DOI:
10.1039/C5RA27898H
(Paper)
RSC Adv., 2016,
6, 28470-28476
Enhanced binding capacity of boronate affinity fibrous material for effective enrichment of nucleosides in urine samples†
Received
28th December 2015
, Accepted 4th March 2016
First published on 7th March 2016
Abstract
In this work, polyethyleneimine-modified boronate affinity fibrous cotton with good selectivity and higher binding capacity for cis-diols was developed for in-pipette-tip solid-phase extraction (SPE). The introduction of polyethyleneimine for the modification of cotton fiber provided abundant binding sites for adsorption of cis-diols, resulting in higher binding capacities up to 300 μg g−1 for catechol, 450 μg g−1 for dopamine and 700 μg g−1 for adenosine, respectively. The adsorbent could still show recognition ability towards cis-diols even with a 1000-fold amount of interferences in the solution. Several extraction parameters were optimized, including adsorbent dose, pipette times, washing and elution solvents. Under optimal extraction conditions, the proposed in-pipette-tip SPE method coupled with reversed-phase liquid chromatography was successfully used to extract nucleosides in urine. The limit of detection was 3.5–4.7 ng mL−1 and the recoveries were in the range of 89–102% with relative standard deviations of less than 9.9%. In conclusion, combination of the specific adsorption performance of the prepared boronate affinity fibrous material and in-pipette-tip SPE provided a fast pretreatment method of trace cis-diols in complex real samples.
1. Introduction
Owing to the unique reversible covalent esterification/hydroxylation between boronic acids on the surface of an adsorbent and cis-diol-containing molecules in alkaline/acidic aqueous media, boronate affinity materials could be employed in the selective recognition and enrichment of cis-diol biomolecules, such as carbohydrates, glycoproteins, catechols, nucleosides and glycopeptides.1–9 For the preparation of boronate affinity materials, the common approach is to graft boronate affinity ligands onto the surface of various support matrixes. At present, the widely used matrixes include mesoporous silica,10 magnetic nanoparticles,11–14 polymeric nanospheres15 and nanotubes.16,17 However, the preparation processes of these matrixes are rather complex and often highly consumptive of organic solvents and time-consuming. In comparison with synthetic matrixes, natural materials possess obvious superiorities such as easy of availability, low-cost and biodegradability.
As a kind of natural vegetable product, cotton has been utilized as a solid-phase extraction (SPE) adsorbent for sample pretreatment over the past few years. The unmodified cotton fiber could not only enrich some hydrophobic compounds, such as oil, colorants and polycyclic aromatic hydrocarbons,18–24 but also purify glycan and glycopeptides.25 Viewed from the present application effect, cotton-based adsorbents generally show good adsorption performance as well as biocompatibility, high mechanical strength and stability, however, the targets capable of being adsorbed were limited due to the presence of only hydroxyl groups on the surface of the cotton fiber.
To broaden the kinds of adsorbed substances, modified cotton fibers were developed for selective adsorption of special substances, such as blue cotton for polycyclic aromatic hydrocarbons,26 sulfhydryl cotton fiber (SCF) for methylmercury,27 and a carboxyl cotton chelator for metal ions in environmental water.28 More recently, Feng et al. reported several cotton-based fibrous adsorbents modified with phenylboronic acid for selectively capturing glycopeptides,29 with immobilized nickel(II) for his-tagged proteins,30 and with immobilized zirconium29 or titanium(IV) for phosphopeptides.31 In spite of these advances in cotton-based adsorbents, the binding capacities of the cotton-based materials are still lower due to the limited functional ligands on the surface of the adsorbents, and need to be improved.
As one of the main characteristics, the binding capacity affects adsorption performance.32 Because the binding capacity depends on the density of the functional groups on the surface of a given material, attention begins to be focused on how to enhance the density of functional groups. As is well known, the modification of materials with functional polymers can achieve this purpose. Ye and our group prepared a high-capacity boronate affinity adsorbent by grafting boronic acid polymer chains on the surface of silica via surface-initiated atom transfer radical polymerization.33,34 Since the polymer brushes contain the repeating functional group units, the prepared adsorbents usually exhibit high binding capacity towards cis-diol-containing compounds. In addition, a dendrimer was also used in the functionalization of the boronate affinity adsorbent.35,36 However, these two methods are complex in the operation.
