DOI:
10.1039/C6RA00194G
(Paper)
RSC Adv., 2016,
6, 33092-33100
One-step green synthesis of gold and silver nanoparticles with ascorbic acid and their versatile surface post-functionalization†
Received
4th January 2016
, Accepted 22nd March 2016
First published on 29th March 2016
Abstract
In this study, we present a rapid and efficient synthesis of metallic gold and silver nanoparticles using ascorbic acid (vitamin C), which acts both as reducing and stabilizing agent. The size of the particles obtained, in the range of 8 to 80 nm for gold and 20 to 175 nm for silver, and their polydispersity (10–50%) are subtly affected, therefore tunable, by the pH of either the metal salt solution or the ascorbic acid solution. We also show that one of the main features of this synthetic approach is the simple, fast and versatile nanoparticle surface modification with a large variety of water soluble surfactants that can be neutral, positively or negatively charged.
Introduction
Studies on gold and silver nanoparticles (Au and Ag NPs) have been the focus of intense and increasing research activity because of their unique optical absorption properties displayed in the visible range. These systems have had tremendous impacts in various domains,1 such as photovoltaics,2–5 optics,6,7 catalysis,8–10 medical imaging and therapy.11–13 The surface plasmon resonance of these noble metallic NPs is caused by the collective oscillations of the conduction band electrons interacting with electromagnetic radiation. This phenomenon is principally responsible for their extinction properties in the visible range of the solar spectrum, and thus is directly responsible for the impact on various technologies noted above. Since the plasmon resonance depends strongly on the NPs size, shape and surrounding environment,14–17 significant effort has been devoted to establish reliable synthetic methods of Au and Ag NPs.18–24 The development of environmentally friendly and biocompatible syntheses25–32 is particularly attractive to enable the use of metallic NPs in a wider array of applications. The reduction of metal salts in aqueous media using a variety reducing agents such as sodium citrate,33–35 ascorbic acid36,37 or tannic acid38 represents an attractive option. But there also are numerous studies dedicated to the synthesis of gold and silver particles using natural and biocompatible compounds.27,39–47 In these procedures, the control of pH, is a crucial parameter to tune the size, size distribution and/or shape of the NPs.33,37,48
Moreover, the control of surface chemistry is a key parameter to enable the use of NPs in various applications and a wide range of methodologies have been explored for their subsequent surface functionalization. Grafting specific molecules onto the surface of NPs is necessary to promote their incorporation into the desired solvents49–51 or assembly in materials and devices, to observe plasmonic enhancement,52 and to develop new functional systems for sensing, imaging, drug delivery, diagnostic or therapy.53–56 To attach the desired molecules onto the NPs surface, the initial stabilizing agent needs to be exchanged by a surfactant which has a stronger affinity with the particle's surface than that of the initial stabilizing agent. Thiolated molecules are commonly used due to their strong binding affinity with metal.51,57–59 To overcome the limitation of the ligand exchange due to their differences in binding affinity, other functionalization methods have been investigated. For example a chemical reaction can be performed on the existing ligand to generate the desired molecular ligand architecture.49,50,60 In addition, one can develop direct syntheses routes involving the presence of the final stabilizing agent, a technique of relevance for biocompatibility purposes (e.g. less contamination).61–65 The former approach is however restricted to only a few chemical reactions, whereas the latter shows little control in the NPs morphology. A reliable synthesis of calibrated plasmonic NPs using a stabilizer that has only a moderate affinity for the NP surface would enable facile surface functionalization by a wide variety of existing ligands, and would therefore represent an attractive advance in methodology for this field of research.
In this study, we present a one-step, green synthesis performed at room temperature using the biocompatible, ascorbic acid (i.e. vitamin C), which serves as both reducing and stabilizing agent. The size of the NPs in this synthesis is tuned by changing the pH of either the metal salt solution or the ascorbic acid (AA) solution. We also demonstrate that the surface chemistry can be easily and quickly modified with a large variety of surfactants used widely in chemical research and industrial processes.
Experimental section
Chemicals
The following chemicals were used as received without further purification. Gold(III) chloride trihydrate (≥99.9%_HAuCl4), silver nitrate (>99%_AgNO3) L-ascorbic acid (BioUltra, ≥ 99.5%_AA), hexadecyltrimethylammonium bromide (BioUltra > 99%_CTAB), sodium dodecyl sulfate (>99%_SDS) and polyvinylpyrrolidone (Mw = 10
000_PVP-10) were purchased from Sigma Aldrich. Sodium hydroxide pellets (≥97.0%) were purchased from Fisher Scientific. Geropon T-77 (negatively charged surfactant), Rhodameen PN-430 (positively charged surfactant) and nonionic surfactants: Antarox L-64 (block copolymer), Igepal CO-630 (nonionic aromatic) and Lubrophos LB-400 (metal lubricant) were provided by Solvay.
Synthesis of gold NPs Au@AA
HAuCl4 pH effect. 1.5 L of a 0.5 mM aqueous fresh gold salt (HAuCl4) solution was separated into 30 vials of 50 mL solutions. Their pH was tuned by adding different amounts of a 0.10 M sodium hydroxide (NaOH) solution (from 0 to 1.6 mL) in order to reach different ratios R1 = [NaOH]/[HAuCl4] from 0 to 6.4. The gold salt mixtures were then allowed to rest for 48 h to stabilize the pH at its equilibrium value. Then, 0.50 mL of a 0.10 M AA solution was added to each pH-adjusted gold solution while vigorously stirring at room temperature. Vigorous stirring (obtention of strong vortex) was maintained for 30 s. During this short period, the solutions became red with a transition rate depending on the R1 ratio (when R1 increases, the reaction rate decreases). After the change of color, the reactions were completed by gentle stirring the solutions for 30 min. The as made suspensions were stored at room temperature and remained stable for about one month.
