DOI:
10.1039/C6RA01862A
(Paper)
RSC Adv., 2016,
6, 30886-30894
Multi-color luminescence properties and energy transfer behaviour in host-sensitized CaWO4:Tb3+,Eu3+ phosphors†
Received
21st January 2016
, Accepted 13th March 2016
First published on 15th March 2016
Abstract
A series of host-sensitized and color-tunable CaWO4:Tb3+,Eu3+ phosphors were prepared via a high-temperature solid-state reaction route, and the crystal structure and luminescence properties, especially the energy transfer behavior, were investigated in detail. Under UV radiation, the CaWO4 host presents a broad emission band from about 320 to 600 nm centered around 415 nm, ascribed to the charge transfer in WO42− groups; while Eu3+ and Tb3+ ion doped CaWO4 samples show both host emission and respective emission lines derived from the characteristic f–f transitions of activators, which present abundant emission colors owing to an efficient energy transfer from the host to Eu3+/Tb3+ ions. The energy transfer from the host to Eu3+ and Tb3+ was evidenced by directly observing appreciable overlap between the excitation spectrum of the host and the emission spectrum of Eu3+/Tb3+, as well as by the decreased decay time of host emission with increasing Eu3+/Tb3+ concentration. The energy transfer mechanisms in CaWO4:Eu3+/Tb3+ phosphors have been determined to be a resonant type via dipole–dipole interaction. In the Eu3+ and Tb3+ co-doped system, CaWO4:Eu3+,Tb3+, the energy transfer phenomenon not only occurs from host to activators, but also from Tb3+ to Eu3+, resulting in color-tunable emission including white light by simply adjusting the doping concentration of Eu3+ and Tb3+ ions. The PL properties of the as-prepared materials indicate their promising application in solid-state lighting fields.
1. Introduction
Recently, the development of inorganic luminescent materials has attracted fast growing interest due to their indispensable applications in lighting apparatus (e.g., fluorescent tubes and white light-emitting diodes), display devices (e.g., cathode ray tubes, field emission displays, and vacuum fluorescent displays), solid-state lasers, biological labeling, medical devices, and so on.1–9 In particular, white light-emitting diodes (WLEDs) have received wide consideration due to their favorable properties of energy saving, high energy efficiency, long operation time, and environmental friendliness.10–15 Therefore, more attention has been paid to searching for highly efficient phosphors with respect to the wide possible applications.16–19
Rare earth ions have been playing an irreplaceable role in modern lighting and display fields due to the abundant emission colors based on their 4f–4f or 4f–5d transitions.20–26 In particular, the Eu3+ ions, as one of the most common and widely used activators, can primarily emit red color originating from its characteristic transitional emissions of 5D0,1,2–7FJ (J = 4, … , 0). Tb3+-doped phosphors have been broadly applied as green emitting materials based on 5D4–7F5 transition of Tb3+ ions.27,28 However, the f–f transitions of rare earth ions generally have low excitation efficiencies because of their forbidden parity selection rules. Therefore, utilizing the host sensitization via energy transfer from the excited host to rare earth ions is an effective way to enhance the emission intensity of activators.
Metal tungstates have emerged as an important family of luminescent materials owing to their unique structural, excellent thermal stability and interesting luminescence properties.29–33 Among them, calcium tungstate (CaWO4) is a self-activated phosphor that can emit a high-efficiency blue emission when excited by ultraviolet light, which due to the tetrahedral WO42− groups in the host lattices.34,35 The luminescent properties of CaWO4 and rare earth ions doped CaWO4 have been described a few years ago.36 Unfortunately, in those reports for Eu3+ or Tb3+ doped CaWO4, there is not a clear description about their photoluminescence (PL) and relevant luminescent mechanism. Furthermore, detailed investigations on PL of CaWO4:A (A = Eu3+ and/or Tb3+) have not been performed. Accordingly, in this paper, we report the synthesis of Eu3+, Tb3+ single-doped and Eu3+, Tb3+ codoped CaWO4 phosphors using a solid-state reaction process, and investigated their PL properties and energy transfer mechanism in more detail. Under UV excitation, energy transfer from host to activators exists in the singly-doped samples, which leads to tunable emission color from blue to red for CaWO4:Eu3+ and green for CaWO4:Tb3+. While, in Eu3+ and Tb3+ co-doped system, CaWO4:Eu3+,Tb3+, energy transfer phenomenon not only occurs from host to activators, but also from Tb3+ to Eu3+. Furthermore, a white emission in a single-composition was achieved by blending simultaneously the blue, green, and red emissions of host, Tb3+, and Eu3+ in the CaWO4:8%Eu3+,4%Tb3+ phosphor. It suggests that the as-prepared products would have good potential application in the solid-state lighting fields.
2. Experimental
2.1 Materials and synthesis
The CaWO4:Eu3+, CaWO4:Tb3+, and CaWO4:Eu3+,Tb3+ samples were all prepared through a high-temperature solid-state method with CaCO3 (A.R.), WO3 (A.R.), Tb4O7 (99.99%), and Eu2O3 (99.99%). In a typical process, the stoichiometric amounts of the raw materials were first thoroughly mixed by grinding them in an agate mortar with an appropriate amount of ethanol and then dried at 80 °C for 0.5 h. After reground for 20 min, the powder mixtures were loaded into the alumina crucible and transferred to the box furnace to calcine at 1100 °C for 6 h in air condition with a heating rate of 3 °C min−1. Finally, the prepared samples were furnace-cooled to room temperature and then ground once more for the subsequent measurements.
2.2 Characterization
The X-ray diffraction (XRD) patterns were recorded on a Rigaku D/max 2400 X-ray diffractometer at a scanning rate of 10° min−1 in the 2θ range from 5° to 80° with graphite-monochromatized CuKα radiation (λ = 0.15405 nm). The photoluminescence emission (PL) and photoluminescence excitation (PLE) spectra as well as quantum efficiency were measured using a Hitachi F-7000 spectrophotometer equipped with a 150 W xenon lamp as the excitation source. The luminescent decay curves were measured using an FLS920 spectrofluorometer (Edinburgh Instruments). All the measurements were performed at room temperature (RT).