As a kind of easily available and cheap coating polymer, polyethyleneimine (PEI) has been used to functionalize the surface of inorganic nanoparticles, silica and polymeric carriers.37–39 Because of the abundant amino groups within the molecule, PEI can endow the adsorbent with a high density of active binding sites, which can significantly increase the binding amount of functional ligands on the surface of the adsorbent.40 In addition, the abundant amino groups within the PEI molecule can improve the hydrophilicity of adsorbents, contributing to the elimination of nonspecific adsorption.41 Therefore, the construction of PEI gives it potential to enhance the density of interesting functional ligands on the surface of cotton fiber.
The purpose of this paper is to combine the features of cotton and PEI to develop a boronate affinity material with high binding capacity for cis-diol-containing compounds. Considering that fibrous adsorbents have good toughness, we employed the boronate affinity cotton fiber in the in-pipette-tip miniaturized SPE mode to facilitate the determination process. Then, the extraction selectivity and binding capability of the adsorbent were investigated, and several operational parameters that affect the extraction efficiency were optimized, including adsorbent dose, extraction times, washing and elution solvent. Finally, the in-pipette-tip SPE coupled with high performance liquid chromatography (HPLC) was applied in the determination of trace nucleosides in human urine to demonstrate the feasibility of the proposed method.
2. Experimental
2.1. Instruments
The morphology of the adsorbent was characterized by scanning electron microscopy (SEM, ZEISS EV018, Germany). The X-ray photoelectron spectroscopy (XPS) analysis (K-Alpha, thermoFisher Scientific, USA) was used to investigate the chemical composition.
A Shimadzu HPLC system (Kyoto, Japan), consisting of two LC-10ATvp pumps, a SCL-10A system controller, a UV-vis detector and a CLASS VP 5.03 chromatographic workstation was employed for all experiments. All separations were carried out on a VP-ODS column (4.6 mm × 150 mm) at room temperature.
2.2. Materials and reagents
Cotton wool was purchased from Yangxue Sanitary Material Co. Ltd (Shandong, China). 2,2′-Azobis(2-methylpropionitrile) (AIBN) was purified by recrystallization from ethanol at 40 °C. PEI (MW = 600 Da), N-hydroxysuccinimide, dopamine hydrochloride, sodium cyanoborohydride, guanosine and adenosine were purchased from Aladdin Chemical Reagent Co. Ltd (Shanghai, China); dicyclohexylcarbodiimide was purchased from Sinopharm Chemical Reagent Co. Ltd (Shanghai, China); 4-formylphenylboronic acid, cytidine and uridine were purchased from J&K Chemical Reagent Co. Ltd (Beijing, China). Other reagents were all of analytical grade.
2.3. Preparation of the boronate affinity cotton fiber
SCF was synthesized by the reported method,27 with minor modification. 20 mL of mercaptoacetic acid, 12 mL of acetic anhydride, 8 mL of 36% acetic acid and 0.06 mL of concentrated sulfuric acid were added into a round-bottom flask in sequence. After the mixture was cooled to room temperature, 3.0 g of cotton wool was added. The reaction proceeded at 45 °C for 3 days. The resulting SCF was washed with 1 L deionized water and 200 mL ethanol in sequence and dried at 40 °C for 24 h under vacuum.
The preparation procedure for boronate affinity cotton fiber (SCF@PEI@PBA) is illustrated in Fig. 1. 250 mg of SCF was immersed in 30 mL of N,N-dimethylformamide (DMF), then 5.0 mL of methylacrylate and 1 wt% AIBN were added. The mixture was degassed with ultrasonication for 10 min and then stirred at 60 °C for 20 h under nitrogen atmosphere. The obtained product was washed with DMF and deionized water and then immersed in a 30 mL aqueous solution containing 50 μL trifluoroacetic acid (TFA) under stirring at room temperature for 7 h. The resulting SCF@COOH was washed with deionized water and ethanol in sequence and dried at 50 °C for 24 h under vacuum. After dissolving PEI (0.3 g) into dried dimethyl sulfoxide (DMSO, 30 mL), SCF@COOH, dicyclohexylcarbodiimide (140 mg) and N-hydroxysuccinimide (70 mg) were added, and the mixture was dispersed by ultrasonic treatment. After stirring for 24 h at room temperature, the achieved SCF@PEI was washed with DMSO, deionized water and ethanol in succession, and then dried at 50 °C for 8 h under vacuum.