AA pH effect. 550 mL of 0.10 M AA solution was separated into 11 vials of 50 mL solutions. Their pH was tuned by adding different amounts of a 10 M NaOH solution (from 0 to 1 mL) in order to reach different ratios R2 = [NaOH]/[AA] from 0 to 2. to reach pH equilibrium, the AA mixtures were allowed to rest for 3 h. Then, 0.50 mL of the pH-adjusted AA solution was added to 50 mL of a 0.50 mM fresh gold salt (HAuCl4) solution while vigorously stirring at room temperature. Vigorous stirring was maintained for 30 s (vortex). During this short time, the solutions became red with a transition rate depending on the R2. After the change of color, the reactions were completed by gentle stirring the solutions for 30 min. The as made suspensions were stored at room temperature and remained stable for about one month.
Synthesis of silver NPs Ag@AA
750 mL of a 0.10 M AA solution was separated into 15 vials of 50 mL solutions. Their pH was tuned by adding different amounts of a 10 M NaOH solution (from 0 to 2 mL) in order to reach different ratios R1 = [NaOH]/[HAuCl4] from 0 to 4. to equilibrate the pH the AA mixtures were allowed to rest for 3 h. Then, 0.50 mL of a 50.0 mM fresh silver salt (AgNO3) solution was added to 50 mL of the pH-adjusted AA solutions under a strong stirring (vortex) at room temperature. Vigorous stirring was maintained for 30 s. During this short time, the solutions became grey or yellow with a transition rate depending on the R3 ratio. After the change of color, the reactions were completed by gentle stirring the solutions for 30 min. During this time, the color became more and more intense, indicating that the reaction was not complete. The mixtures were kept undisturbed for 12 h. The as made suspensions can be stored at room temperature, in the dark, and remained stable for about one month.
Surface modification of the NPs
The process used is the same regardless of the surfactant considered. An aqueous surfactant solution (arbitrary concentration) was added, under gentle stirring, to 50 mL of as made Au@AA NPs in order to reach a final concentration of 5.0 mM of surfactant in solution. The Au@surfactants NP were washed to remove the excess of AA. A centrifugation of the solutions at 4500 rpm for 30 min induces the precipitation of the NPs and allows the replacement of the supernatant by controlled solutions of surfactants. For the first two centrifugations, the supernatant was replaced by a 5.0 mM surfactant solution. For the third centrifugation, water was used to replace the supernatant.
Structural and optical characterizations
Transmission electron microscopy (TEM) images were acquired on a JEM-1400 microscope operating at 120 kV. The size and polydispersity of the particles have been obtained by statistical measurements on TEM images (≈200 particles per sample). For a better comparison between the different samples, we defined and computed the polydispersity p, as a percentage corresponding to the sample standard deviation (σ) normalized by the sample mean (absolute polydispersity are presented for information purposes). Optical extinction spectra were recorded in transmission mode using a Cary 5000 spectrophotometer. The pH of the solutions was measured with an Accumet AP115 pH meter (From Fisher Scientific). Zeta potential measurements were performed on a Delsa Nano C (Beckman Coulter) instrument using a quartz cell.
Results and discussions
Gold NPS: effect of the HAuCl4 solution pH
The Au@AA NPs have been synthesized using different ratios, R1 = [NaOH]/[HAuCl4], varying from 0 to 6.4, that lead to a variation in the pH of the gold salt solution. The pH curve (Fig. 1) exhibits a continuous increase as more NaOH is added with a pH jump at R1 = 5. For R1 < 1, the added NaOH primarily consumes the free H+ ions from HAuCl4. Then, for 1 < R1 < 5, the gold salt complex [AuCl4]− evolves into [AuCl4−x(OH)x]− through the reaction shown in eqn (1). This exchange between OH− and Cl− leads to a slow rise of the pH.48 The pH increases sharply when all the Cl− are exchanged with OH− around R1 = 5. For R1 > 5, the increase of pH is only due to excess of NaOH. |
[AuCl4]− + xOH− ⇄ [AuCl4−x(OH)x]− + xCl−
| (1) |
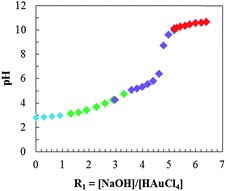 |
| Fig. 1 pH curves for the gold salt solutions with different R1 = [NaOH]/[HAuCl4] ratios. | |
