DOI:
10.1039/C6RA00618C
(Paper)
RSC Adv., 2016,
6, 28512-28521
ZLD1122, a novel EZH2 and EZH1 small molecular inhibitor, blocks H3K27 methylation and diffuse large B cell lymphoma cell growth
Received
8th January 2016
, Accepted 2nd March 2016
First published on 3rd March 2016
Abstract
The histone methyltransferase enhancer of zeste homolog 2 (EZH2) has been reported to be overexpressed in a variety of cancers and is associated with tumor malignancy. This is mainly because EZH2 catalyzes the hypertrimethylation of histone 3 at lysine 27 (H3K27) at the promoter of target genes, leading to the silencing of downstream tumor suppressor genes. Hence, blocking its catalytic function may be a therapeutic strategy for the treatment of tumors which over-express or have a gain-of-function mutation in EZH2, such as lymphomas. Here, we reported a novel, selective, small-molecule inhibitor of EZH2 and EZH1 synthesized by us, ZLD1122, which inhibited both EZH1 and wild type and mutant EZH2 activities with nanomolar potency. ZLD1122 significantly inhibited intracellular H3K27 trimethylation without affecting the levels of H3, H3K9me3, and H3K4me3, indicating its selective inhibition of polycomb repressive complex 2 (PRC2) methyl catalytic function. Moreover, ZLD1122 induced G0/G1 phase arrest in diffuse large B cell lymphoma (DLBCL) cells in a dose-dependent manner via downregulation of cyclinE and CDK4 as well as upregulation of p21 and cyclinD1. Furthermore, it induced apoptosis and loss of mitochondrial membrane potential (Δψm), and elevated the levels of cleaved caspase-9 in Su-DHL-6 and Pfeiffer cells, suggesting that ZLD1122 suppresses the viability of DLBCL cells by inducing caspase-mediated intrinsic apoptosis. Taken together, these data demonstrated that ZLD1122, owing to its pharmacologically inhibitory activity against EZH2, could be a promising agent for the treatment of lymphomas with EZH2 gain-of-function mutations.
1. Introduction
The importance of chromatin-associated proteins and post-translational modifications of histones has been demonstrated by a plethora of studies.1 Particularly, methylation events at lysine and arginine residues, catalyzed by histone methyltransferases (HMTs), have been subjected to intense investigations in recent years. This is because of their critical role in regulating chromatin structure and gene expression.2 PRC2 is a highly conserved HMT that methylates H3K27, leading to the transcriptional repression of target downstream genes involved in cell differentiation, proliferation, and cell cycle regulation.3 PRC2 contains the enzymatic subunit EZH2 or EZH1.4,5 EZH2 has been widely implicated for its correlation with poor prognosis and disease stage. However, studies using EZH2-null embryonic stem cells (ESCs) demonstrated the existence of residual H3K27me3, and discovered the presence of the methyltransferase EZH1. These findings suggest that these two enzymes can, at least partially, compensate for each other. In general, genetic loss of EZH2 has less severe consequences than the loss of other PRC2 components, owing to the compensatory presence of EZH1.6 Thus, an inhibitor targeting both EZH1 and EZH2 may work well in the abrogation of redundant H3K27me3 and be a bona fide PRC2 catalytic inhibitor.7
Non-Hodgkin’s Lymphoma (NHL) is a common aggressive hematological cancer, which has various subtypes. Most of the NHLs develop from the progressive differentiation of germinal center B cells. Diffuse large B-cell lymphoma (DLBCL) is the most common subtype of NHLs and accounts for 47% of NHLs.8 Although the Bruton’s tyrosine kinase (BTK) inhibitor ibrutinib has been used successfully for the treatment of several kinds of NHL,9 its effect in DLBCL was unsatisfactory. On the other hand, many NHL patients respond to the current standard combination therapy of rituximab, cyclophosphamide, hydroxydaunorubicin, oncovin (vincristine), and prednisone (R-CHOP).10,11 However, most of these agents are nonspecific and are associated with side effects such as nausea, vomiting, neutropenia, alopecia, and hemorrhagic cystitis. Therefore, there is an urgent need to develop a new-generation of anti-NHL agents. In DLBCL, heterozygous missense mutations frequently occur at amino acids Y641N, Y641F, and A677G, within the SET domain of EZH2. This may be correlated to EZH2 overexpression.12 Since overexpression of wild-type or mutant EZH2 is frequently detected in DLBCL13 and DLBCL cell lines with mutant EZH2 are dependent on EZH2 activity for cell growth, the overexpression of EZH2 might serve as a biomarker of aggressive DLBCL with poor prognosis, and is a promising therapeutic target for DLBCL treatment.
Our laboratory developed a novel EZH2 and EZH1 small-molecule inhibitor, 5-(cyclohexyl(ethyl)amino)-N-((1,7-dimethyl-3-oxo-2,3,5,6,7,8-hexahydroisoquinolin-4-yl)methyl)-4-methyl-4′-((4-methylpiperazin-1-yl)methyl)biphenyl-3-carboxamide (ZLD1122),14 which significantly inhibited EZH1 and EZH2 activities among a panel of HMTs. Moreover, ZLD1122 inhibited a diverse panel of cancer cell lines, among which NHL cells were most sensitive to ZLD1122. Because of inhibition of EZH2 activity, ZLD1122 decreased the intracellular protein level of H3K27me3 and H3K27me3 without affecting the level of H3 in DLBCL cells. Therefore, we further investigated the mechanism underlying DLBCL cells’ sensitivity to ZLD1122 treatment. The results of our study revealed that ZLD1122 exhibits promising anticancer efficacy in vitro, by decreasing cell viability, cell cycle arrest, and cell apoptosis.