3. Result and discussion
3.1 Phase identification and structure
The composition and phase purity of as-prepared representative samples CaWO4 host, CaWO4:4%Eu3+, CaWO4:4%Tb3+, CaWO4:4%Tb3+,2%Eu3+ and CaWO4:6%Tb3+,1%Eu3+ were firstly identified by XRD patterns, as shown in Fig. 1. It is obvious that all diffraction peaks of the synthesized phosphors are well assigned to the tetragonal phase of CaWO4 according to the JCPDS card no. 41-1431, and no traces of impurity phases are detected, implying that the Eu3+ and Tb3+ ions can be completely incorporated into the host lattice and their introductions do not cause any significant changes to the crystal structure. The CaWO4 crystallizes belong to a scheelite-type tetragonal structure with the space group of I41/a (88) and cell parameters of a = b = 5.243 Å, c = 11.373 Å, V = 312.63 Å3 and Z = 4.37,38 Fig. 2 shows the unit cell structure of CaWO4 and the coordination environments of the Ca2+ and W6+ sites. It can be seen that the tungsten (W) atoms are surrounded by four oxygen atoms, forming the tetrahedral [WO4] clusters, where the tetrahedral angles are slightly distorted. The calcium (Ca) atoms are coordinated by eight oxygen atoms ([CaO8] clusters) and have a S4 point symmetry with no inversion center.39 There is only one type of Ca cationic site with a Wyckoff position 4b for activators to accommodate attributed to the similar ionic radius of Ca2+ [r = 1.00 Å for coordination number (CN) = 8] and Eu3+ (r = 0.95 Å for CN = 8) and Tb3+ (r = 0.92 Å for CN = 8).
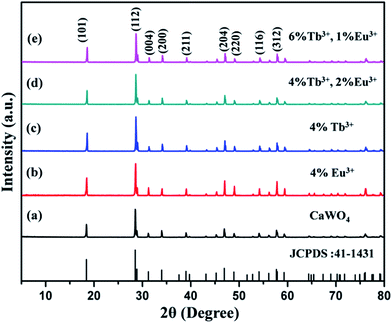 |
| Fig. 1 XRD patterns of representative CaWO4 host (a), CaWO4:4%Eu3+ (b), CaWO4:4%Tb3+ (c), CaWO4:4%Tb3+,2%Eu3+ (d), CaWO4:6%Tb3+,1%Eu3+ (e) samples. The standard data of CaWO4 (JCPDS no. 41-1431) is shown as a reference. | |
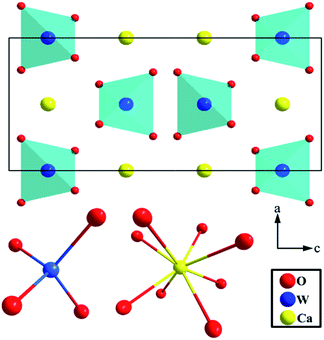 |
| Fig. 2 The unit cell structure of CaWO4 and the coordination environment of cations in the host lattice. | |
3.2 Photoluminescence properties analysis
Fig. 3 illustrates the PLE and PL spectra of as-prepared CaWO4, CaWO4:Eu3+, and CaWO4:Tb3+ samples, respectively. The excitation spectrum of CaWO4 (Fig. 3a) contains a broad band from 200 to 300 nm with a maximum at 248 nm, which is ascribed to the charge-transfer absorption from the oxygen ligands to the central tungsten atoms inside the WO42− complex.40 Upon excitation at 248 nm, the emission spectrum of pure CaWO4 phosphors displays a broad band ranging from 320 to 600 nm with a maximum at 415 nm. The excitation and emission spectra obtained are mainly owing to the charge transfer absorption within the WO42− groups. From the viewpoint of molecular orbital theory, these bands in the excitation and emission spectra can be attributed to the transitions from the 1A1 ground state to the high vibration level of 1B (1T2) and from the low vibration level of 1B (1T2) to the 1A1 ground state within the WO42− ion.41 Fig. 3b presents the PL excitation and emission spectra of CaWO4:2%Eu3+ phosphors. When monitoring by the red emission of Eu3+ (617 nm, 5D0 → 7F2), the PLE spectrum of CaWO4:2%Eu3+ reveals a broad band ranging from 200 to 300 nm ascribed to the combined absorptions of host and Eu3+–O2− charge transfer transition.42 In addition, the narrow lines beyond 300 nm are the characteristic f–f transitions (320 nm, 7F0 → 5H6; 363 nm, 7F0 → 5D4; 383 nm, 7F0 → 5G2; 395 nm, 7F0 → 5L6; 417 nm, 7F0 → 5D3) of Eu3+ within its 4f6 configuration.43 Upon excitation at 276 nm, both the wide host emission band and Eu3+ 4f–4f characteristic transition emission lines appear, namely, 5D0,1 excited states to the 7FJ ground states including 5D0 → 7F1 (594 nm), 5D0 → 7F2 (617 nm), 5D0 → 7F3 (657 nm) and 5D0 → 7F4 (706 nm), respectively.44 It is well-known that Eu3+ is an outstanding structural probe for exploring the local environment in a host lattice. The electric dipole transition 5D0 → 7F2 is a hypersensitive one, which is strongly influenced by the chemical environment around the Eu3+ ions. When Eu3+ ions are located at a low symmetry site, the 5D0 → 7F2 transition often dominates in the emission spectrum. Nevertheless, the magnetic dipole transition (5D0 → 7F1) is independent from the local crystal field environment.45 From the results in Fig. 3b, the emission intensity of the electric dipole transition is considerably higher than that of the magnetic dipole transition, implying that the Eu3+ ions occupy the sites without inversion symmetry, which is consistent with the crystal structure of CaWO4.