The extinction spectra of the NPs synthesized at each point on the pH curve are represented in Fig. 2. These spectra exhibit four regimes: consumption of the free H+ (blue), evolution of [AuCl4]− into [AuCl4−x(OH)x]− (green), termination of the [Au(OH)4]− formation (purple) and addition of free NaOH (red). To better visualize the evolution of the plasmonic properties with an increase of R1, the plasmonic peak positions (λmax, dots) are plotted in Fig. 3a, and the half width of the peak (HWHM, dots) in Fig. 3b, respectively. As it is well known, for spherical NPs, the extinction spectrum is sensitive to the particle diameter and polydispersity; as the diameter and polydispersity increase, the maximum of the plasmonic absorption is red-shifted and the half-width at half-max (HWHM) increases, respectively. In order to check these correlations, the size and the polydispersity of the NPs have been measured by TEM (representative samples displayed on Fig. 4) and compared to the optical properties. In Fig. 3a, NPs diameters (triangles) are compared to the position of the plasmon peak (dots). The polydispersity (triangles) and HWHM (dots) are compared in Fig. 3b. The half width is used here since the plasmonic peak is not symmetric due to the absorbance plateau resulting from the interband transition of metallic gold.66
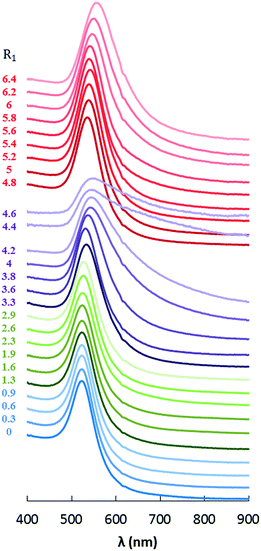 |
| Fig. 2 Extinction spectra of the Au NPs obtained with different R1 = [NaOH]/[HAuCl4] ratios (normalized at 400 nm). | |
 |
| Fig. 3 Comparison of (a) the diameters measured by TEM and λmax; (b) the polydispersity measured by TEM and HWHM of NPs obtained with different R1 = [NaOH]/[HAuCl4]. | |
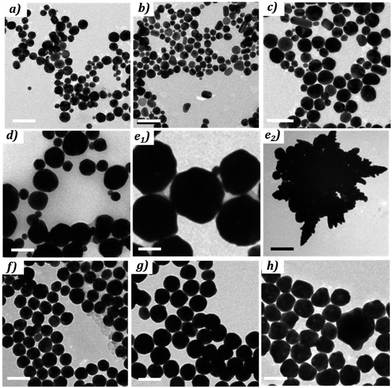 |
| Fig. 4 TEM pictures of NPs obtained with different ratio R1 = [NaOH]/[HAuCl4] (reported in Table 1) scale bare = 100 nm, (e2) scale bare = 0.5 μm. | |
As the free H+ are consumed (0 < R1 < 1, blue), no significant change in the extinction spectra is observed, indicating that the synthetized NPs are similar: diameter = 28 nm (σ = 30%) (Fig. 4a and b). Through the exchange of Cl− by OH− (green), 1 < R1 < 4, the maximum of the plasmonic resonance is red-shifted and the peak is broadened as R increases. This is confirmed by the TEM images, which reveal a clear increase in size and polydispersity of the NPs from 28 nm (σ = 30%) to 70 nm (σ = 50%) (Fig. 4c and d). The third regime, occurs at the pH jump for 4 < R1 < 5 (purple). This regime appears to be unstable during which the synthesized NPs coexist as a mixture of large spheres (200 nm, σ = 20%) and small populations of very large (∼2 μm) dendritic particles (Fig. 4e). In the last regime (red), R1 > 5, the plasmon response is red-shifted and the resonance is broadened when the amount of free OH− increases. Indeed, the NPs sizes increase from 55 ± 12% up to 82 ± 24% (Fig. 4f–h). In this regime, the polydispersity of the NPs decreases by a factor of 2 when compared to that of the NPs obtained at 0 < R1 < 4.
The different types of NPs (sizes, shapes, dispersity,…) obtained in these four regimes can be explained by the relationship between pH and the redox reactivity of the complexes [AuCl4−x(OH)x]−. Here, we assume that the pH only affects the redox potential of [AuCl4−x(OH)x]−. As the reaction between the AA and the pH-adjusted gold salt is very fast (∼30 s), we consider that the redox potential of AA does not have enough time to be modified by the pH (as the AA pH stabilizes on the order of 10 min). As previously stated, for R1 < 1 (blue domains), the added NaOH primarily consumes free H+ from HAuCl4, so the reactivity of the gold salt does not change. This can account for the similarity of the NPs made at R1 < 1. It has been shown that during the evolution of the complex [AuCl4]− into [AuCl4−x(OH)x]−, the redox potential E0 of the Au(III) complexes abruptly decreases.33,48 Decreasing E0 leads to a decrease in the difference of redox potentials between [AuCl4−x(OH)x]− and the reducing agent, which slows the rate of the reaction. By slowing down the reduction of gold, fewer nuclei are created which explains why the NPs formed at 1 < R1 < 4 (green domains) and R1 > 5 (red domains) are larger when the pH increases. Decreasing the reduction rate also creates competition between nucleation and growth of the existing NPs. Having simultaneous nucleation and growth on older nuclei lead to the formation of NPs of different sizes.
The particles obtained for 4 < R1 < 5 present the highest polydispersity and in some case the presence of large dendritic objects (Fig. 4d and e) which explain the broad plasmonic pics observed in this regime. Indeed this regime corresponds to the pH jump meaning that there multiple complexes [AuCl4−x(OH)x]− in presence. Knowing that each complex has a different reactivity, it is expected to obtain various and/or non-ordinary objects.
Then, when [Au(OH)4]− is the only compound present, R1 > 5, the redox potential continues to decrease as the pH increases. In this case, the kinetics of nucleation and growth appear to give NPs that are more monodisperse. As we keep increasing the pH, the nucleation rate decreases and leads to an increase in the polydispersity.
