystallization Kinetics of New Structurally Well-Defined Star-Shaped Biodegradable Poly(L-Lactide)s Initiated with Diverse Natural Sugar Alcohols, Biomacromolecules, 2005, 6(4), 2236–2247 CrossRef CAS PubMed.
-
(a) J. Hoffman, G. T. Davis, J. Lauritzen and N. Hannay, Treatise on solid state chemistry, ed. N. B. Hannay, 1976, p. 497 Search PubMed;
(b) T. Miyata and T. Masuko, Crystallization behaviour of poly(L-lactide), Polymer, 1998, 39(22), 5515–5521 CrossRef CAS.
- R. Vasanthakumari and A. J. Pennings, Crystallization kinetics of poly(L-lactic acid), Polymer, 1983, 24(2), 175–178 CrossRef CAS.
- N. Ding, B. Shentu, P. Pan, G. Shan, Y. Bao and Z. Weng, Synthesis and crystallization of poly(vinyl acetate)-g-poly(L-lactide) graft copolymer with controllable graft density, Ind. Eng. Chem. Res., 2013, 52(36), 12897–12905 CrossRef CAS.
- S. Xiang, S. Jun, L.-d. Feng, X.-s. Chen, F.-q. Liu and S.-y. Huang, Effects of molecular weight on the crystallization and melting behaviors of poly(L-lactide), Chin. J. Polym. Sci., 2016, 34(1), 69–76 CrossRef CAS.
- L. Wang, X. Jing, H. Cheng, X. Hu, L. Yang and Y. Huang, Rheology and crystallization of long-chain branched poly(L-lactide)s with controlled branch length, Ind. Eng. Chem. Res., 2012, 51(33), 10731–10741 CrossRef CAS.
- H. Tsuji, T. Miyase, Y. Tezuka and S. K. Saha, Physical Properties, Crystallization, and Spherulite Growth of Linear and 3-Arm Poly(L-Lactide)s, Biomacromolecules, 2005, 6(1), 244–254 CrossRef CAS PubMed.
- Y.-L. Zhao, Q. Cai, J. Jiang, X.-T. Shuai, J.-Z. Bei, C.-F. Chen and F. Xi, Synthesis and thermal properties of novel star-shaped poly(L-lactide)s with starburst PAMAM-OH dendrimer macroinitiator, Polymer, 2002, 43(22), 5819–5825 CrossRef CAS.
Footnote |
† Electronic supplementary information (ESI) available. See DOI: 10.1039/c6ra02697d |
|
This journal is © The Royal Society of Chemistry 2016 |