2. Materials and methods
2.1 Materials
The universal of MTT was 3-(4,5-dimethyl-2-thiazolyl)-2,5-diphenyl-2-H-tetrazolium bromide. The Annexin V-FITC apoptosis detection kit was purchased from KeyGEN Biology Co. Ltd. (Nanjing, China). The antibodies against cleaved-caspase-3, cleaved-caspase-9, Bcl-2 and Bax were obtained from Cell Signaling Technology Company (Beverly, MA), the antibody against GAPDH was purchased from Chengdu Zen BioScience Co. Ltd. (Chengdu, China), the antibodies against H3K27me3 and H3K27me2 were purchased from Merck Millipore Co. Ltd. (Massachusetts, MA, USA), and the antibodies against H3K27me9, H3K27me4 and H3K27me1 were obtained from ABclonal (Boston, MA, USA). The other primary antibodies were purchased from Abcam (Cambridge, MA, USA).
2.2 Preparation of ZLD1122
The ZLD1122 (Fig. 2G) was synthesized at the State Key Laboratory of Biotherapy, Sichuan University. The compound was synthesized according to Fig. 1. Briefly, treatment of ketone 1 with ethyl acetate under sodium hydride or acetyl chloride under LDA afforded 1,3-dione 2. Then the key building block pyridine-2-(1H)-one 3 was prepared by treating 1,3-dione with cyanoacetamide in the presence of triethylenediamine (DABCO) in refluxing ethanol. Subsequent reduction of 3 by sodium borohydride and iodine afforded amine 4. Compound 7, used for the preparation of acid 8, was synthesized under a two-step reductive amination reaction, starting from methyl 3-amino-5-bromo-2-methylbenzoate 5. Amine 4 was subjected to a coupling reaction with acid 8, affording 9. Next, the title compound 10 was gained by Suzuki coupling reaction with boronic ester.
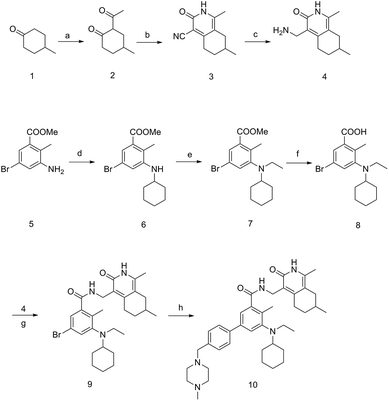 |
| Fig. 1 The synthetic route for and structure of ZLD1122. Reagents and conditions: (a) NaH, EA, PhMe, 0 °C–RT or LDA, acetyl chloride, THF, −78 °C; (b) cyanothioacetamide, DABCO, ethanol, reflux; (c) NaBH4, I2, THF, 0 °C–reflux; (d) sodium triacetoxyborohydride, acetic acid, trichloromethane, 0 °C–RT; (e) sodium triacetoxyborohydride, acetic acid, 1,2-dichloroethane, 0 °C–RT; (f) 3 M NaOH, ethanol, RT, 3 h, then adjust to pH 4–5 with 1 N HCl; (g) EDC, HOAT, NMM, DMSO, RT, 12–48 h; (h) PdCl2(dppf)·CH2Cl2, Na2CO3, 10 : 1 dioxane/H2O, 110 °C. | |
2.3 Biochemical assay
The viabilities of enzymes in the presence of the compound were determined by the AlphaLISA immunodetection assay conducted by an enzyme profiling service provided by Shanghai ChemPartner (Shanghai, China). Each value was determined using an AlphaLISA methyltransferase Assay kit (PerkinElmer, MA, USA) according to the manufacturer’s protocol. All reactions were incubated at room temperature. The enzyme and substrate concentrations are listed in Table 1.
Table 1 The enzyme and substrate concentration of the biochemical assay
Target |
Enzyme conc. (nM) |
Substrate conc. (μM) |
EZH2wt |
3 |
3 |
EZH2(Y641N) |
0.4 |
2 |
EZH2(A677G) |
1 |
4 |
EZH2(Y641F) |
2 |
3 |
EZH1 |
5 |
15 |
DOT1L |
2 |
1 |
G9a |
0.06 |
50 |
SUV39H1 |
6 |
20 |
2.4 Cell culture
Human lymphoma cell lines Pfeiffer and Su-DHL-6 were obtained from American Type Culture Collection (ATCC), along with other cell lines (MDA-MB-231, SMMC-7721, HCT-116, HT-29, HepG2, BT-474, B16-F10, MDA-MB-435S, Jurkat, HBL-1, and OCI-LY-10). The cells were cultured in DMEM or RPMI 1640 medium supplemented with 10% fetal bovine serum and 0.1% amikacin sulfate under humidified conditions with 5% CO2 at 37 °C.
2.5 Cell proliferation assay
Cell viability after treatment with ZLD1122 was measured using a MTT assay and 12 day proliferation assays.15 In the MTT assay, 100 μL of the exponentially growing NHL cells (1–3 × 103 cells per well) were seeded in 96 well plates and cultured for 24 h, then 100 μL of medium with various concentrations of ZLD1122 was added and incubated for 2, 4 and 6 days, respectively. The other cancer cell lines were planted in 96-well plates using the same method.
In a 12 day proliferation assay, 5 × 105 cells per well were seeded in 6 well plates, and the original cells were replanted at the original seeding densities on days 4 and 8. The number of cells per well was counted by a Countstar automated cell counter, and the final number of cells was calculated by multiplied counted numbers.
2.6 Cell cycle and apoptosis analysis by flow cytometry (FCM)
Cell cycle distribution was determined by FCM with PI staining. Briefly, cells were treated with ZLD1122 in various concentrations and for different times, then the treated cells were fixed and stained with PI staining solution for 30 min in the dark and analyzed by FCM. Data were analyzed with NovoExpress 1.1.2 software.
For the apoptosis assay, cells were harvested and incubated with Annexin V-FITC for 30 min in the dark at 4 °C and then incubated with PI for 10 min, then detected by FCM.