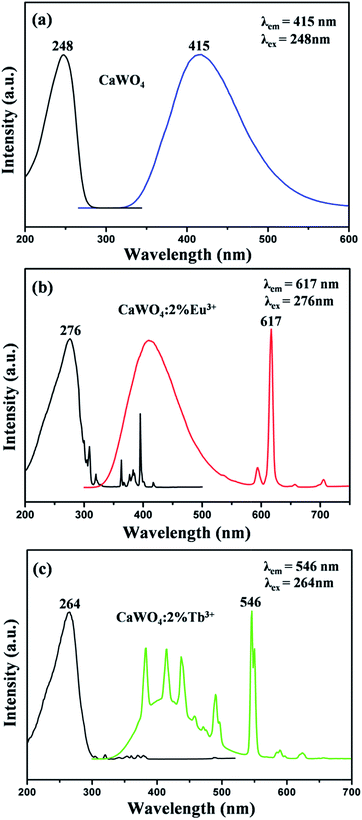 |
| Fig. 3 PL excitation and emission spectra of CaWO4 (a), CaWO4:2%Eu3+ (b) and CaWO4:2%Tb3+ (c) samples. | |
As for CaWO4:2%Tb3+ phosphors, the excitation spectra monitoring the emission at 546 nm corresponding to the 5D4 → 7F5 transition of Tb3+ is shown in Fig. 3c. It can be seen that the excitation spectrum of CaWO4:2%Tb3+ consists of a strong broad band from 200 to 300 nm with a maximum at 264 nm due to the WO42− groups as described above, and the other weak lines in longer wavelength region are attributed to the f–f transition within the Tb3+ 4f8 configuration.46 Upon excitation into the WO42− group at 264 nm, not only the broad host emission but also the Tb3+ 4f–4f transition emission lines [383 nm (5D3 → 7F6), 415 nm (5D3 → 7F5), 437 nm (5D3 → 7F4), 490 nm (5D4 → 7F6), 546 nm (5D4 → 7F5), 590 nm (5D4 → 7F4), and 624 nm (5D4 → 7F3) for Tb3+ ion] can be clearly observed.47 Moreover, it is worth noting that the presence of the excitation peak of the WO42− groups in the excitation spectrum of Eu3+/Tb3+ indicates that there is an energy transfer from the WO42− groups of host to Eu3+/Tb3+ ions.48
In addition, we can deduce that the energy transfer primarily takes place from the WO42− groups of host to Eu3+/Tb3+ by the spectral overlaps between the emission bands of host and the characteristic excitation peaks of Eu3+/Tb3+ ions in phosphors, as shown in Fig. 4. Fig. 5 illustrates the decay curves of activators characteristic emissions in CaWO4:4%Eu3+ (λex = 276 nm, λem = 617 nm) and CaWO4:4%Tb3+ (λex = 264 nm, λem = 546 nm), which can be well fitted with a single exponential formula as follows:49–51
|
I = I0 exp(−t/τ)
| (1) |
where
I0 and
I are the luminescence intensities at time 0 and
t, respectively, and
τ is the decay lifetime. The value of lifetimes were determined to be 552.7 and 621.8 μs for Eu
3+ and Tb
3+, respectively, which are consistent with the previous results reported in the literature
6,52 and much longer than that of the host emission below in
Fig. 7. These results illustrate that the Eu
3+ and Tb
3+ ions not only can be accommodated into this type of host but also they can present their characteristic emission spectra.
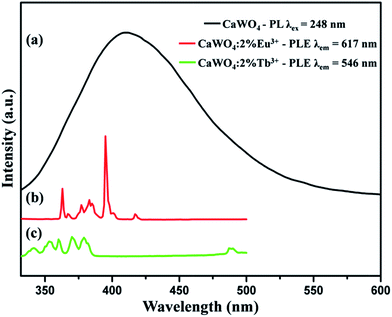 |
| Fig. 4 PL emission spectrum of CaWO4 (a) and PL characteristic excitation spectra of activators in CaWO4:2%Eu3+/2%Tb3+ (b and c). | |
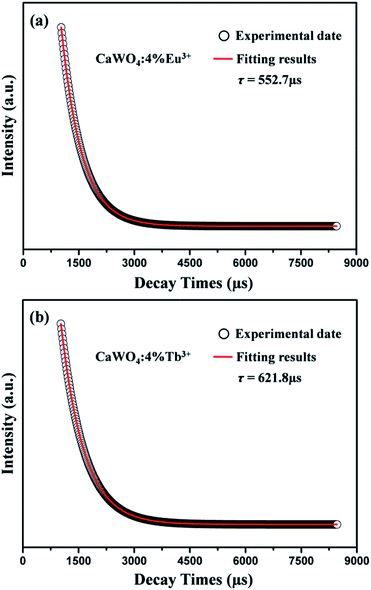 |
| Fig. 5 The decay curves for the luminescence of Eu3+ ions in CaWO4:4%Eu3+ samples (a) and (b) Tb3+ ions in CaWO4:4%Tb3+ samples. | |
The variations in the emission spectra as a function of Eu3+/Tb3+ concentration in CaWO4:x%Eu3+/y%Tb3+ (x = 0–20, y = 0–10) are shown in Fig. 6. In the CaWO4:x%Eu3+ system, the emission intensity of the host decreases sharply with increasing Eu3+ concentration under the excitation of 276 nm UV. However, the emission intensity of Eu3+ initially increases until the Eu3+ content becomes 8%, and subsequently descends with further increasing the Eu3+ concentration due to the concentration quenching effect as shown in Fig. 6a. This phenomenon can confirm the existence of energy transfer from the host to the activators in CaWO4:Eu3+ samples. In order to provide further evidence to validate the energy transfer from the host to Eu3+ ions in CaWO4:x%Eu3+, we investigated the decay curves and lifetimes of host (λex = 276 nm, λem = 415 nm) emission with the increasing Eu3+ (x = 0, 1, 4, 6, 8) concentration in CaWO4:xEu3+ (Fig. 7a). The decay curves can be well fitted using a second-order exponential function as given by the following equation:53–56