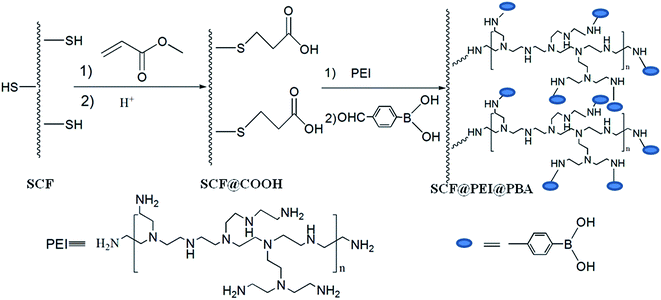 |
| Fig. 1 The route for synthesis of SCF@PEI@PBA. | |
The obtained SCF@PEI was re-dispersed in 30 mL of dried methanol under ultrasonication, and then 1.5 g of 4-formylphenylboronic acid and 1.2 g of sodium cyanoborohydride were added. After stirring at room temperature for 72 h, the product was washed with 5% NaHCO3, 5% NaCl, deionized water and ethanol in order. Finally, the obtained SCF@PEI@PBA was dried at 45 °C under vacuum.
2.4. Determination of binding capacity and selectivity
Catechol, dopamine and adenosine were selected as the model compounds for cis-diols to measure the binding capacity. 10 mg of SCF@PEI@PBA was suspended in a series of 2 mL standard solutions of each analyte with different concentration. The solution was shaken for 30 min at room temperature, and the supernatant was analyzed by HPLC-UV. In the plot of adsorption amount with peak area, the turning point corresponds to the maximum binding capacity of SCF@PEI@PBA.
To evaluate the selectivity, the mixture was prepared by adding 2′-deoxyguanosine and thymidine as interferences at a concentration of 1-fold, 10-fold, 100-fold and 1000-fold into 100 ng mL−1 of uridine and guanosine. The extraction procedure was the same as the following in-pipette-tip SPE procedure.
2.5. In-pipette-tip SPE procedure
10 mg of the obtained SCF@PEI@PBA was packed into a 1 mL commercial pipette tip. 1 mL of the sample solution (50 ng mL−1) was pipetted up and down 40 times. After 100 μL of washing solution (NH3·H2O–acetonitrile (ACN), 1
:
99, v/v) was pipetted up and down five times, the adsorbent was pipetted up and down five times by 100 μL of desorption solvent (TFA–H2O, 1
:
99, v/v). Finally, the eluent was filtered through a 0.45 μm nylon membrane for analysis by HPLC.
2.6. Sample pretreatment
A urine sample was collected from a healthy volunteer and added with sodium metabisulfite (2 mg mL−1). The utilization of human urine complied with guidelines of Ethics Committee of the Institute, and the volunteer gave her informed consent. Then, the solution was diluted 5 fold with deionized water and stored in refrigerator prior to use. The extraction procedure was the same as mentioned above.
3. Results and discussion
3.1. Preparation and characterization of the SCF@PEI@PBA fiber
As shown in Fig. 1, there are four steps during the preparation of the SCF@PEI@PBA fiber: (1) preparation of SCF; (2) functionalization of SCF with carboxyl; (3) modification of PEI onto the surface of SCF; (4) grafting of boronic acid onto the surface of SCF@PEI by the Schiff base reaction. All steps are convenient to operate under mild reaction conditions.
Surface morphology of cotton, SCF, SCF@PEI and the SCF@PEI@PBA were characterized by SEM, as shown in Fig. 2. The diameters of four kinds of fibers were kept approximately the same, with sizes in the range of 10 to 20 μm, indicating that fibrous toughness was well kept without disruption of the fibrous morphology in the reaction process, thus, the as-prepared SCF@PEI@PBA fiber was able to pack in a pipette-tip.
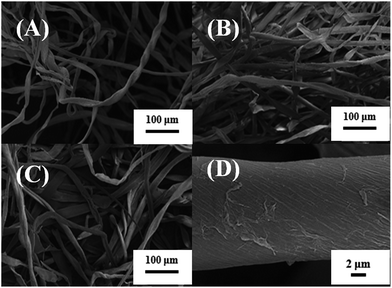 |
| Fig. 2 SEM images of (A) cotton, (B) SCF, (C) SCF@PEI and (D) SCF@PEI@PBA. | |
Fig. 3 shows the XPS of cotton, SCF, SCF@PEI and SCF@PEI@PBA and Table 1 lists the calculated element content. The S content of SCF was 1.8%, while there was no S element in cotton, confirming the successful immobilization of the thiol group onto cotton. No N element in SCF was found, whereas the N content in SCF@PEI was raised to 4.6% after the immobilization of PEI onto SCF. Furthermore, the appearance of the B1s peak at 191.5 eV in SCF@PEI@PBA suggested the successful attachment of boronic acid groups onto SCF@PEI (Fig. 3 inset plot), and the B content was calculated to be 3.0%.