Gold NPs: effect of ascorbic acid solutions pH
The Au@AA NPs have been synthesized using different ratios R2 = [NaOH]/[AA], ratios varying from 0 to 2. Each point on the pH curve (Fig. 5) corresponds to a distinct Au@AA synthesis and the pH evolution is in agreement with the values of the two pKa of AA (pKa1 = 4.1, at the very beginning of the curve, and pKa2 = 11.6 occurring at R2 ≈ 1, also consistent with the stoichiometry).
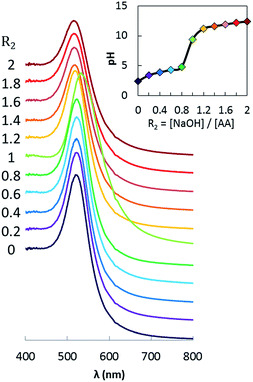 |
| Fig. 5 pH variation of the AA solution as a function of R2 = [NaOH]/[AA] and extinction spectra of the Au NPs obtained with these ratios (normalized at 400 nm). | |
The extinction spectra of the NPs synthesized at different R2 are presented in Fig. 5 and the corresponding TEM measurements in Fig. 7. The morphology of the NPs and their optical properties are compared in Fig. 6 for different values of R2. In Fig. 6a, the plasmonic peak positions (λmax, dots) and the diameter of the NPs (triangles) (from Fig. 5 & 7) as a function of R2 are superimposed on the same graph. The HWHM (dots) and the polydispersity (triangles) are plotted in Fig. 6b. These data reveal two different regimes: before the equivalent point (R2 = 1), the NPs morphology does not depend on the stoichiometry. In this range, the NPs diameter is 30 nm (σ = 30%). Beyond the equivalent point, a different behavior arises, and the diameter of the NPs decreases from 11 nm (σ = 28%) to 7.8 (σ = 23%).
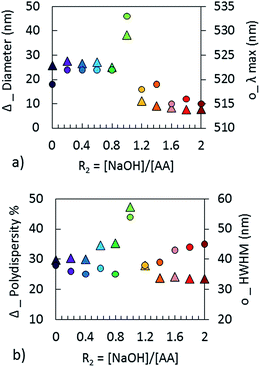 |
| Fig. 6 Comparison of (a) the diameters measured by TEM and λmax; (b) the polydispersity measured by TEM and HWHM of NPs obtained with different R2 = [NaOH]/[AA]. | |
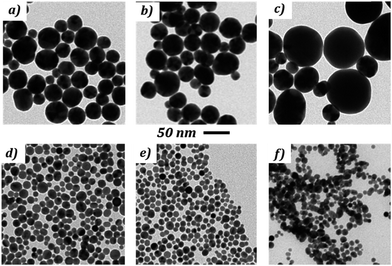 |
| Fig. 7 TEM pictures of gold NPs obtained with different R2 = [NaOH]/[AA] ratio reported in Table 2. | |
These observations can be explained as follows. Goia et al.48 reported that the redox potential of AA is a function of the pH: when the pH increases, the redox potential decreases. For pH < 11.6, the slope of the curve E0 = f(pH) is significantly less important than at a higher pH. Below this pH value (<11.6), due to the very fast rate of reaction between the gold salt and the AA, the complex [AuCl4]− does not have enough time to be converted into [AuCl4−x(OH)x]−. Moreover, in this range, the pH has a small effect on the redox potential, therefore, its reactivity remains the same regardless of the pH of the solution. Above pH = 11.6, there is an increase in the difference of redox potentials between [AuCl4]− and the AA, making the reaction faster. By increasing the reactivity between the gold salt and the reducing agent, more nuclei are created which explains why the NPs are smaller when the pH increases.
Table 1 Diameters, polydispersity and plasmonic resonance wavelength associated to the particles presented Fig. 4
|
a |
b |
c |
d |
e |
f |
g |
h |
R1 |
0 |
1.6 |
2.6 |
4 |
4.6 |
5 |
5.4 |
6.4 |
Diameter nm |
29 |
30 |
43 |
70 |
234 |
55 |
68 |
82 |
Polydispersity% (nm) |
28 (8) |
30 (9) |
31 (13) |
53 (37) |
19 (44) |
12 (7) |
15 (10) |
24 (19) |
λ resonance nm |
522 |
523 |
528 |
542 |
552 |
530 |
537 |
555 |
Table 2 Diameters, polydispersity and plasmonic resonance wavelength associated to the gold particles presented Fig. 7
|
a |
b |
c |
d |
e |
f |
R2 |
0 |
0.6 |
1 |
1.2 |
1.6 |
2 |
Diameter nm |
28 |
27 |
38 |
11 |
8.5 |
7.8 |
Polydispersity% (nm) |
27 (7) |
34 (9) |
47 (18) |
28 (3) |
24 (2) |
23 (1.8) |
λ resonance nm |
520 |
522 |
533 |
518 |
515 |
515 |
Gold NPS: effect of the polydispersity on the optical properties
The size variation of these Au@AA NPs goes with some fluctuations of the polydispersity (due to the kinetic of nucleation vs. growth). Nevertheless by comparing the extinction spectrum of Au@AA NPs and monodisperse Au@CTAB NPs with similar sizes, we have shown that, except for very high polydispersity (above 40%), the optical properties remain the same: ESI-2.†
Silver NPs: effect of ascorbic acid solutions pH
The Ag@AA NPs have been prepared using different ratios R3 = [NaOH]/[AA] varying from 1.3 to 3. For this reaction, it is necessary to reach a pH greater than 8 to reduce the silver into stable NPs. For this reason the minimal value of R3 is 1.3 (pH curve, Fig. 8).