|
I = A1 exp(−t/τ1) + A2 exp(−t/τ2)
| (2) |
where
I represents the luminescent intensity;
A1 and
A2 are constants;
t is the time;
τ1 and
τ2 are short- and long-decay components, respectively. According to these parameters, the average decay times (
τ*) can be determined by the following equation:
|
τ* = (A1τ12 + A2τ22)/(A1τ1 + A2τ2)
| (3) |
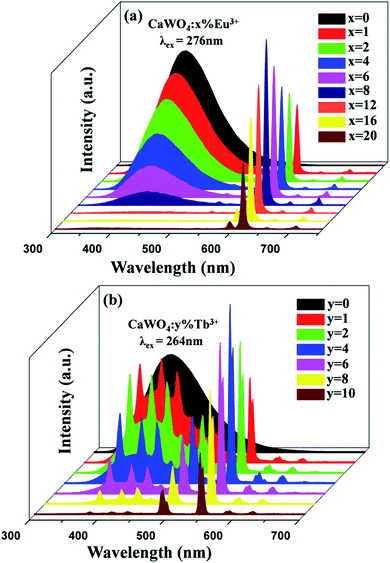 |
| Fig. 6 PL spectra of CaWO4:x%Eu3+ and CaWO4:y%Tb3+ samples as a function of (a) Eu3+ and (b) Tb3+ doping concentrations, respectively. | |
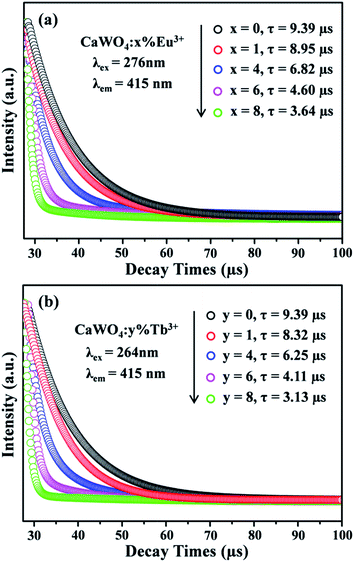 |
| Fig. 7 Decay curves of host emission in (a) CaWO4:xEu3+ samples with different Eu3+ doping concentrations (b) CaWO4:yTb3+ samples with different Tb3+ doping concentrations. | |
On the basis of eqn (3), the average lifetimes of host are determined to be 9.39, 8.95, 6.82, 4.60 and 3.64 μs for Eu3+ concentrations of 0%, 1%, 4%, 6%, and 8%, respectively. The declined decay times of host with increasing Eu3+ concentration strongly certifies the occurrence of energy transfer from host to Eu3+ in CaWO4:Eu3+ phosphors. Also, such energy transfer phenomenon demonstrates that the as-synthesized CaWO4:xEu3+ is a solid solution, in which Eu3+ has successfully incorporated into CaWO4 lattice,57 agreeing well with the XRD results. The efficiency (ηT) of energy transfer from the host to Eu3+ in CaWO4:x%Eu3+ system can be calculated using the following equation:58
where
ηT is the energy transfer efficiency, and
IS0 and
IS are the luminescence intensity of the host in the absence and presence of an activator (Eu
3+), respectively. As shown in
Fig. 8a, the
ηT value from host to activators in CaWO
4:
x%Eu
3+ system is plotted as a function of concentrations of Eu
3+ ions, which show that the
ηT value monotonously increase to reach maximums at 98% under 276 nm UV radiation, indicating the energy transfer from the host to Eu
3+ becomes more and more efficient with the increasing concentration of Eu
3+ ions.
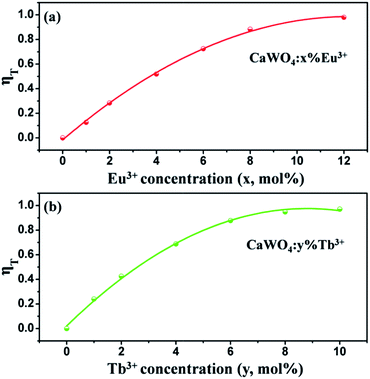 |
| Fig. 8 Energy transfer efficiency from (a) host to Eu3+ in CaWO4:x%Eu3+ samples and (b) host to Tb3+ in CaWO4:y%Tb3+ samples. | |
In order to determine the energy transfer mechanism from host to activators in CaWO4:x%Eu3+ phosphors, it is necessary to know the critical distance (Rc) between the neighbouring Eu3+ ions. According to Blasse,59,60 the critical distance can be expressed as
|
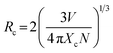 | (5) |
where
V represents the volume of the unit cell,
N refers to the number of the host cations in the unit cell, and
Xc is the critical concentration of the activator. For CaWO
4 host,
N = 4 and
V = 312.63 Å
3,
Xc is 0.08 for Eu
3+; therefore, the critical distance (
Rc) was calculated to be about 12.32 Å. With the increase of Eu
3+ content, the energy transfer from the host to Eu
3+ becomes more efficient, and the probability of energy migration between activators increases simultaneously. When the distance becomes small enough, the concentration quenching phenomenon takes place and the energy migration is hindered. In general, there are two main mechanisms for a non-radiative energy transfer process: one is exchange interaction, and the other is electric multipolar interactions.
61 The value of
Rc acquired above indicates the little possibility of exchange interaction since it can be predominant only for about 5 Å.