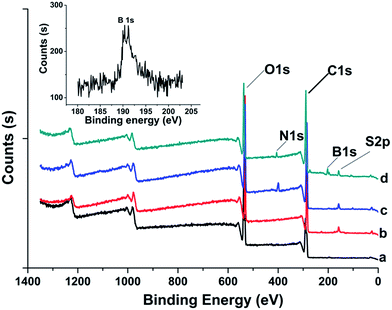 |
| Fig. 3 XPS spectrum of (a) cotton, (b) SCF, (c) SCF@PEI and (d) SCF@PEI@PBA. The inset spectrum shows the peak of B1s. | |
Table 1 Elemental contents obtained by XPS
Samples |
C1s (%) |
O1s (%) |
S1s (%) |
N1s (%) |
B1s (%) |
Cotton |
70.1 |
29.9 |
0 |
— |
— |
SCF |
66.4 |
31.8 |
1.8 |
— |
— |
SCF@PEI |
66.6 |
27.4 |
1.4 |
4.6 |
— |
SCF@PEI@PBA |
66.4 |
25.7 |
0.9 |
4.0 |
3.0 |
3.2. Binding capacity and selectivity
The binding capacities of the adsorbent towards catechol, dopamine and adenosine were evaluated in pH range of 7.0–9.5. As shown in Fig. S1,† the binding capacities towards dopamine and adenosine increased from 7.0 to 8.5, while they decreased slightly at pH 9.0 and were kept constant at pH 9.5. Meanwhile the binding capacity towards catechol increased from pH 7.0 to 9.0, and was kept constant at pH 9.5. This phenomenon was in good agreement with the theoretical expectation that a basic pH condition favors the formation of covalent bonds between cis-diols and boronic acids. On the other hand, the abundant amino groups of PEI possibly had electrostatic interaction with the analytes. To exclude the possibility of hydrolysis of cotton in strong alkaline solution, the sample solution was adjusted to pH 8.5 in the following experiments. At pH 8.5, the binding capacity of SCF@PEI@PBA was estimated to be 300 μg g−1 for catechol, 450 μg g−1 for dopamine and 700 μg g−1 for adenosine, respectively (Fig. S2†). And due to the higher density of active binding sites, the adsorption capacity of the SCF@PEI@PBA fiber was much higher than that of the reported cotton fiber modified directly with boronic-acid ligand (65 μg g−1 for adenosine).29
The extraction selectivity of the SCF@PEI@PBA fiber was demonstrated by using uridine, guanosine, 2′-deoxyguanosine and thymidine as tested solutes. The mixture was extracted with in-pipette-tip SPE and the eluent was analyzed by a HPLC system. As shown in Fig. 4, even if the molar ratio of non-cis-diols to cis-diols increased from 1
:
1 to 1000
:
1 based on the concentration of cis-diols remaining constant, only cis-diol containing uridine and guanosine are captured by the SCF@PEI@PBA fiber, whereas non-cis-diols, 2′-deoxyguanosine and thymidine, are all excluded. Notably, the signal intensities of the extracted uridine and guanosine are nearly stable when the amounts of interferences are raised. Thus, the prepared SCF@PEI@PBA fiber presented specific selectivity towards cis-diols over non-cis-diols.
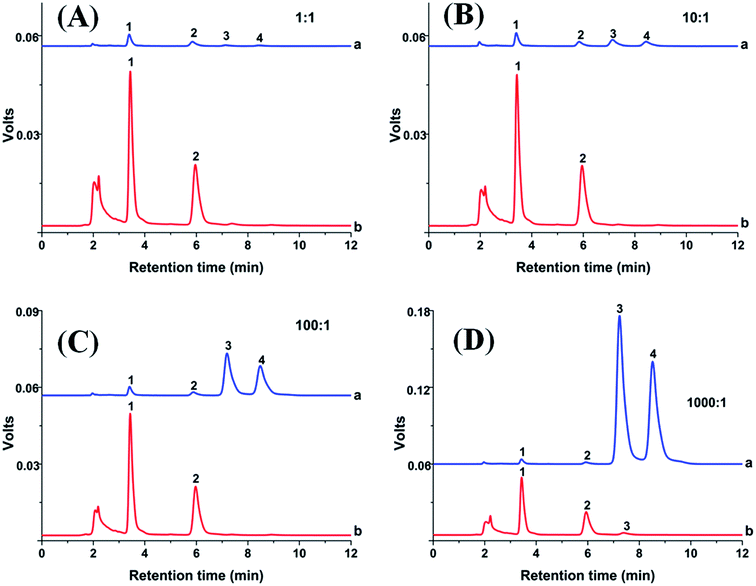 |
| Fig. 4 Chromatograms of the mixture analyzed (a) before and (b) after enrichment. Concentration ratios of non-cis-diol to cis-diol-containing compounds: (A) 1 : 1, (B) 10 : 1, (C) 100 : 1 and (D) 1000 : 1. Peaks: (1) uridine, (2) guanosine, (3) 2′-deoxyguanosine, and (4) thymidine. Mobile phase: 10% methanol–90% NaH2PO4 (10 mM, pH 4.3). | |
3.3. The selective enrichment of nucleosides in urine samples
3.3.1. Optimization of the extraction conditions. SCF@PEI@PBA was utilized as an adsorptive material in an in-pipette-tip SPE. The extraction recovery was used to evaluate the effect of extraction parameters on the extraction performance, including adsorbent dose, pipette times, washing and elution solvents.The effect of adsorbent dose on the extraction recovery was studied in the range of 4–12 mg. As illustrated in Fig. S3A,† the extraction recovery increased with increasing adsorbent dose, and reached maximum at 8 mg. When the adsorbent dose continued to increase, the extraction recovery no longer increased. Therefore, 8 mg of SCF@PEI@PBA was selected as a reasonable dose for the in-pipette-tip SPE. Extraction is an equilibrium technique, in which analytes are partitioned between sample solution and the adsorbent. The extraction recovery increased with increasing extraction times from 20 to 40, and then basically kept constant (Fig. S3A†). This means that the adsorption equilibrium was reached at about 40 pipette times.