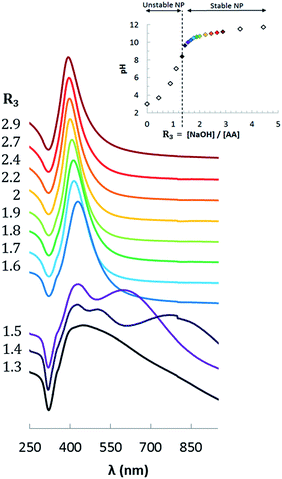 |
| Fig. 8 pH variation of the AA solution as a function of R3 = [NaOH]/[AA] and extinction spectra of the Au NPs obtained with these ratios (normalized at 250 nm). | |
The morphology of the NPs was determined from TEM measurements, as shown in Fig. 10. Their optical properties were measured by UV spectroscopy. The change of the optical properties with changing values of initial stoichiometry (R3) can be seen in Fig. 8. Fig. 9a compares the plasmonic peak positions (λmax, dots) with the NPs diameters (Fig. 10 & Table 3), while the HWHM (dots) and the polydispersity (triangles) are superimposed on Fig. 9b. We can conclude that an increase of the AA pH leads to the formation of smaller silver NPs. For R3 < 1.6, the spectra are broad and present several maxima. This behavior is due to the large size of the NPs: when the size becomes large enough, the electronic oscillations, which dictate the plasmonic response, have a multipolar mode of resonance and lead to the appearance of several peaks.36,67
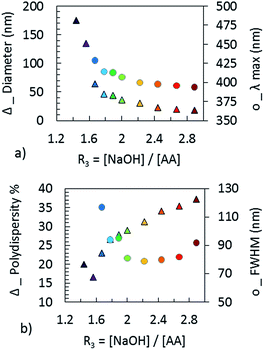 |
| Fig. 9 Comparison of (a) the diameters measured by TEM and λmax; (b) the polydispersity measured by TEM and HWHM of NPs obtained with different R3 = [NaOH]/[AA]. | |
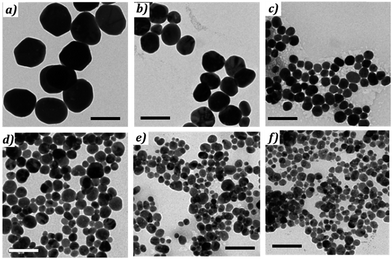 |
| Fig. 10 TEM pictures of silver NPs obtained with different R3 = [NaOH]/[AA] ratio reported in Table 3. From (a) to (c) scale bare = 100 nm, from (d) to (f) scale bare = 200 nm. | |
Table 3 Diameters, polydispersity and plasmonic resonance wavelength associated to the gold particles presented Fig. 10
|
a |
b |
c |
d |
e |
f |
R3 |
1.44 |
1.56 |
1.67 |
2 |
2.22 |
2.44 |
Diameter nm |
175 |
135 |
64 |
36 |
31 |
23 |
Polydispersity% (nm) |
20 (35) |
17 (22) |
23 (14) |
29 (10) |
31 (9) |
34 (8) |
λ resonance nm |
524 |
428 |
429 |
407 |
400 |
398 |
501 |
606 |
Due to the fast reaction rate between the silver salt and AA, we may consider that the redox potential of the metallic salt remains the same regardless of the pH. As for AA, when the pH of solution increases, its redox potential decreases. This leads to an increase in the difference of redox potentials between the silver salt and the reducing agent,68 making the reaction faster. By increasing the reactivity between the silver salt and the reducing agent, more nuclei are created which explains why the NPs are smaller at higher pH.
Surface modification of the NPs
In this section, we show that these NPs have the important advantage of been easily stabilized by a large variety of water soluble surfactants. In the following, all the data presented are obtained from the Au@AA NPs made with gold solution at pH = 2.7 and an ascorbic acid solution at pH = 2.4. The as-synthesized AA-stabilized NPs are stable over a month when left undisturbed, however as soon as an external disturbance, such as energetic stirring or centrifugation, is applied the NPs tend to aggregate. A succession of two centrifugations, followed by re-dispersion in an AA solution, leads to complete aggregation of the NPs (Fig. 11). Thus, it is clear that the AA is only a weak stabilization agent for AuNPs.
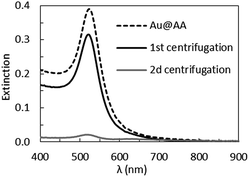 |
| Fig. 11 Extinction spectra (non normalized) of successive centrifugations of Au@AA NPs. | |
The Au@AA NPs are functionalized with ligands commonly used to stabilize metallic NPs in aqueous media69–72 (SDS, CTAB and PVP) as well as surfactants provided by Solvay: Geropon T-77®, Rhodameen PN-430®, Antarox L-64®, Igepal CO-630® and Lubrhophos LB-400®, currently applied inpersonal care and coating products, agricultural formulations, surface wetting applications and corrosion inhibition among other applications. The structures are presented in the ESI-1.† The final extinction spectra of the Au@ligands NPs are presented Fig. 12. These spectra are strikingly similar to those of the NPs after synthesis. This set of data shows that the presence of any of these surfactants on the surface of the NPs ensure short range repulsions between the NPs and prevent aggregation. This improvement of the NPs stability could be explained by two different mechanisms: exchange or wrapping. As AA appears to be weakly bound to the surface of the particles, the addition of another compound could be sufficient to initiate an exchange. If the exchange does not happen, the new surfactant may wrap and stabilized the whole Au@AA NPs.