62–64 Therefore, we can infer that the energy transfer mechanism from the host to Eu
3+ is dominated by the electric multipolar interactions. On the basis of Dexter's energy transfer formula of multipolar interactions and Reisfeld's approximation, the following relationship can be attained:
65,66 |
 | (6) |
where
ηS0 and
ηS represent the luminescence quantum efficiency of the host in the absence and presence of Eu
3+ ions, respectively.
C is the concentration of the Eu
3+ ions. The value for
α = 6, 8, and 10 corresponds to dipole–dipole, dipole–quadrupole, and quadrupole–quadrupole interactions, respectively. The values of
ηS0/
ηS also can be approximately calculated by the ratio of related luminescence intensities as
67–70 |
 | (7) |
where
IS0 and
IS stand for the luminescence intensity of the host without and with the Eu
3+ ions, respectively. The relationship between
IS0/IS and
Cα/3 according to the above equation are shown in
Fig. 9. It is obvious that a linear relationship can be observed only when
α = 6, implying that the energy transfer from the host to Eu
3+ ions takes place through the dipole–dipole interaction mechanism.
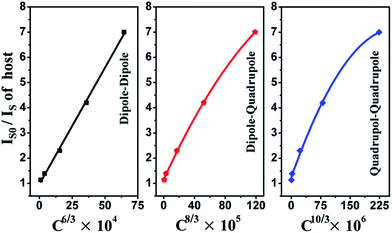 |
| Fig. 9 Dependence of IS0/IS of host emission on (a) C6/3Eu, (b) C8/3Eu, and (c) C10/3Eu. | |
Similarly, Tb3+ ions could also be sensitized by host transition through energy transfer process as confirmed by the PL spectra of CaWO4:y%Tb3+ shown in Fig. 6b and the decay lifetimes presented in Fig. 7b. The maximal energy transfer efficiency from host to Tb3+ ions is 97%. The critical distance (Rc) was calculated to be about 15.51 Å, and the energy transfer mechanism from the host to Tb3+ ions is also dipole–dipole interaction (Fig. S1, ESI†).
In order to enrich the emission colors, the Eu3+ and Tb3+ codoped CaWO4 phosphors were synthesized. Fig. 10 shows the PL emission spectra for CaWO4:4%Tb3+,x%Eu3+ (x = 0, 1, 2, 4, 6, 8, 10) and CaWO4:1%Eu3+,y%Tb3+ (y = 0, 1, 2, 4, 6) upon 264 nm UV excitation. We can observe that the intensities of host and Tb3+ emissions in CaWO4:4%Tb3+,x%Eu3+ both decreases monotonously with an increase in the Eu3+ content, whereas the emission intensity of Eu3+ first increases and saturates at x = 8, as depicted in Fig. 10a, which illustrates the energy transfers from the host and Tb3+ to Eu3+ ions can take place. Energy transfer between Tb3+ and Eu3+ is a well-known phenomenon since the 5D4 → 7F6,5,4,3 emissions of Tb3+ are effectively overlapped with the 7F0,1 → 5D0,1,2 absorptions of Eu3+.16,52 In addition, the decrease in the lifetimes of Tb3+ with increasing Eu3+ concentration in CaWO4:4%Tb3+,x%Eu3+ system strongly demonstrates the existence of an energy transfer process from Tb3+ to Eu3+ ions (Fig. S2, ESI†). In CaWO4:1%Eu3+,y%Tb3+ system, the host emission intensity decreases monotonously, while the emission intensity of Tb3+ increases gradually with an increase in Tb3+ concentration (Fig. 10b). However, the intensity of Eu3+ emission varies little. The result suggests that the energy transfer from the host to Tb3+ is more apt to that from Tb3+ to Eu3+ ions in these phosphors.
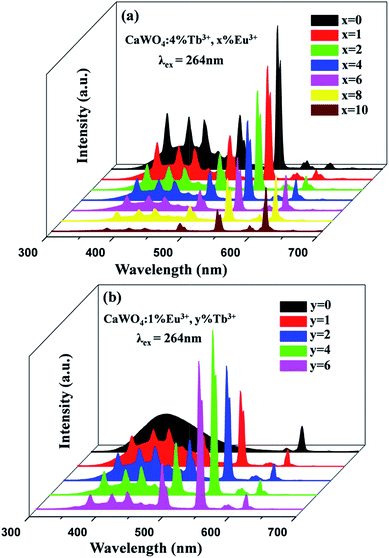 |
| Fig. 10 PL emission spectra of (a) CaWO4:4%Tb3+,x%Eu3+ and (b) CaWO4:1%Eu3+,y%Tb3+ samples as a function of Eu3+ and Tb3+ concentration, respectively. | |
Table 1 summarizes the CIE chromaticity coordinates of CaWO4:Eu3+/Tb3+ and CaWO4:Eu3+,Tb3+ samples. All the values were calculated on the basis of their corresponding PL spectrum. The CIE chromaticity coordinates of CaWO4:Eu3+/Tb3+ phosphors can vary from (0.149, 0.100) for CaWO4 host to (0.625, 0.307) for CaWO4:20%Eu3+ and (0.255, 0.560) for CaWO4:10%Tb3+, which correspond to the color changes from blue to red and green, respectively. In CaWO4:4%Tb3+,x%Eu3+ system, the CIE chromaticity coordinate varies from (0.212, 0.302) to (0.434, 0.383) corresponding to x = 0 and 10, respectively. In particular, the CIE chromaticity coordinates for CaWO4:4%Tb3+,8%Eu3+ is (0.320, 0.324), which is very close to the standard value of white emission (0.323, 0.333). In CaWO4:1%Eu3+,y%Tb3+ system, the CIE coordinate shifts from (0.197, 0.109) to (0.277, 0.462) corresponding to y = 0 and 6, respectively. For the practical applications, the quantum yield (QY) is an important parameter for phosphors. Therefore, the QY of these representative phosphors, CaWO4 (blue emission), CaWO4:10%Tb3+ (green emission), CaWO4:20%Eu3+ (red emission) and CaWO4:4%Tb3+,8%Eu3+ (white emission), are measured. The value of QY is 31.9% for CaWO4, 24.6% for CaWO4:10%Tb3+, 20.3% for CaWO4:20%Eu3+ and 17.2% for CaWO4:4%Tb3+,8%Eu3+. It is believed that the QY of these phosphors could be further improved by optimizing the synthesis conditions and chemical composition. The variety of CIE chromaticity coordinates for the as-prepared samples and digital luminescence photographs of representative samples upon UV lamp excitation at 254 nm are illustrated in Fig. 11, which indicate that the tunable color can be realized by doping different activators and controlling the doping concentration of the activators. These results indicate that the as-obtained products emerge the advantage of multicolor emissions in the visible region and have potential applications in solid-state lighting fields.