The washing step aims to reduce the impurity interference and maximize the specific interactions between the analytes and adsorbent. The washing solvents are crucial to reach this purpose. In this work, seven kinds of washing solvents were investigated, including NH3·H2O–ACN, NH3·H2O–H2O, NH3·H2O–ethanol, NH3·H2O–methanol, NH3·H2O–acetone, NH3·H2O–tetrahydrofuran and NH3·H2O–DMF. Among these washing solvents, NH3·H2O–ACN yielded the highest extraction recoveries to the analytes (Fig. S3B†). Furthermore, the ratio of NH3·H2O–ACN affected the recovery of analytes. The extraction recoveries reached maximum at a NH3·H2O–ACN ratio of 5
:
95 (v/v), whereas the recoveries decreased slightly as the ratio continued to increase. Therefore, 5% NH3·H2O–95% ACN was selected as the optimal washing solvent.
In a typical SPE, the elution solvent directly decides whether analytes could be completely eluted from the adsorbent, and finally affects the extraction efficiency. Three types of elution solvents, TFA–H2O, formic acid–H2O and acetic acid–H2O were investigated. Obviously, TFA–H2O achieved the best desorption efficiency (Fig. S3C†). Furthermore, a higher TFA concentration favored desorption. The extraction recoveries increased sharply in the concentration range from 1% to 5% TFA, and raised slowly from 5% to 9% TFA (Fig. S3C†). Considering that cotton fiber should be used under a relatively mild acidic condition, 5% TFA solution was selected as the elution solvent in the in-pipette-tip SPE.
3.3.2. Determination of nucleosides in urine. Based on the obtained optimum conditions, the proposed in-pipette-tip SPE method coupled with HPLC was used to determine four nucleosides (uridine, cytidine, guanosine and adenosine) in urine. Fig. 5 shows the chromatograms of untreated urine, the urine and the spiked urine treated with the in-pipette-tip SPE. It was observed that the urine sample with direct injection presented many undefined interfering peaks, whereas these interfering peaks disappeared after the urine was enriched by the in-pipette-tip SPE. Therefore, the SCF@PEI@PBA adsorbent has good feasibility to selectively extract nucleosides from practical samples. The standard addition method was employed to perform the quantification. The analytical performance was investigated regarding linearity, precision and sensitivity (Table 2). Good linearity was achieved in the range of 20–800 ng mL−1 with squared regression coefficients (R2) ranging from 0.9992 to 0.9995. The limit of detection (LOD) and limit of quantification (LOQ) were expressed as the concentration of nucleosides based on a signal-to-noise ratio of 3 and 10, and the measured values were 3.5–4.7 ng mL−1 and 11.7–15.7 ng mL−1, respectively. The precision was also calculated by analyzing the urine samples spiked with 50 ng mL−1, and the relative standard deviations (RSDs) were in the range of 2.3–6.6% for run-to-run and 4.2–13.2% for column-to-column (n = 5), respectively. In the urine of the volunteer, the concentrations of four nucleosides were found to be 26.1 ng mL−1 for cytidine, 12.8 ng mL−1 for uridine, 18.1 ng mL−1 for guanosine and 34.5 ng mL−1 for adenosine, respectively.