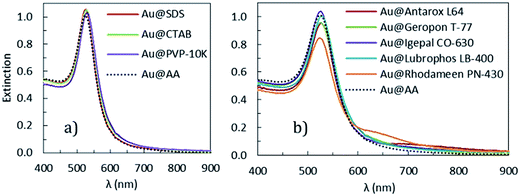 |
| Fig. 12 Final extinction spectra (non normalized) of NPs stabilized with different (a) common ligands (b) Solvay's surfactants. | |
This last section is to provide evidence of the presence of the new ligands on the particles. For PVP coated NPs, an easy way to verify that the PVP is on the surface of the NPs is to perform a silica coating surface modification using the process originally presented by Graf et al.73 In this process, PVP has both the role of stabilizing agent for the phase transfer of the NPs in ethanol as well as nucleation sites for the growth of silica. Briefly, the NPs are covered with PVP and transferred in ethanol by centrifugation. Then water, ammonia and tetraethoxysilane are added and the mixture is stirred overnight. Finally, the Au@SiO2 NPs are washed couple of times with ethanol. The presence of a silica shell around the Au NPs shown by TEM (Fig. 13) is convincing evidence PVP is around the NPs.
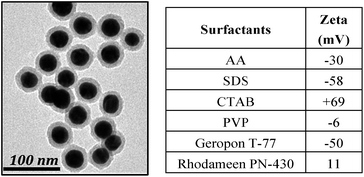 |
| Fig. 13 Au@SiO2 made from Au@AA using the Graf method + zeta potential measurements (average of 3 measurements) of different Au@ligands NPs. | |
Zeta potential measurements were performed to evaluate the relative change of the charge when a surface modification is done, and the results are presented in Fig. 13. Although these measurements need to be considered carefully in the cases of colloidal dispersions, the values of the potential of Au@ligands nevertheless show the expected trend and are consistent with the electric charges of all the commonly used surfactants (SDS, CTAB, PVP) as well as for Geropon T-77 and Rhodameen PN-430. This proves that the ligands added are surrounding the particles.
Conclusion
In this study, we have shown that ascorbic acid can be used as a reducing as well as a stabilizing agent to synthetize gold and silver NPs in a one-step aqueous procedure, at room temperature. We have also shown that by controlling either the pH of the metallic salt solution or that of the AA solutions, we were able to tune relatively well the particle sizes from 8 to 80 nm for the gold NPs, and from 20 to 175 nm for silver NPs. The distribution in size of the particles is also affected by the pH. This tunability is explained by the modification of the redox potential of each component with pH. The as-synthesized NPs are stable for more than a month and we have shown that despite their mean quality regarding size distribution, their optical properties are very similar to monodisperse particles (ESI-2†). Finally, we have also demonstrated that a facile and fast modification of the NPs surface was possible using a wide range of water based functional surfactants.
As these Au@AA particles are fast and easy to synthesize, have a good plasmonic response regarding their sizes and can be easily functionalized, they represent potentially interesting candidates for a large number of applications.
Acknowledgements
This work was supported by Complex Assemblies of Soft Matter Laboratory (COMPASS), CNRS-SOLVAY-UPENN and by the NSF through MRSEC grant DMR-1120901. C. B. M. acknowledges the Richard Perry University Professorship.
References
- S. Eustis and M. A. El-Sayed, Chem. Soc. Rev., 2006, 35, 209–217 RSC.
- P. H. Wang, M. Millard and A. G. Brolo, J. Phys. Chem. C, 2014, 118, 5889–5895 CAS.
- W. Lee, S. Mubeen, G. D. Stucky and M. Moskovits, Faraday Discuss., 2015, 178, 413–420 RSC.
- S. Mubeen, J. Lee, W. Lee, N. Singh, G. D. Stucky and M. Moskovits, ACS Nano, 2014, 8, 6066–6073 CrossRef CAS PubMed.
- M. Notarianni, K. Vernon, A. Chou, M. Aljada, J. Liu and N. Motta, Sol. Energy, 2014, 106, 23–37 CrossRef CAS.
- B. J. Roxworthy, A. M. Bhuiya, V. V. G. K. Inavalli, H. Chen and K. C. Toussaint, Nano Lett., 2014, 14, 4687–4693 CrossRef CAS PubMed.
- L. Malassis, P. Massé, M. Tréguer-Delapierre, S. Mornet, P. Weisbecker, V. Kravets, A. Grigorenko and P. Barois, Langmuir, 2013, 29, 1551–1561 CrossRef CAS PubMed.
- M. Cargnello, B. T. Diroll, E. A. Gaulding and C. B. Murray, Adv. Mater., 2014, 26, 2419–2423 CrossRef CAS PubMed.
- C. Clavero, Nat. Photonics, 2014, 8, 95–103 CrossRef CAS.
- X. Zhang, Y. L. Chen, R.-S. Liu and D. P. Tsai, Rep. Prog. Phys., 2013, 76, 046401 CrossRef PubMed.
- P. Ghosh, G. Han, M. De, C. K. Kim and V. M. Rotello, Adv. Drug Delivery Rev., 2008, 60, 1307–1315 CrossRef CAS PubMed.