2.7 Mitochondrial membrane potential (Δψm) assay
Mitochondrial membrane potential was measured by FCM when the cells were dyed with Rh123. The procedure was as below: Pfeiffer and Su-DHL-6 cells were treated with indicated doses ZLD1122 for 4 days. Cells were harvested and washed with cold PBS (0.1 M, pH 7.4) once and then incubated with 5 μg mL−1 Rh123 for 30 min in the dark. Then cells were washed once more with cold PBS. The fluorescence emitted from Rh123 was detected by FCM.16
2.8 Western blotting analysis
Cells were incubated separately with DMSO (vehicle control) or the indicated concentration of ZLD1122 for 4 days, or incubated with 4 μM ZLD1122 for the indicated times. Harvested cells were lysed in RIPA buffer (Beyotime, Beijing, China) on ice for 30 min. Then the cell lysates were centrifuged at 13
000g at 4 °C for 20 min, and the supernatant was harvested. The protein lysates were equalized by the BCA method before loading. After denaturing in loading buffer, about 20–60 mg of total protein from each sample was separated according to the molecular weight on 12.5% sodium dodecyl sulfate-polyacrylamide gel (SDS-PAGE) and transferred onto polyvinylidene fluoride (PVDF) membranes (Merck Millipore, Massachusetts, USA). After blocking with 5% fat-free milk in TBS/T for 2 h at room temperature, the membranes were incubated in primary antibody overnight at 4 °C. Then, the membranes were incubated with corresponding horseradish peroxidase-conjugated secondary antibodies.17 The immunoreactive protein bands were detected using an enhanced chemiluminescence kit (Millipore, USA). A monoclonal GAPDH antibody was used as a control.
2.9 Statistical analyses
Statistical analyses were carried out in GraphPad Prism 5.01 software. All data were statistically compared by one-way analysis of variance (ANOVA) followed by the Student’s t-test. A p value < 0.05 was considered statistically significant.
3. Results
3.1 Biochemical characterization of ZLD1122 as a potent inhibitor of EZH2 and EZH1
In biochemical assays using reconstituted PRC2, ZLD1122 exhibited potent and concentration-dependent inhibition of EZH2 (half-maximal inhibitory concentration [IC50] = 9.80 nM), EZH2 Y641N mutant (IC50 = 329.3 ± 35.57 nM), EZH2 Y641F mutant (IC50 = 182.3 ± 6.36 nM), EZH2 A677G mutant (IC50 = 15.77 ± 2.18 nM) and EZH1 (IC50 = 136.5 ± 27.72 nM; Fig. 2A–E). At the same time, the inhibition of EZH2 by the reported EZH2 inhibitors GSK126 and EPZ6438 was tested and the IC50 values were respectively 1.2 nM and 0.27 nM. We also assessed the inhibitory effect of ZLD1122 against a panel of HMTs other than EZH2. The results showed that EZH2 and EZH1 exhibited >10
000-fold selectivity compared to four other HMTs tested. These data suggested that ZLD1122 is a selective and potent inhibitor of EZH1 and EZH2.
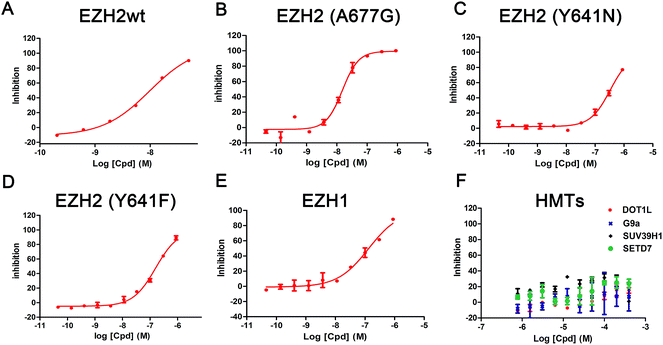 |
| Fig. 2 The enzyme preference of ZLD1122. (A–E) Potency of ZLD1122 against EZH1, wild-type and mutant EZH2. IC50 values were calculated from ten point dose response curves. Points: mean value; bars: SD. (F) Selectivity of ZLD1122 against a panel of HKMTs, including SETD7, SUV39H1, G9a and DOT1L. None of the tested enzymes were inhibited even at concentrations up to 100 mM. All enzyme assays were carried out under balance conditions as a ten point dose response. Points: mean value; bars: SD. | |
3.2 ZLD1122 inhibited the proliferation of cancer cells
In this study, we chose a panel of cancer cell lines of different histotypes to investigate the antiproliferative effect of ZLD1122, and the IC50 values were calculated. The results demonstrated that ZLD1122 differentially inhibited all of the tested cell lines after 4 days of incubation, with an optimal inhibitory effect on lymphoma cell lines at IC50 between 1.16 and 4.16 μM. Moreover, we examined the inhibitory effects of ZLD1122 on one noncancer cell line Vero, while the IC50 values were more than 20 μM (Table 2). Thus, we decided to study the effect of ZLD1122 on DLBCL cells.
Table 2 The effects of ZLD1122 on cell line viability. Each cell line was treated with ZLD1122 for 4 days and an MTT assay was used to determine the IC50 values. Data are expressed as mean ± SD for 3 independent experiments
Cell lines |
Cell type |
IC50 (μM) |
MDA-MB-435S |
Breast cancer |
2.70 ± 0.14 |
B16-F10 |
Melanoma |
11.56 ± 0.78 |
MDA-MB-231 |
Breast cancer |
5.40 ± 0.57 |
SKBR-3 |
Breast cancer |
7.93 ± 0.89 |
SMMC-7721 |
Hepatocellular carcinoma |
4.13 ± 0.52 |
HepG2 |
Hepatocellular carcinoma |
3.28 ± 0.60 |
HT-29 |
Colon carcinoma |
12.45 ± 2.20 |
HCT-116 |
Colon carcinoma |
15.94 ± 1.33 |
Pfeiffer |
Lymphoma |
1.43 ± 0.38 |
Su-DHL-6 |
Lymphoma |
2.01 ± 1.11 |
HBL-1 |
Lymphoma |
5.38 ± 1.87 |
OCI-LY-10 |
Lymphoma |
1.91 ± 0.70 |
Jurkat |
Acutelymphoblasticleukemia |
1.95 ± 0.63 |
Vero |
African monkey kidney cell line |
>20 |
To do this, we chose several DLBCL cell lines and conducted viability and proliferation assays with different concentrations of ZLD1122 for the indicated times. As shown in Fig. 3C and D, treatment of Pfeiffer cells with ZLD1122 for 2, 4, and 6 days decreased the IC50 from 2.76 to 1.16 μM after 2 to 4 days of treatment, while there was no significant shift in IC50 between 4 days and 6 days. Similar results were obtained for Su-DHL-6. Net decreases in Su-DHL-6 and Pfeiffer cell number were evident after 4 days of treatment (Fig. 3E and F). All these data suggested that ZLD1122 inhibited DLBCL cell proliferation in a dose-dependent manner and that the optimal incubation time is 4 days. Moreover, we found significant changes in cell morphology at increasing concentrations of ZLD1122 (Fig. 3G), which were further investigated.