Table 1 CIE chromaticity coordinates for CaWO4:x%Tb3+,y%Eu3+ samples
Sample no. |
CaWO4:x%Eu3+/y%Tb3+ |
Excitation |
CIE (x, y) |
1 |
x = 0 |
248 |
(0.149, 0.100) |
2 |
x = 1 |
276 |
(0.197, 0.109) |
3 |
x = 2 |
276 |
(0.222, 0.117) |
4 |
x = 4 |
276 |
(0.265, 0.135) |
5 |
x = 6 |
276 |
(0.350, 0.179) |
6 |
x = 8 |
276 |
(0.486, 0.247) |
7 |
x = 12 |
276 |
(0.613, 0.301) |
8 |
x = 20 |
276 |
(0.625, 0.307) |
9 |
y = 1 |
264 |
(0.175, 0.149) |
10 |
y = 2 |
264 |
(0.187, 0.199) |
11 |
y = 4 |
264 |
(0.212, 0.302) |
12 |
y = 6 |
264 |
(0.236, 0.407) |
13 |
y = 8 |
264 |
(0.251, 0.542) |
14 |
y = 10 |
264 |
(0.255, 0.560) |
Sample no. |
CaWO4:4%Tb3+/x%Eu3+ |
Excitation |
CIE (x, y) |
11 |
x = 0 |
264 |
(0.212, 0.302) |
15 |
x = 1 |
264 |
(0.229, 0.337) |
16 |
x = 2 |
264 |
(0.245, 0.367) |
17 |
x = 4 |
264 |
(0.257, 0.346) |
18 |
x = 6 |
264 |
(0.294, 0.329) |
19 |
x = 8 |
264 |
(0.320, 0.324) |
20 |
x = 10 |
264 |
(0.434, 0.383) |
Sample no. |
CaWO4:1%Eu3+/y%Tb3+ |
Excitation |
CIE (x, y) |
2 |
y = 0 |
264 |
(0.197, 0.109) |
21 |
y = 1 |
264 |
(0.230, 0.232) |
22 |
y = 2 |
264 |
(0.251, 0.303) |
23 |
y = 4 |
264 |
(0.269, 0.401) |
24 |
y = 6 |
264 |
(0.277, 0.462) |
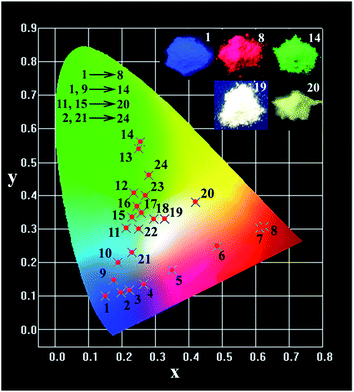 |
| Fig. 11 The variety of the CIE chromaticity coordinates of the as-prepared phosphors. The insets are digital photographs of representative samples upon 254 nm UV lamp box excitation. | |
4. Conclusions
In summary, a series of Eu3+ and Tb3+ singly-doped and co-doped CaWO4:Eu3+/Tb3+ phosphors have been prepared by high-temperature solid-state reaction method. Under UV excitation, the CaWO4 host can emit an intense blue light. When Eu3+/Tb3+ were singly doped into the host, the tunable emission colors occur attributed to the energy transfer from the host to the activators. The monotonous decreases in the lifetimes of host with increasing activator concentrations strongly certify the existence of energy transfer from the host to activators in CaWO4:Eu3+/Tb3+. The energy transfer mechanism from the host to Eu3+ and Tb3+ in CaWO4:Eu3+/Tb3+ phosphors has been determined to be a resonant type via dipole–dipole interaction. Moreover, by adjusting the concentration ratio of Eu3+ and Tb3+ ions, a white emission in a single-composition was realized by blending simultaneously the blue, green, and red emissions of host, Tb3+, and Eu3+ in the CaWO4:8%Eu3+,4%Tb3+ phosphor. These results indicate that the as-prepared CaWO4:Eu3+, CaWO4:Tb3+, and CaWO4:Eu3+,Tb3+ phosphors have potential application in solid state-lighting technology.
Acknowledgements
This work was supported by the National Natural Science Foundation of China (21476045) and the Excellent talents plan project for universities of Liaoning province (LJQ2014055).
Notes and references
- M. K. Tsang, G. Bai and J. Hao, Chem. Soc. Rev., 2015, 44, 1585 RSC.
- Y. T. Tsai, C. Y. Chiang, W. Zhou, J. F. Lee, H. S. Sheu and R. S. Liu, J. Am. Chem. Soc., 2015, 137, 8936 CrossRef CAS PubMed.
- W. Lu, Y. Jia, Q. Zhao, W. Lv and H. You, Chem. Commun., 2014, 50, 2635 RSC.
- C. Zhang, L. Yang, J. Zhao, B. Liu, M. Y. Han and Z. Zhang, Angew. Chem., Int. Ed., 2015, 54, 11531 CrossRef CAS PubMed.