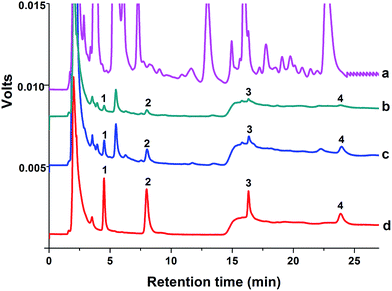 |
| Fig. 5 Chromatograms of nucleosides. (a) Blank urine; (b) blank urine after enrichment; (c) spiked urine with 20 ng mL−1 after enrichment; (d) mixed standard solution of 500 ng mL−1. Peaks: (1) cytidine, (2) uridine, (3) guanosine and (4) adenosine. Gradient elution condition: 0–10 min, 2% methanol–98% NaH2PO4 (10 mM, pH 4.3); 10–35 min, 10% methanol–90% NaH2PO4. | |
Table 2 Linearity characteristics of the determination of nucleosides using the proposed in-pipette-tip SPE mode
Analytes |
Conc. (ng mL−1) |
Linear range (ng mL−1) |
R2 |
LOD (ng mL−1) |
LOQ (ng mL−1) |
RSD (%, n = 5) |
Run-to-run |
Column-to-column |
Cytidine |
26.1 |
20–800 |
0.9993 |
3.5 |
11.7 |
2.3 |
6.4 |
Uridine |
12.8 |
20–800 |
0.9995 |
3.8 |
12.7 |
2.6 |
4.2 |
Guanosine |
18.1 |
20–800 |
0.9992 |
4.1 |
13.7 |
6.6 |
10.4 |
Adenosine |
34.5 |
20–800 |
0.9995 |
4.7 |
15.7 |
3.4 |
13.2 |
In order to evaluate the applicability of the proposed method, the validity of the method was validated (Table 3). The urine samples spiked with high (100 ng mL−1), medium (50 ng mL−1) and low (20 ng mL−1) levels of nucleosides were analyzed by the in-pipette-tip SPE-HPLC method. The recoveries at different spiking levels were in the range of 89% to 102% with RSDs from 1.7 to 9.9% (n = 3).
Table 3 Average recoveries and RSDs (n = 3) of spiked nucleosides in human urine with the in-pipette-tip SPE mode
Analytes |
20 ng mL−1 |
50 ng mL−1 |
100 ng mL−1 |
Recovery (%) |
RSD (%) |
Recovery (%) |
RSD (%) |
Recovery (%) |
RSD (%) |
Cytidine |
102 |
3.9 |
89 |
4.7 |
101 |
1.7 |
Uridine |
93 |
8.1 |
97 |
5.6 |
99 |
4.8 |
Guanosine |
99 |
5.2 |
95 |
2.6 |
98 |
7.5 |
Adenosine |
96 |
9.9 |
98 |
7.1 |
102 |
8.3 |
Finally, the proposed method was compared with the previously reported ones for nucleoside analysis (Table 4). The SCF@PEI@PBA based in-pipette-tip SPE/HPLC method provided lower LODs than the reported methods along with similar accuracy and recovery. Therefore, our method showed better analytical performance.
Table 4 Comparison of the present method with the reported methods for the determination of nucleosides in urine
Sample treatment |
Analytical technique |
Recovery (%) |
LOD (ng mL−1) |
RSD (%) |
Reference |
SPE with Varian PBA column |
LC-MS/MS |
97.76–107.51 |
80–180 |
1.18–11.04 |
42 |
SPE with Oasis MCX |
LC-MS/MS |
— |
30–75 |
— |
43 |
SPE with Affi-Gel 601 |
CE-UV |
83.8–113 |
217–405 |
5.7–7.2 |
44 |
SPE with Affi-Gel 601 |
MEKC-UV |
85.85–117.08 |
40–210 |
1.86–26.40 |
45 |
SPE with Affi-Gel 601 |
CE-MS |
— |
5.03–498 |
— |
46 |
In-pipette-tip SPE with SCF@PEI@PBA |
LC-UV |
89–102 |
3.5–4.7 |
1.7–9.9 |
This work |
4. Conclusion
In this study, the prepared boronate affinity fibrous adsorbent for selective extraction of nucleosides in urine samples was reported. Cotton was utilized as the base material, which was much cheaper and degradable, and thus, kind to the environment. The introduction of PEI onto cotton fiber significantly increased the density of amino groups, and thus provided more binding sites for grafting functional ligands. Owing to the ability of fibrous materials to be packed into the pipette-tip, the miniaturized SPE was performed to simplify the determination process quickly. Notably, the proposed strategy exhibited good selectivity, higher sensitivity, satisfactory accuracy and reproducibility, and thus has potential application in determination of trace cis-diols in complex biological analysis.