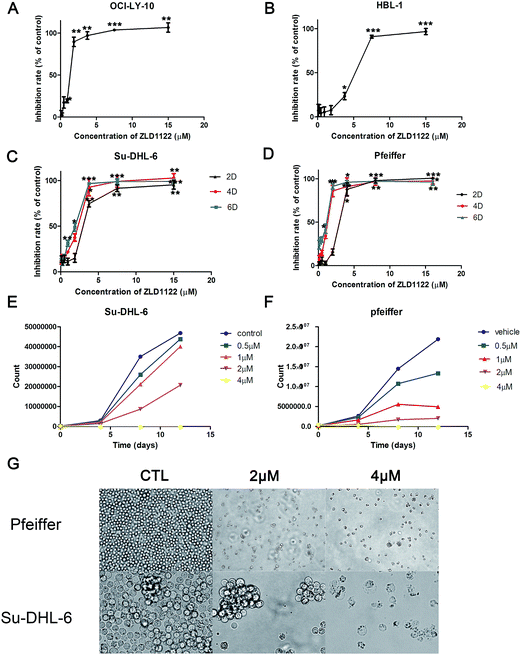 |
| Fig. 3 ZLD1122 inhibited the proliferation of several DLBCL cell lines. (A and B) Inhibition rate of ZLD1122 on EZH2 wild type DLBCL cells. OCI-LY-10 (A) and HBL-1 (B) were treated with ZLD1122 for 4 days. Each point represents the mean ± SD for 3 independent experiments (*p < 0.05, **p < 0.01, ***p < 0.001 vs. vehicle control). (C and D) Inhibition rate of ZLD1122 on EZH2 mutant DLBCL cells. Su-DHL-6 (C) and Pfeiffer (D) cell lines were treated with increasing doses of ZLD1122 for 2, 4 and 6 days. Each point represents the mean ± SD for 3 independent experiments (*p < 0.05, **p < 0.01, ***p < 0.001 vs. vehicle control). (E and F) Inhibition of proliferation of EZH2-mutant DLBCL cells by ZLD1122. Su-DHL-6 (E) and Pfeiffer (F) were replated at the original seeding densities on days 4 and 8. Each point represents the mean for each concentration (n = 3). (G) Effect of cell viability and morphological alterations of Su-DHL-6 and Pfeiffer treated with ZLD1122. Su-DHL-6 and Pfeiffer were incubated with ZLD1122 for 4 days and visualized using an inverted microscope. CTL represents the control group. | |
3.3 Intracellular inhibition of H3K27 methylation
Previous biochemical assays showed that ZLD1122 could target both wild type and mutant EZH2; however, whether targeting these enzymes will modulate histone methylation or not is not completely understood. For this purpose, western blotting assay was performed to detect the changes in the protein levels in ZLD1122-treated Pfeiffer and Su-DHL-6 cells. As shown in Fig. 4A, ZLD1122 most potently inhibited the expression of H3K27me3 in a dose-dependent manner, followed by H3K27me2 and H3K27me1, while it did not affect the expression of total histone H3. These results indicated that reduction of H3K27 methylation was due to direct inhibition of EZH2 methyltransferase activity and not the degradation of histone H3.
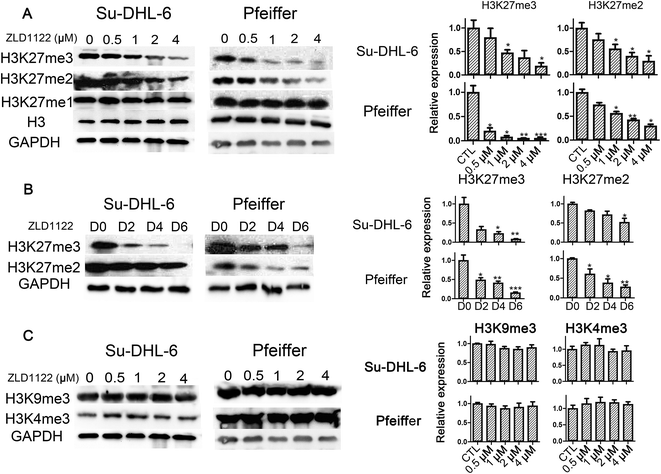 |
| Fig. 4 ZLD1122 led to a decrease in the tri- and di-methylation of H3K27. (A) Dose-dependent inhibition of cellular H3K27me3 and H3K27me2 levels in Su-DHL-6 and Pfeiffer cells, and H3K27me1 and total H3 levels, determined by western blotting. Pfeiffer and Su-DHL-6 were incubated with ZLD1122 for 4 days. (B) Time-dependent inhibition of cellular H3K27me3 and H3K27me2 levels in Su-DHL-6 and Pfeiffer cells, determined by western blotting. Pfeiffer and Su-DHL-6 were incubated with 4 μM ZLD1122 for 2, 4 and 6 days. (C) Protein levels of H3K9me3 and H3K4me3, determined by western blotting. Protein expressions were qualified by densitometry analysis using Image J (shown in the right panel). Data are expressed as mean ± SD for 3 independent experiments. Columns: mean; bars: SD (*p < 0.05, **p < 0.01, ***p < 0.001), D represents day. | |
A time-course study was performed on Pfeiffer and Su-DHL-6 cells to determine the relationship between response time of inhibition of EZH2 methylate function and cell apoptosis. We found that the expression of H3K27me3 and H3K27me2 proteins was inhibited in a time-dependent manner (Fig. 4B). In this study, two other histone methyl marks, H3K9me3 and H3K4me3, were tested to verify the specificity of ZLD1122 (Fig. 4C), and the results were consistent with the biochemical assay data. These data indicated that ZLD1122 might exert its effect on DLBCL cell growth through inhibition of H3K27 methylation.