- E. Boisselier and D. Astruc, Chem. Soc. Rev., 2009, 38, 1759 RSC.
- C. Ayala-Orozco, C. Urban, M. W. Knight, A. S. Urban, O. Neumann, S. W. Bishnoi, S. Mukherjee, A. M. Goodman, H. Charron, T. Mitchell, M. Shea, R. Roy, S. Nanda, R. Schiff, N. J. Halas and A. Joshi, ACS Nano, 2014, 8, 6372–6381 CrossRef CAS PubMed.
- V. Myroshnychenko, E. Carbó-Argibay, I. Pastoriza-Santos, J. Pérez-Juste, L. M. Liz-Marzán and F. J. García de Abajo, Adv. Mater., 2008, 20, 4288–4293 CrossRef CAS.
- K. A. Willets and R. P. Van Duyne, Annu. Rev. Phys. Chem., 2007, 58, 267–297 CrossRef CAS PubMed.
- K.-S. Lee and M. A. El-Sayed, J. Phys. Chem. B, 2006, 110, 19220–19225 CrossRef CAS PubMed.
- X. Ye, C. Zheng, J. Chen, Y. Gao and C. B. Murray, Nano Lett., 2013, 13, 765–771 CrossRef CAS PubMed.
- Y. Xia, Y. Xiong, B. Lim and S. E. Skrabalak, Angew. Chem., Int. Ed. Engl., 2009, 48, 60–103 CrossRef CAS PubMed.
- S. D. Perrault and W. C. W. Chan, J. Am. Chem. Soc., 2009, 131, 17042–17043 CrossRef CAS PubMed.
- H. M. Chen, R.-S. Liu and D. P. Tsai, Cryst. Growth Des., 2009, 9, 2079–2087 CAS.
- Q. Zhang, J. Xie, Y. Yu and J. Y. Lee, Nanoscale, 2010, 2, 1962–1975 RSC.
- S. E. Lohse, N. D. Burrows, L. Scarabelli, L. M. Liz-Marzán and C. J. Murphy, Chem. Mater., 2013, 26, 34–43 CrossRef.
- Z. Qian and S.-J. Park, Chem. Mater., 2014, 26, 6172–6177 CrossRef CAS.
- A. Jimenez-Ruiz, P. Perez-Tejeda, E. Grueso, P. M. Castillo and R. Prado-Gotor, Chem.–Eur. J., 2015, 21, 9596–9609 CrossRef CAS PubMed.
- J. A. Dahl, B. L. S. Maddux and J. E. Hutchison, Chem. Rev., 2007, 107, 2228–2269 CrossRef CAS PubMed.
- Z. Wang, Q. Zhang, D. Kuehner, A. Ivaska and L. Niu, Green Chem., 2008, 10, 907–909 RSC.
- J. Kasthuri, S. Veerapandian and N. Rajendiran, Colloids Surf., B, 2009, 68, 55–60 CrossRef CAS PubMed.
- C. Singh, Adv. Mater. Lett., 2012, 3, 279–285 CrossRef CAS.
- F. Cheng, J. W. Betts, S. M. Kelly, J. Schaller and T. Heinze, Green Chem., 2013, 15, 989–998 RSC.
- D.-L. Xia, Y.-F. Wang, N. Bao, H. He, X. Li, Y.-P. Chen and H.-Y. Gu, Appl. Biochem. Biotechnol., 2014, 174, 2458–2470 CrossRef CAS PubMed.
- M. Blosi, S. Albonetti, S. Ortelli, A. L. Costa, L. Ortolani and M. Dondi, New J. Chem., 2014, 38, 1401–1409 RSC.
- H. Dong, Y.-C. Chen and C. Feldmann, Green Chem., 2015, 17, 4107–4132 RSC.
- X. Ji, X. Song, J. Li, Y. Bai, W. Yang and X. Peng, J. Am. Chem. Soc., 2007, 129, 13939–13948 CrossRef CAS PubMed.
- H. Xia, S. Bai, J. Hartmann and D. Wang, Langmuir, 2010, 26, 3585–3589 CrossRef CAS PubMed.
- N. G. Bastús, J. Comenge and V. Puntes, Langmuir, 2011, 27, 11098–11105 CrossRef PubMed.
- J. Rodríguez-Fernández, J. Pérez-Juste, F. J. García de Abajo and L. M. Liz-Marzán, Langmuir, 2006, 22, 7007–7010 CrossRef PubMed.
- Y. Qin, X. Ji, J. Jing, H. Liu, H. Wu and W. Yang, Colloids Surf., A, 2010, 372, 172–176 CrossRef CAS.
- Y. Cao, R. Zheng, X. Ji, H. Liu, R. Xie and W. Yang, Langmuir, 2014, 30, 3876–3882 CrossRef CAS PubMed.
- P. Raveendran, J. Fu and S. L. Wallen, Green Chem., 2006, 8, 34–38 RSC.
- S. Li, Y. Shen, A. Xie, X. Yu, L. Qiu, L. Zhang and Q. Zhang, Green Chem., 2007, 9, 852–858 RSC.
- M. N. Nadagouda and R. S. Varma, Green Chem., 2008, 10, 859–862 RSC.
- S. L. Smitha, D. Philip and K. G. Gopchandran, Spectrochim. Acta, Part A, 2009, 74, 735–739 CrossRef CAS PubMed.