Acknowledgements
This work was supported by the National Natural Science Foundation of China (No. 21275115, 21475104 and 21575114).
References
- S. Y. Xu, H. C. Wang, S. E. Flower, J. S. Fossey, Y. B. Jiang and T. D. James, RSC Adv., 2014, 4, 35238–35241 RSC.
- Z. Lin, J. L. Pang, H. H. Yang, Z. W. Cai, L. Zhang and G. N. Chen, Chem. Commun., 2011, 47, 9675–9677 RSC.
- G. H. Jiang, T. T. Jiang, Y. Wang, X. X. Du, Z. Wei and H. J. Zhou, RSC Adv., 2014, 4, 33658–33661 RSC.
- Y. Q. Jiang and Y. F. Ma, Anal. Chem., 2009, 81, 6474–6480 CrossRef CAS PubMed.
- M. Chen, Y. Lu, Q. Ma, L. Guo and Y. Q. Feng, Analyst, 2009, 134, 2158–2164 RSC.
- X. H. Zhang, J. W. Wang, X. W. He, L. X. Chen and Y. K. Zhang, ACS Appl. Mater. Interfaces, 2015, 7, 24576–24584 CAS.
- S. T. Zhang, X. W. He, L. X. Chen and Y. K. Zhang, New J. Chem., 2014, 38, 4212–4218 RSC.
- F. Yang, J. Mao, X. W. He, L. X. Chen and Y. K. Zhang, Anal. Bioanal. Chem., 2013, 405, 6639–6648 CrossRef CAS PubMed.
- F. Yang, J. Mao, X. W. He, L. X. Chen and Y. K. Zhang, Anal. Bioanal. Chem., 2013, 405, 5321–5331 CrossRef CAS PubMed.
- Y. W. Xu, Z. Q. Wu, L. J. Zhang, H. J. Lu, P. Y. Yang, P. A. Webley and D. Y. Zhao, Anal. Chem., 2009, 81, 503–508 CrossRef CAS PubMed.
- N. Ž. Knežević, E. R. Hernández, W. E. Hennink and M. V. Regí, RSC Adv., 2013, 3, 9584–9593 RSC.
- W. Zhou, N. Yao, G. P. Yao, C. H. Deng, X. M. Zhang and P. Y. Yang, Chem. Commun., 2008, 5577–5579 RSC.
- K. Sparbier, A. Asperger, A. Resemann, I. Kessler, S. Koch, T. Wenzel, G. Stein, L. Vorwerg, D. Suckau and M. Kostrzewa, J. Biomol. NMR, 2007, 18, 252–258 Search PubMed.
- K. Sparbier, S. Koch, I. Kessler, T. Wenzel and M. Kostrzewa, J. Biomol. NMR, 2005, 16, 405–411 Search PubMed.
- X. J. Zou, D. Liu, L. J. Zhong, B. Yang, Y. X. Lou and Y. X. Yin, Carbohydr. Polym., 2012, 90, 799–804 CrossRef CAS PubMed.
- S. R. Ali, R. R. Parajuli, Y. Balogun, Y. F. Ma and H. X. He, J. Sens., 2008, 8, 8423–8452 CrossRef CAS.
- A. Vlandas, T. Kurkina, A. Ahmad, K. Kern and K. Balasubramanian, Anal. Chem., 2010, 86, 6090–6097 CrossRef PubMed.
- M. T. O. Jonker, Chemosphere, 2008, 70, 778–782 CrossRef CAS PubMed.
- G. Deschamps, H. Caruel, M. E. Borredon, C. Bonnin and C. Vignoles, Environ. Sci. Technol., 2003, 37, 1013–1015 CrossRef CAS PubMed.
- R. F. Johnson and T. G. Manjrekar, Environ. Sci. Technol., 1973, 7, 439–443 CrossRef CAS PubMed.
- H. M. Choi and H. J. Kwon, Text. Res. J., 1993, 63, 211–218 CrossRef.
- J. P. Wang, S. Q. Liu, C. Y. Chen, Y. Zou, H. P. Hu, Q. Y. Cai and S. Z. Yao, Analyst, 2014, 139, 3593–3599 RSC.
- J. F. Liu, Y. G. Chi, G. B. Jiang, C. Tai and J. T. Hu, Microchem. J., 2004, 77, 19–22 CrossRef CAS.
- M. Gonzalez, M. Gallego and M. Valcarcel, Anal. Chem., 2003, 75, 685–693 CrossRef CAS PubMed.