3.4 ZLD1122 induced G0/G1 phase arrest of DLBCL cells
To further understand the role of ZLD1122 in the inhibition of DLBCL cell proliferation, we performed cell cycle analysis by FCM. As shown in Fig. 5A, after incubation with ZLD1122 for 4 days, the percentage of Su-DHL-6 cells in G0/G1 stage increased from 46.43% in the control group to 47.72%, 49.50%, 54.70%, and 63.33% in groups treated with increasing concentrations of ZLD1122. At the same time, a reduction in the percentage of G2/M phase cells was observed. Similar results were observed for Pfeiffer cells. We therefore concluded that ZLD1122 might inhibit DLBCL proliferation by inducing G0/G1 phase arrest.
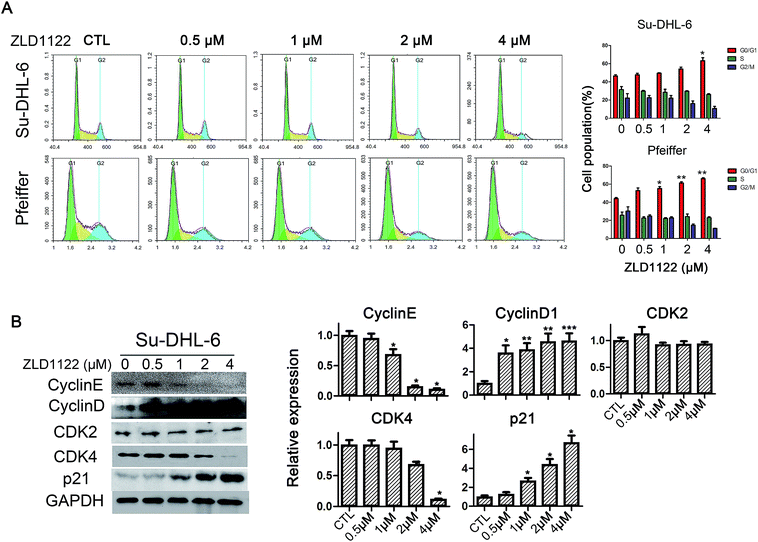 |
| Fig. 5 ZLD1122 induced G0/G1 arrest in DLBCL cells. (A) Cell-cycle distribution analyzed by FCM of PI staining. Su-DHL-6 and Pfeiffer cells were treated with escalating doses of ZLD1122 (0, 0.5, 1, 2 and 4 μM) for 4 days. (B) Intracellular protein levels of cyclinE, cyclinD1, CDK2, CDK4 and p21 determined via western blotting. Protein expression was qualified by densitometry analysis using Image J (shown in the right panel). Columns: mean; bars: SD (*p < 0.05, **p < 0.01, ***p < 0.001), CTL represents the control groups. | |
Cell cycle progression is also regulated by various cyclins and related cyclin-dependent kinases (CDKs). Therefore, we evaluated their expression in ZLD1122-treated cells by western blotting. As shown in Fig. 5B, the levels of cyclinE and CDK4 proteins decreased after treatment with ZLD1122 for 4 days, the expression of cyclinD1 increased, and no significant change was observed in the levels of CDK2 protein. Next, we investigated the protein level of p21, which is activated by p53 in many G0/G1 arrests, and found a increase in its level. Therefore, we indicated that the activation of p21 might inhibit the combination of cyclinD1 with CDK4, and consequently inhibit the phosphorylation of downstream genes such as pRb, which releases the transcription factor E2F to promote the transcription of other cyclins (like cyclinE) and CDKs and to promote the transition of the cell cycle to S phase. Thus, ZLD1122 induced G0/G1 arrest via the activation of the p53-dependent pathway.
3.5 ZLD1122 led to caspase-mediated apoptosis in DLBCL cells
The changes in morphology (Fig. 3G) indicated the activation of an apoptosis mechanism in Su-DHL-6 and Pfeiffer cells after ZLD1122 treatment. We investigated this mechanism by FCM and by measuring intracellular proteins. Propidium iodide (PI) staining showed that the percentage of sub-G1 phase Pfeiffer cells increased significantly from 3.47% to 62.49% after treatment with ZLD1122 for 4 days. When treated with 4 μM ZLD1122 for 2 and 4 days, the population of sub-G1 phase Pfeiffer cells increased from 32.82% to 62.49%. Similar results were obtained for Su-DHL-6 cells (Fig. 6A), indicating that ZLD1122 caused apoptosis of Pfeiffer and Su-DHL-6 cells in a concentration and time-dependent manner. We then performed Annexin V/PI staining to further determine the pro-apoptotic effect of ZLD1122 on DLBCL cell lines. As shown in Fig. 6B, when Su-DHL-6 cells were exposed to ZLD1122 for 4 days, the proportion of early (Q3, only Annexin V-positive) and late apoptotic cells (Q4, both Annexin V- and PI-positive) remarkably increased from 2.07% to 3.55% and to 54.28% when the concentration increased from 1 to 2 and 4 μM, respectively. Almost no apoptotic cells were detected in the control group. Similar results were obtained for Pfeiffer cells. These results indicated that ZLD1122 induced apoptosis of Pfeiffer and Su-DHL-6 cells in a concentration-dependent manner.