- D. Philip, C. Unni, S. A. Aromal and V. K. Vidhu, Spectrochim. Acta, Part A, 2011, 78, 899–904 CrossRef PubMed.
- V. T. P. Vinod, P. Saravanan, B. Sreedhar, D. K. Devi and R. B. Sashidhar, Colloids Surf., B, 2011, 83, 291–298 CrossRef CAS PubMed.
- S. Iravani, Green Chem., 2011, 13, 2638–2650 RSC.
- M. Noruzi, D. Zare and D. Davoodi, Spectrochim. Acta, Part A, 2012, 94, 84–88 CrossRef CAS PubMed.
- C. Singh, Adv. Mater. Lett., 2012, 3, 279–285 CrossRef CAS.
- D. Goia and E. Matijević, Colloids Surf., A, 1999, 146, 139–152 CrossRef CAS.
- S. Khatua, P. Manna, W.-S. Chang, A. Tcherniak, E. Friedlander, E. R. Zubarev and S. Link, J. Phys. Chem. C, 2010, 114, 7251–7257 CAS.
- Q. Li, J. He, E. Glogowski, X. Li, J. Wang, T. Emrick and T. P. Russell, Adv. Mater., 2008, 20, 1462–1466 CrossRef CAS.
- H. Jung, B. Koo, J.-Y. Kim, T. Kim, H. J. Son, B. Kim, J. Y. Kim, D.-K. Lee, H. Kim, J. Cho and M. J. Ko, ACS Appl. Mater. Interfaces, 2014, 6, 19191–19200 CAS.
- N. S. Abadeer, M. R. Brennan, W. L. Wilson and C. J. Murphy, ACS Nano, 2014, 8, 8392–8406 CrossRef CAS PubMed.
- L. Duchesne, D. Gentili, M. Comes-Franchini and D. G. Fernig, Langmuir, 2008, 24, 13572–13580 CrossRef CAS PubMed.
- L. Vigderman and E. R. Zubarev, Adv. Drug Delivery Rev., 2013, 65, 663–676 CrossRef CAS PubMed.
- D. Yang, J. Ma, M. Gao, M. Peng, Y. Luo, W. Hui, C. Chen, Z. Wang and Y. Cui, RSC Adv., 2013, 3, 9681–9686 RSC.
- V. Biju, Chem. Soc. Rev., 2014, 43, 744–764 RSC.
- J. Milette, V. Toader, L. Reven and R. B. Lennox, J. Mater. Chem., 2011, 21, 9043–9050 RSC.
- J. Tian, G. Liu, C. Guan and H. Zhao, Polym. Chem., 2013, 4, 1913–1920 RSC.
- W. E. Ghann, O. Aras, T. Fleiter and M.-C. Daniel, Langmuir, 2012, 28, 10398–10408 CrossRef CAS PubMed.
- E. Boisselier, L. Salmon, J. Ruiz and D. Astruc, Chem. Commun., 2008, 5788–5790 RSC.
- G. Palui, S. Ray and A. Banerjee, J. Mater. Chem., 2009, 19, 3457–3468 RSC.
- G. Park, D. Seo, I. S. Chung and H. Song, Langmuir, 2013, 29, 13518–13526 CrossRef CAS PubMed.
- Y. E. Hur, S. Kim, J.-H. Kim, S.-H. Cha, M.-J. Choi, S. Cho and Y. Park, Mater. Lett., 2014, 129, 185–190 CrossRef CAS.
- A. Mahal, M. K. Goshisht, P. Khullar, H. Kumar, N. Singh, G. Kaur and M. S. Bakshi, Phys. Chem. Chem. Phys., 2014, 16, 14257–14270 RSC.
- N. Wangoo, S. Kaur, M. Bajaj, D. V. S. Jain and R. K. Sharma, Nanotechnology, 2014, 25, 435608 CrossRef PubMed.
- A. Pinchuk, G. von Plessen and U. Kreibig, J. Phys. D: Appl. Phys., 2004, 37, 3133 CrossRef CAS.
- V. Myroshnychenko, J. Rodríguez-Fernández, I. Pastoriza-Santos, A. M. Funston, C. Novo, P. Mulvaney, L. M. Liz-Marzán and F. J. G. de Abajo, Chem. Soc. Rev., 2008, 37, 1792–1805 RSC.
- D. V. Goia, J. Mater. Chem., 2004, 14, 451–458 RSC.
- J. Rodríguez-Fernández, J. Pérez-Juste, F. J. García de Abajo and L. M. Liz-Marzán, Langmuir, 2006, 22, 7007–7010 CrossRef PubMed.
- Y.-Y. Fong, J. R. Gascooke, B. R. Visser, G. F. Metha and M. A. Buntine, J. Phys. Chem. C, 2010, 114, 15931–15940 CAS.
- C. M. Gonzalez, Y. Liu and J. C. Scaiano, J. Phys. Chem. C, 2009, 113, 11861–11867 CAS.
- Q. Zhang, C. Cobley, L. Au, M. McKiernan, A. Schwartz, L.-P. Wen, J. Chen and Y. Xia, ACS Appl. Mater. Interfaces, 2009, 1, 2044–2048 CAS.
- C. Graf, D. L. J. Vossen, A. Imhof and A. van Blaaderen, Langmuir, 2003, 19, 6693–6700 CrossRef CAS.
Footnote |
† Electronic supplementary information (ESI) available. See DOI: 10.1039/c6ra00194g |
|
This journal is © The Royal Society of Chemistry 2016 |