- M. H. J. Selman, M. Hemayatkar, A. M. Deelder and M. Wuhrer, Anal. Chem., 2011, 83, 2492–2499 CrossRef CAS PubMed.
- Y. Takagai, R. Akiyama and S. Igarashi, Anal. Bioanal. Chem., 2006, 385, 888–894 CrossRef CAS PubMed.
- V. Celo, R. V. Ananth, S. L. Scott and D. R. S. Lean, Anal. Chim. Acta, 2004, 516, 171–177 CrossRef CAS.
- R. M. Gong, Y. Hu, J. Chen, F. Y. Chen and Z. L. Liu, Microchim. Acta, 2007, 158, 315–320 CrossRef CAS.
- X. M. He, G. T. Zhu, Y. Y. Zhu, X. Chen, Z. Zhang, S. T. Wang, B. F. Yuan and Y. Q. Feng, ACS Appl. Mater. Interfaces, 2014, 6, 17857–17864 CAS.
- X. M. He, G. T. Zhu, W. Lu, B. F. Yuan, H. Wang and Y. Q. Feng, J. Chromatogr. A, 2015, 1405, 188–192 CrossRef CAS PubMed.
- X. M. He, X. Chen, G. T. Zhu, Q. Wang, B. F. Yuan and Y. Q. Feng, ACS Appl. Mater. Interfaces, 2015, 7, 17356–17362 CAS.
- Q. J. Li, C. C. Lu, H. Y. Li, Y. C. Liu, H. Y. Wang, X. Wang and Z. Liu, J. Chromatogr. A, 2012, 1256, 114–120 CrossRef CAS PubMed.
- Z. F. Xu, K. M. A. Uddin, T. Kamra, J. Schnadt and L. Ye, ACS Appl. Mater. Interfaces, 2014, 6, 1406–1414 CAS.
- W. Wang, M. F. He, C. Z. Wang and Y. M. Wei, Anal. Chim. Acta, 2015, 886, 66–74 CrossRef CAS PubMed.
- H. Y. Wang, Z. J. Bie, C. C. Lü and Z. Liu, Chem. Sci., 2013, 4, 4298–4303 RSC.
- L. Gao, C. Z. Wang and Y. M. Wei, RSC Adv., 2015, 5, 106161–106170 RSC.
- Y. K. Buchman, E. Lellouche, S. Zigdon, M. Bechor, S. Michaeli and J. P. Lellouche, Bioconjugate Chem., 2013, 24, 2076–2087 CrossRef CAS PubMed.
- T. Xia, M. Kovochich, M. Liong, H. Meng, S. Kabehie, S. George, J. I. Zink and A. E. Nel, ACS Nano, 2009, 3, 3273–3286 CrossRef CAS PubMed.
- K. H. Chen, J. X. Zhang and H. C. Gu, J. Mater. Chem., 2012, 22, 22005–22012 RSC.
- X. W. Zhang, J. Yang, S. F. Liu, X. C. Lin and Z. H. Xie, J. Sep. Sci., 2011, 34, 3383–3391 CrossRef CAS PubMed.
- R. Y. Zhang, Q. Li, Y. Gao, J. Li, Y. D. Huang, C. Song, W. Q. Zhou, G. H. Ma and Z. G. Su, J. Chromatogr. A, 2014, 1343, 109–118 CrossRef CAS PubMed.
- W. Struck, D. Siluk, A. Y. Mpanga, M. Markuszewski, R. Kaliszan and M. J. Markuszewski, J. Chromatogr. A, 2013, 1283, 122–131 CrossRef CAS PubMed.
- L. B. Jeng, W. Y. Lo, W. Y. Hsu, W. D. Lin, C. T. Lin, C. C. Lai and F. J. Tsai, Rapid Commun. Mass Spectrom., 2009, 23, 1543–1549 CrossRef CAS PubMed.
- Y. Q. Jiang and Y. F. Ma, Anal. Chem., 2009, 81, 6474–6480 CrossRef CAS PubMed.
- E. Szymańska, M. J. Markuszewski, K. Bodzioch and R. Kaliszan, J. Pharm. Biomed. Anal., 2007, 44, 1118–1126 CrossRef PubMed.
- S. F. Wang, X. P. Zhao, Y. Mao and Y. Y. Cheng, J. Chromatogr. A, 2007, 1147, 254–260 CrossRef CAS PubMed.
Footnote |
† Electronic supplementary information (ESI) available. See DOI: 10.1039/c5ra27898h |
|
This journal is © The Royal Society of Chemistry 2016 |
Click here to see how this site uses Cookies. View our privacy policy here.