- M. K. Tsang, C. F. Chan, K. L. Wong and J. Hao, J. Lumin., 2015, 157, 172 CrossRef CAS.
- K. Li, X. Liu, Y. Zhang, X. Li, H. Lian and J. Lin, Inorg. Chem., 2014, 54, 323 CrossRef PubMed.
- S. P. Lee, C. H. Huang, T. S. Chan and T. M. Chen, ACS Appl. Mater. Interfaces, 2014, 6, 7260 CAS.
- X. Huang, Z. Lin, Z. Hu, L. Zhang, J. Huang and G. Wang, J. Cryst. Growth, 2004, 269, 401 CrossRef CAS.
- W. B. Im, N. George, J. Kurzman, S. Brinkley, A. Mikhailovsky, J. Hu, B. F. Chmelka, S. P. DenBaars and R. Seshadri, Adv. Mater., 2011, 23, 2300 CrossRef CAS PubMed.
- M. M. Shang, C. X. Li and J. Lin, Chem. Soc. Rev., 2014, 43, 1372 RSC.
- Y. Jia, R. Pang, H. Li, W. Sun, J. Fu, L. Jiang, S. Zhang, Q. Su, C. Li and R. S. Liu, Dalton Trans., 2015, 44, 11399 RSC.
- H. Zhou, Q. Wang and Y. Jin, J. Mater. Chem. C, 2015, 3, 11151 RSC.
- J. Zhou and Z. Xia, J. Mater. Chem. C, 2015, 3, 7552 RSC.
- M. D. Weber, L. Niklaus, M. Proschel, P. B. Coto, U. Sonnewald and R. D. Costa, Adv. Mater., 2015, 27, 5493 CrossRef CAS PubMed.
- J. Ding, Q. Wu, Y. Li, Q. Long, C. Wang and Y. Wang, Dalton Trans., 2015, 44, 9630 RSC.
- M. Shang, G. Li, X. Kang, D. Yang, D. Geng and J. Lin, ACS Appl. Mater. Interfaces, 2011, 3, 2738 CAS.
- J. Yu, W. Gong, Y. Zhang and G. Ning, J. Lumin., 2014, 147, 250 CrossRef CAS.
- X. Li, J. D. Budai, F. Liu, J. Y. Howe, J. Zhang, X. J. Wang, Z. Gu, C. Sun, R. S. Meltzer and Z. Pan, Light: Sci. Appl., 2013, 2, e50 CrossRef.
- Q. Dai, M. E. Foley, C. J. Breshike, A. Lita and G. F. Strouse, J. Am. Chem. Soc., 2011, 133, 15475 CrossRef CAS PubMed.
- H. Liu, T. Chu, Z. Rao, S. Wang, Y. Yang and W. T. Wong, Adv. Opt. Mater., 2015, 3, 1545 CrossRef CAS.
- X. Zhang and M. Gong, Ind. Eng. Chem. Res., 2015, 54, 7632 CrossRef CAS.
- M. Zhang, Y. Liang, R. Tang, D. Yu, M. Tong, Q. Wang, Y. Zhu, X. Wu and G. Li, RSC Adv., 2014, 4, 40626 RSC.
- P. Huang, W. Zheng, S. Zhou, D. Tu, Z. Chen, H. Zhu, R. Li, E. Ma, M. Huang and X. Chen, Angew. Chem., Int. Ed., 2014, 53, 1252 CrossRef CAS PubMed.
- P. Chen, Q. Li, S. Grindy and N. Holten-Andersen, J. Am. Chem. Soc., 2015, 137, 11590 CrossRef CAS PubMed.
- X. Liu, W. Hou, X. Yang and J. Liang, CrystEngComm, 2014, 16, 1268 RSC.
- Y. Liu, X. Zhang, Z. Hao, X. Wang and J. Zhang, Chem. Commun., 2011, 47, 10677 RSC.
- S. Xu, P. Li, Z. Wang, T. Li, Q. Bai, J. Sun and Z. Yang, J. Mater. Chem. C, 2015, 3, 9112 RSC.
- C. Zeng, Y. Hu, Z. Xia and H. Huang, RSC Adv., 2015, 5, 68099 RSC.
- A. M. Kaczmarek and R. Van Deun, Chem. Soc. Rev., 2013, 42, 8835 RSC.
- D. Yue, Q. Li, W. Lu, Q. Wang, M. Wang, C. Li, L. Jin, Y. Shi, Z. Wang and J. Hao, J. Mater. Chem. C, 2015, 3, 2865 RSC.
- A. M. Kaczmarek, D. Ndagsi, I. Van Driessche, K. Van Hecke and R. Van Deun, Dalton Trans., 2015, 44, 10237 RSC.
- M. Yin, Y. Liu, L. Mei, M. S. Molokeev, Z. Huang and M. Fang, RSC Adv., 2015, 5, 73077 RSC.
- Z. Hou, Z. Cheng, G. Li, W. Wang, C. Peng, C. Li, P. Ma, D. Yang, X. Kang and J. Lin, Nanoscale, 2011, 3, 1568 RSC.
- M. Maheshwary, B. P. Singh and R. A. Singh, New J. Chem., 2015, 39, 4494 RSC.
- Y. Zhang, W. Gong, J. Yu, H. Pang, Q. Song and G. Ning, RSC Adv., 2015, 5, 62527 RSC.
- M. V. Nazarov, D. Y. Jeon, J. H. Kang, E. J. Popovici, L. E. Muresan, M. V. Zamoryanskaya and B. S. Tsukerblat, Solid State Commun., 2004, 131, 307 CrossRef CAS.
- Y. Zheng, Y. Huang, M. Yang, N. Guo, H. Qiao, Y. Jia and H. You, J. Lumin., 2012, 132, 362 CrossRef CAS.
- Q. Xiao, Q. Zhou and M. Li, J. Lumin., 2010, 130, 1092 CrossRef CAS.