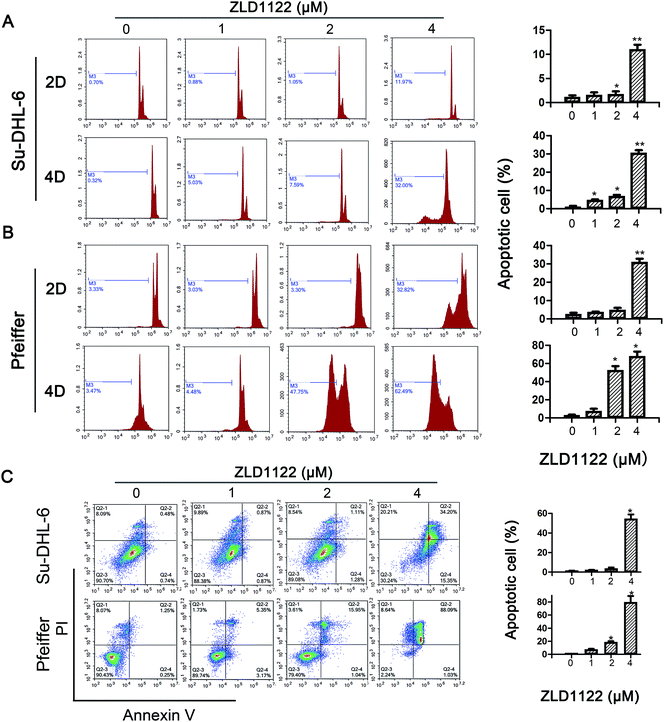 |
| Fig. 6 ZLD1122 induced apoptosis in DLBCL cells. (A and B) Dose- and time-dependent apoptosis of Su-DHL-6 and Pfeiffer cells. FCM analysis of PI-stained Su-DHL-6 (A) and Pfeiffer (B) cell lines. The two cell lines were treated with ZLD1122 with increasing concentration for 2 and 4 days. (C) Su-DHL-6 and Pfeiffer cells were treated with ZLD1122 for 4 days and apoptosis was detected by FCM after Annexin V/PI staining, the Annexin V positive population was considered to be apoptosis cells. | |
Western blotting was performed to detect the changes of caspase and Bcl-2 family proteins, which are related to apoptosis. Bcl-2 family members are the key regulators of apoptosis. As shown in Fig. 7A, the level of the pro-apoptotic protein Bax increased while that of the anti-apoptotic protein Bcl-2 decreased with an increase in ZLD1122 concentration. Moreover, an increase in the level of cleaved caspase-3, the main executor of apoptosis, was observed after a 4 day treatment with ZLD1122 in both cell lines.
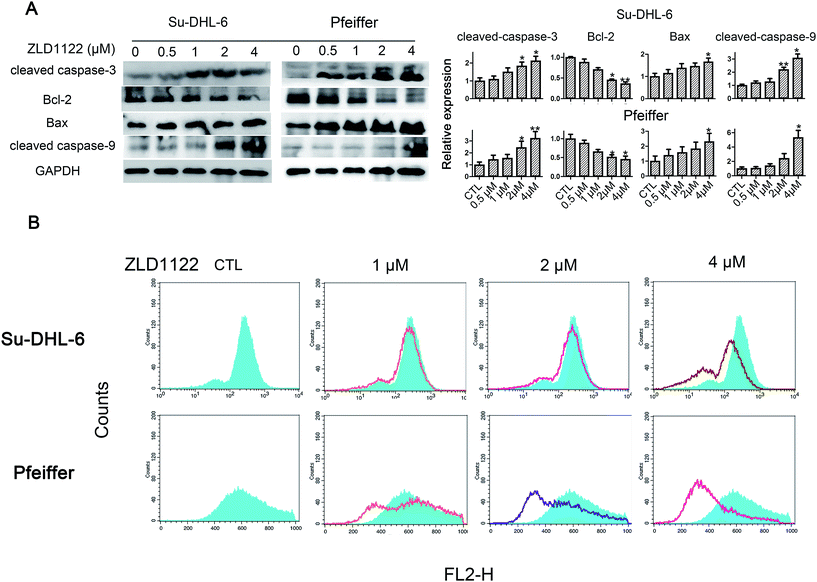 |
| Fig. 7 ZLD1122 induced apoptosis through an intrinsic pathway. (A) The levels of cleaved caspase-3, Bcl-2, Bax and cleaved caspase-9 determined via western blotting. Protein expression was qualified by densitometry analysis using Image J (shown in the right panel). Columns: mean; bars: SD (*p < 0.05, **p < 0.01, ***p < 0.001, vehicle control). (B) Changes of the mitochondrial membrane potential of Su-DHL-6 and Pfeiffer cells were detected by FCM after treatment with ZLD1122 for 4 days, CTL represents the control groups. | |
To further elucidate the mechanism of this intrinsic apoptosis, we investigated the expression of cleaved caspase-9, an important member of the mitochondria-mediated intrinsic apoptosis pathway. The expression of cleaved caspase-9 increased in a dose-dependent manner after ZLD1122 treatment for 4 days.
Next, we investigated the effect of ZLD1122 on the mitochondrial membrane potential (Δψm) of Pfeiffer and Su-DHL-6 cells by FCM using rhodamine 123 (Rh123), to further verify that ZLD1122 treatment induced cell apoptosis through the mitochondria-mediated intrinsic apoptosis pathway. Δψm loss was induced in a ZLD1122 concentration-dependent manner in both cell lines (Fig. 7B). From the data above, we can preliminarily conclude that ZLD1122 induced apoptosis in DLBCL cells most probably through the mitochondria-mediated intrinsic apoptosis pathway.
4. Discussion
Thus far, a major hurdle to the development of new molecular cancer treatments has been the inability to distinguish between passenger and driver mutations. Selective inhibitors of genetically altered enzymes and antagonists of altered receptors have been proven to be valuable in making such distinctions.18 NHL, in particular DLBCL, is still an aggressive cancer worldwide, since it metastasizes throughout the body and suppresses immunity. NHL patients treated via the CHOP strategy experience several side effects, as well as a 90% relapse rate.19,20 Although ibrutinib has been widely proven to be efficient against some subtypes of NHL, DLBCL was reported to be resistant to it.21 Epigenetic enzymes, HMTs, play an important role in the regulation of gene transcription. EZH2, the key catalytic subunit of PRC2 which works by catalyzing methylation of H3K27 in core histones, is attracting increasing attention in targeted therapies of solid tumors and myeloma. Previous studies have shown that mountains of cancer cells were highly dependent on PRC2 for proliferation.3 And several molecular mechanisms leading to a hypertrimethylated state of H3K27 have been detected among human cancers such as breast cancer,22 prostate cancer,23 hepatocellular carcinoma,24 and lymphoma.25 In DLBCL, overexpression and mutation of EZH2 are the main causes of hypertrimethylation of H3K27, leading to new directions in DLBCL therapy, i.e., inhibiting the tumor’s progression by blocking its PRC2 dependency. Thus, inhibitors of EZH2 could be developed as a new generation of antilymphoma agents.
Our group synthesized a series of compounds, of which ZLD1122 exhibited selective inhibition of EZH1 and EZH2 enzymatic activity. In addition, ZLD1122 inhibited the growth of a broad spectrum of human cancer cell lines and displayed optimal antiproliferative activities against NHL cells (with IC50 values from 1.16 to 4.16 μM after 4 days of treatment). In DLBCL viability and progression assays, ZLD1122 was found to inhibit DLBCL cell growth and viability in both a dose- and time-dependent manner. These primary data indicated that ZLD1122 inhibited NHL cell growth by inhibiting the trimethylation of H3K27. Therefore, in this study, we investigated the effect of ZLD1122 on DLBCL cells and its underlying mechanism. Western blotting showed that ZLD1122 significantly inhibited the tri- and di-methylation of H3K27, while the protein levels of H3, H3K9me3, and H3K4me3 were not affected. This indicates that ZLD1122 exhibited its effect on DLBCL cells through the inhibition of PRC2 catalytic function on H3K27, verifying the inference that ZLD1122 is a bona fide, selective, functional inhibitor of PRC2.
Since previous studies demonstrated that blocking of H3K27 hypertrimethylation on promoters might reactivate the expression of PRC2 target genes associated with proliferation, we performed cell cycle distribution and apoptosis assays to further understand the mechanism underlying ZLD1122’s effect on DLBCL cells. Results of the cell cycle analysis showed that ZLD1122 blocked Su-DHL-6 and Pfeiffer cell cycle progression and caused a G0/G1 phase arrest. The reactivation of upstream genes might lead to the transcriptional activation of p21WAF1/ClPl/SDI1 (p21).26 p21 inhibits G1 cyclin–CDK complexes, such as cyclinD–CDK4/CDK6, which would, in turn, inhibit the phosphorylation of pRb,27,28 thereby preventing the activation of E2F and the corresponding G1/S transition genes.29 In our study, we detected an upregulation of p21 and cyclinD1, indicating that ZLD1122 most probably induced G0/G1 phase arrest of DLBCL cells through reactivation of downstream genes of PRC2.
In our apoptosis study, we found that ZLD1122 induced DLBCL cell apoptosis. After treatment of Su-DHL-6 and Pfeiffer cells with ZLD1122, the level of the pro-apoptotic protein Bax was upregulated while the level of the anti-apoptotic protein Bcl-2 was downregulated. In addition, an increase in the expression of cleaved caspase-3,9 and a decrease in Δψm were observed. So we demonstrated that treatment with ZLD1122 could further activate the mitochondria dependent intrinsic apoptotic pathway in DLBCL cells. Zhou, et al. attest that targeting EZH2 regulates tumor apoptosis through modulating mitochondria dependent cell-death pathways,30 so although the underlying mechanism is not entirely clear, our data showed that ZLD1122 inhibition of EZH2 triggers a loss of Δψm and changes in proteins of mitochondrial related cell death pathways.
In conclusion, our synthesized compound ZLD1122, an EZH2 and EZH1 inhibitor, had remarkable efficacy in inhibiting human NHL progression in vitro. Mechanistically, ZLD1122 blocked the trimethylation of H3K27 and further led to cell cycle arrest and apoptosis by reactivation of PRC2 target genes. Together, the results indicated that ZLD1122 is a promising agent for application in NHL therapy. In vivo studies and preclinical evaluation are therefore warranted.
Acknowledgements
This work was supported by Zhejiang Apeloa Medical Technology Co., Ltd.
References
- J. Z. Tan, Y. Yan, X. X. Wang, Y. Jiang and H. E. Xu, Acta Pharmacol. Sin., 2014, 35, 161–174 CrossRef CAS PubMed.
- K. Helin and D. Dhanak, Nature, 2013, 502, 480–488 CrossRef CAS PubMed.
- L. Y. Li, Biomedicine, 2014, 4, 1 CrossRef CAS PubMed.
- A. Kuzmichev, K. Nishioka, H. Erdjument-Bromage, P. Tempst and D. Reinberg, Genes Dev., 2002, 16, 2893–2905 CrossRef CAS PubMed.
- R. Cao, L. Wang, H. Wang, L. Xia, H. Erdjument-Bromage, P. Tempst, R. S. Jones and Y. Zhang, Science, 2002, 298, 1039–1043 CrossRef CAS PubMed.
- W. K. Bae and L. Hennighausen, Mol. Cell. Endocrinol., 2014, 382, 593–597 CrossRef CAS PubMed.
- S. Garapaty-Rao, C. Nasveschuk, A. Gagnon, E. Y. Chan, P. Sandy, J. Busby, S. Balasubramanian, R. Campbell, F. Zhao and L. Bergeron, Chem. Biol., 2013, 20, 1329–1339 CrossRef CAS PubMed.
- M. Mounier, N. Bossard, L. Remontet, A. Belot, P. Minicozzi, R. De Angelis, R. Capocaccia, J. Iwaz, A. Monnereau and X. Troussard, Lancet Haematology, 2015, 2, e481–e491 CrossRef PubMed.
- R. Chakraborty, P. Kapoor, S. M. Ansell and M. A. Gertz, Expert Rev. Hematol., 2015, 8, 569–579 CrossRef CAS PubMed.
- S. Park, J. Hong, I. Hwang, J. Y. Ahn, E. Y. Cho, J. Park, E. K. Cho, D. B. Shin and J. H. Lee, Journal of Geriatric Oncology, 2015, 6, 470–478 CrossRef PubMed.
- F. Iioka, K. Izumi, Y. Kamoda, T. Akasaka and H. Ohno, Int. J. Clin. Oncol., 2015, 1–8 Search PubMed.