- L. S. Cavalcante, V. M. Longo, J. C. Sczancoski, M. A. P. Almeida, A. A. Batista, J. A. Varela, M. O. Orlandi, E. Longo and M. S. Li, CrystEngComm, 2012, 14, 853 RSC.
- J. Han, C. McBean, L. Wang, C. Jaye, H. Liu, D. A. Fischer and S. S. Wong, J. Phys. Chem. C, 2015, 119, 3826 CAS.
- F. Kang and M. Peng, Dalton Trans., 2014, 43, 277 RSC.
- C. Li, C. Lin, X. Liu and J. Lin, J. Nanosci. Nanotechnol., 2008, 8, 1183 CAS.
- B. Wei, Z. Liu, C. Xie, S. Yang, W. Tang, A. Gu, W. T. Wong and K. L. Wong, J. Mater. Chem. C, 2015, 3, 12322 RSC.
- Y. C. Chen, Y. C. Wu, D. Y. Wang and T. M. Chen, J. Mater. Chem., 2012, 22, 7961 RSC.
- Y. Liu, Y. Wang, L. Wang, Y. Y. Gu, S. H. Yu, Z. G. Lu and R. Sun, RSC Adv., 2014, 4, 4754 RSC.
- Z. Y. Hou, C. X. Li, J. Yang, H. Z. Lian, P. P. Yang, R. T. Chai, Z. Y. Cheng and J. Lin, J. Mater. Chem., 2009, 19, 2737 RSC.
- M. Jiao, W. Lv, W. Lü, Q. Zhao, B. Shao and H. You, ChemPhysChem, 2015, 16, 817 CrossRef CAS PubMed.
- Y. Liu, G. Liu, J. Wang, X. Dong and W. Yu, Inorg. Chem., 2014, 53, 11457 CrossRef CAS PubMed.
- G. Blasse and B. C. Grabmarier, Luminescent Materials, Springer-Verlag, Berlin, 1994 Search PubMed.
- G. Li, Y. Zhang, D. Geng, M. Shang, C. Peng, Z. Cheng and J. Lin, ACS Appl. Mater. Interfaces, 2012, 4, 296 CAS.
- Y. Jia, H. Qiao, Y. Zheng, N. Guo and H. You, Phys. Chem. Chem. Phys., 2012, 14, 3537 RSC.
- K. Li, Y. Zhang, X. Li, M. Shang, H. Lian and J. Lin, Phys. Chem. Chem. Phys., 2015, 17, 4283 RSC.
- J. Zhou and Z. Xia, J. Mater. Chem. C, 2015, 3, 7552 RSC.
- Z. Xia, S. Miao, M. Chen, M. S. Molokeev and Q. Liu, Inorg. Chem., 2015, 54, 7684 CrossRef CAS PubMed.
- Y. Zhang, W. Gong, J. Yu, Y. Lin and G. Ning, RSC Adv., 2015, 5, 96272 RSC.
- J. Wang, H. Zhang, B. Lei, Z. Xia, H. Dong, Y. Liu, M. Zheng and Y. Xiao, J. Mater. Chem. C, 2015, 3, 4445 RSC.
- W. H. Di, X. J. Wang, P. F. Zhu and B. J. Chen, J. Solid State Chem., 2007, 180, 467 CrossRef CAS.
- C. H. Huang, T. W. Kuo and T. M. Chen, ACS Appl. Mater. Interfaces, 2010, 2, 1395 CAS.
- G. Blasse, J. Solid State Chem., 1986, 62, 207 CrossRef CAS.
- W. R. Liu, C. H. Huang, C. W. Yeh, J. C. Tsai, Y. C. Chiu, Y. T. Yeh and R. S. Liu, Inorg. Chem., 2012, 51, 9636 CrossRef CAS PubMed.
- Y. Zhang, D. L. Geng, X. J. Li, J. Fan, K. Li, H. Z. Lian, M. M. Shang and J. Lin, J. Phys. Chem. C, 2014, 118, 17983 CAS.
- D. L. Dexter, J. Chem. Phys., 1953, 21, 836 CrossRef CAS.
- W. R. Liu, C. H. Huang, C. P. Wu, Y. C. Chiu, Y. T. Yeh and T. M. Chen, J. Mater. Chem., 2011, 21, 6869 RSC.
- K. Li, J. Xu, X. Cai, J. Fan, Y. Zhang, M. Shang, H. Lian and J. Lin, J. Mater. Chem. C, 2015, 3, 6341 RSC.
- D. L. Dexter and J. A. Schulman, J. Chem. Phys., 1954, 22, 1063 CrossRef CAS.
- G. Blasse, Philips Res. Rep., 1969, 24, 131 CAS.
- P. I. Paulose, G. Jose, V. Thomas, N. V. Unnikrishnan and M. K. R. Warrier, J. Phys. Chem. Solids, 2003, 64, 841 CrossRef CAS.
- M. Chen, Z. Xia, M. S. Molokeev and Q. Liu, J. Mater. Chem. C, 2015, 3, 12477 RSC.
- M. Jiao, Y. Jia, W. Lü, W. Lv, Q. Zhao, B. Shao and H. You, J. Mater. Chem. C, 2014, 2, 90 RSC.
- P. Li, Z. Wang, Q. Guo and Z. Yang, RSC Adv., 2015, 5, 4448 RSC.
Footnote |
† Electronic supplementary information (ESI) available: The decay curves of Tb3+ in CaWO4:4%Tb3+,x%Eu3+ with changing Eu3+ concentrations, and dependence of IS0/IS of host emission on (a) C6/3Tb, (b) C8/3Tb, and (c) C10/3Tb respectively. See DOI: 10.1039/c6ra01862a |
|
This journal is © The Royal Society of Chemistry 2016 |
Click here to see how this site uses Cookies. View our privacy policy here.