- A. Chase and N. C. Cross, Clin. Cancer Res., 2011, 17, 2613–2618 CrossRef CAS PubMed.
- R. D. Morin, M. Mendez-Lago, A. J. Mungall, R. Goya, K. L. Mungall, R. D. Corbett, N. A. Johnson, T. M. Severson, R. Chiu, M. Field, S. Jackman, M. Krzywinski, D. W. Scott, D. L. Trinh, J. Tamura-Wells, S. Li, M. R. Firme, S. Rogic, M. Griffith, S. Chan, O. Yakovenko, I. M. Meyer, E. Y. Zhao, D. Smailus, M. Moksa, S. Chittaranjan, L. Rimsza, A. Brooks-Wilson, J. J. Spinelli, S. Ben-Neriah, B. Meissner, B. Woolcock, M. Boyle, H. McDonald, A. Tam, Y. Zhao, A. Delaney, T. Zeng, K. Tse, Y. Butterfield, I. Birol, R. Holt, J. Schein, D. E. Horsman, R. Moore, S. J. Jones, J. M. Connors, M. Hirst, R. D. Gascoyne and M. A. Marra, Nature, 2011, 476, 298–303 CrossRef CAS PubMed.
- L. Zhang, X. Song, N. Wang, L. Zhao, Q. Feng, X. You, C. Peng, T. Gao, M. Xiong and B. He, RSC Adv., 2015, 5, 25967–25978 RSC.
- S. K. Knutson, S. Kawano, Y. Minoshima, N. M. Warholic, K. C. Huang, Y. Xiao, T. Kadowaki, M. Uesugi, G. Kuznetsov, N. Kumar, T. J. Wigle, C. R. Klaus, C. J. Allain, A. Raimondi, N. J. Waters, J. J. Smith, M. Porter-Scott, R. Chesworth, M. P. Moyer, R. A. Copeland, V. M. Richon, T. Uenaka, R. M. Pollock, K. W. Kuntz, A. Yokoi and H. Keilhack, Mol. Cancer Ther., 2014, 13, 842–854 CrossRef CAS PubMed.
- Q. Lei, L. Zhang, Y. Xia, T. Ye, F. Yang, Y. Zhu, X. Song, N. Wang, Y. Xu and X. Liu, RSC Adv., 2015, 5, 41341–41351 RSC.
- Y. Xia, Q. Lei, Y. Zhu, T. Ye, N. Wang, G. Li, X. Shi, Y. Liu, B. Shao, T. Yin, L. Zhao, W. Wu, X. Song, Y. Xiong, Y. Wei and L. Yu, Cancer Lett., 2014, 355, 297–309 CrossRef CAS PubMed.
- S. K. Knutson, T. J. Wigle, N. M. Warholic, C. J. Sneeringer, C. J. Allain, C. R. Klaus, J. D. Sacks, A. Raimondi, C. R. Majer and J. Song, Nat. Chem. Biol., 2012, 8, 890–896 CAS.
- G. G. Vega, A. Aviles-Salas, J. R. Chalapud, M. Martinez-Paniagua, R. Pelayo, H. Mayani, R. Hernandez-Pando, O. Martinez-Maza, S. Huerta-Yepez, B. Bonavida and M. I. Vega, BMC Cancer, 2015, 15, 722 CrossRef PubMed.
- G. G. Vega, L. A. Franco-Cea, S. Huerta-Yepez, H. Mayani, S. L. Morrison, B. Bonavida and M. I. Vega, Int. J. Oncol., 2015, 47, 1735–1748 Search PubMed.
- J. H. Kim, W. S. Kim, K. Ryu, S. J. Kim and C. Park, Leuk. Lymphoma, 2015, 1–10, DOI:10.3109/10428194.2015.1113276.
- K. H. Yoo and L. Hennighausen, Int. J. Biol. Sci., 2012, 8, 59–65 CrossRef CAS PubMed.
- F. Crea, E. M. Hurt, L. A. Mathews, S. M. Cabarcas, L. Sun, V. E. Marquez, R. Danesi and W. L. Farrar, Mol. Cancer, 2011, 10, 40 CrossRef CAS PubMed.
- S.-B. Gao, Q.-F. Zheng, B. Xu, C.-B. Pan, K.-L. Li, Y. Zhao, Q.-L. Zheng, X. Lin, L.-X. Xue and G.-H. Jin, Mol. Cancer Res., 2014, 12, 1388–1397 CrossRef CAS PubMed.
- H. J. Lee, D. H. Shin, K. B. Kim, N. Shin, W. Y. Park, J. H. Lee, K. U. Choi, J. Y. Kim, C. H. Lee and M. Y. Sol, Leuk. Lymphoma, 2014, 55, 2056–2063 CrossRef CAS PubMed.
- W. S. El-Deiry, J. W. Harper, P. M. O’Connor, V. E. Velculescu, C. E. Canman, J. Jackman, J. A. Pietenpol, M. Burrell, D. E. Hill and Y. Wang, Cancer Res., 1994, 54, 1169–1174 CAS.
- J. W. Harper, G. R. Adami, N. Wei, K. Keyomarsi and S. J. Elledge, Cell, 1993, 75, 805–816 CrossRef CAS PubMed.
- V. Dulić, W. K. Kaufmann, S. J. Wilson, T. D. Tisty, E. Lees, J. W. Harper, S. J. Elledge and S. I. Reed, Cell, 1994, 76, 1013–1023 CrossRef.
- S. P. Linke, K. C. Clarkin, A. Di Leonardo, A. Tsou and G. M. Wahl, Genes Dev., 1996, 10, 934–947 CrossRef CAS PubMed.
- X. Zhou, Y. Ren, L. Kong, G. Cai, S. Sun, W. Song, Y. Wang, R. Jin, L. Qi, M. Mei, X. Wang, C. Kang, M. Li and L. Zhang, Oncotarget, 2015, 6, 33720–33732 Search PubMed.
Footnote |
† These authors contributed equally to this work. |
|
This journal is © The Royal Society of Chemistry 2016 |
Click here to see how this site uses Cookies. View our privacy policy here.