DOI:
10.1039/C5RA27819H
(Paper)
RSC Adv., 2016,
6, 33755-33762
High-contrast mechanochromism and polymorphism-dependent fluorescence of difluoroboron β-diketonate complexes based on the effects of AIEE and halogen†
Received
27th December 2015
, Accepted 25th March 2016
First published on 29th March 2016
Abstract
Tetraphenylethylene (TPE) is known for its aggregation-induced emission enhancement (AIEE) effect due to the restriction of intramolecular rotation. Some halogen-substituted fluorophores show interesting luminescent phenomena via C–H⋯halogen interactions. By combining TPE units, a halogen with a difluoroboron β-diketonate fluorophore, three complexes, 2a, 2b and 2c, were designed and synthesized. All of them exhibited intramolecular charge transfer, high fluorescence quantum yields, AIEE characteristics and high-contrast mechanochromism. By either grinding-fuming or grinding–annealing cycles, the pristine state or the original emission color of the three complexes can be almost recovered reversibly. In particular, 2c exhibits spontaneous reversibility after grinding at room temperature. Owing to the effect of halogen (Cl, Br), 2b and 2c show polymorphism-dependent fluorescence (yellow plate-like crystals and green needlelike crystals) and higher contrast than 2a before/after grinding. The maximum fluorescence emission wavelength of 2b and 2c shifts bathochromically 70 nm and 59 nm after grinding, respectively. To reveal the mechanochromic mechanism, a series of tests including powder XRD, fluorescence lifetime, DSC, and solid state UV-visible absorbance were completed. The results indicate that mechanochromism of the complexes can be correlated with the transformation between the amorphous and the crystalline states and metastable state formation after grinding, rather than molecular planarization, excimer or exciplex formation. To the best of our knowledge, this is the first example of no alkyl- or alkoxy-substituted difluoroboron β-diketonate complexes that exhibit reversible mechanochromic luminescence.
Introduction
The significance of stimuli-responsive materials (SR) lies not only in fundamental research but also in potential applications. Currently, the application fields of SR have covered sensors,1–6 memory chips,7–10 security inks,11 data storage,12–14 deformation detectors,15 camouflaging and optoelectronic devices,16–18 and so forth. According to the different stimulus methods, SR can be divided into mechano-, piezo-, thermo-, photo-, electro- and vapochromism. Among of them, the reversible mechanofluorochromic (MFC) materials have become a research focus, whose emitting colours or intensity can be changed to external mechanical force (such as grinding, scraping, rubbing, etc.), and then, reversibly recovered to initial phase via heating or fuming with organic solvent treatment even spontaneously restored. To date, metal-bearing luminogens (Au,19–22 Ag,23 Zn,24 Pd,25 Pt,26 Al27) and organic fluorophores (TPE derivatives,28–33 9,10-divinylanthracene,34–39 cyanostilbene and oligo(p-phenylene vinylene),40–43 ect.) have been reported showing excellent luminescence mechanochromism. The underlying mechanisms of mechanochromism were attributed to the phase transition, the different stacking modes or intramolecular conformation, formation of intermolecular excimers or chemical changes. Further, more fruitful molecular design of MFC materials is suggested by tuning inter- or intramolecular non-covalent weak interactions possibly, such as π–π stacking, hydrogen bonding and hydrophobic interaction, which can be destroyed easily under external stimuli. Even so, high-contrast mechanochromic and multicolour switching materials are also deficiency. The rate or degree of recovery is still difficult to predict after grinding.
As far as we know, most of the reported mechanochromic materials involve the phase transition from crystal state to amorphous state, suffered by the decline of the fluorescence quantum efficiency or even the fluorescence completely quenched owing to increasing rotational or vibrational motions. In this sense, enhancing crystalline-state emissions is beneficial to gain high-contrast mechanochromic materials. Fortunately, many aggregation-induced emission (AIE) active luminogens take propeller-like conformations, possess loose of packing patterns, and exhibit high solid-state fluorescence quantum efficiency by effectively restricting their intramolecular rotations.44,45 These properties make AIE dyes become ideal candidates for high-contrast mechanochromic materials. Exactly, more and more AIE-active molecules were found to be mechanochromic activity. Moreover, functionalisation of traditional dyes is another important way to obtain mechanochromic materials by adopting AIE units. For example, quinacridone and perylenebisimides conjugated with TPE, not suffering from the severe aggregation caused quenching (ACQ) effect, but simultaneously possess AIE property and mechanochromic feature.37,46 It is also known that organoboron complexes form one of the important classes of fluorescent dyes. The synthesis and application of them are a highly relevant topic owing to their unique characteristics. A series of β-diketone boron complexes with MFC behaviors was successively reported by Fraser et al.47–50 More importantly, several AIE-active luminogens including difluoroboron complexes exhibit multicolor switching.51–56
Considering the charming luminescent performance of β-diketonate complexes (DFD), propeller-like conformations of TPE and the unique effect of halogen, herein, three luminogens (2a, 2b and 2c) were designed and synthesized by conjugating TPE with DFD or halogen-substituted DFD. As expected, they all exhibit obviously ICT emission, AIE active and reversible high-contrast mechanochromic properties. Based on effect of halogen, 2b and 2c show polymorphism-dependent fluorescence and increased mechanochromic contrast. Furthermore, the ground powders of 2c could be self-recovered into crystalline state at room temperature. It suggested that 2c will be a good candidate in the anti-faking fields.
Results and discussion
Photophysical properties in solutions
The UV-vis absorption and fluorescence emission spectra of 2a, 2b and 2c in different dilute solution (1 × 10−5 mol L−1) were studied, as shown in Fig. 1, and the corresponding photophysical data were summarized in Table S1.† It was clear show that three complexes emerged two obvious characteristic absorption bands at ca. 360 nm and ca. 420 nm in different polarity solutions, respectively. The weak absorption bands appeared at ca. 360 nm were attributed to π–π* transitions localized on the conjugated aromatic rings, which confirmed by the red-shift absorption with increasing polarity of the solvent. For example, the π–π* transitions bands of 2a emerge at 351 nm in hexane, and red-shifted to 367 nm in DMSO. Owing to electron-donating properties of TPE moiety and electron-accepting properties of difluoroboron β-diketonate moiety, the maximum absorption peak at ca. 420 nm can be coming from intramolecular charge transfer (ICT) transition. With the increasing solvent polarity from 0.13 in n-hexane to 7.20 in DMSO, 2a shows a significant 87 nm red-shifted red emission under 360 nm light irradiation. In comparison, the absorption of 2b and 2c shows an obvious bathochromic shift than 2a due to the electron-withdrawing effect of halogen. However, the emission of 2b and 2c show the abnormal solvation effects in DMSO, which might be due to the small dipole moments of excited molecule greatly increased the excited state energy.57 In addition, it should be noted that both 2c and 2b emerged a shoulder peak at 495 nm and 501 nm in toluene respectively, indicating that two separated close-lying excited states existed. We deduced that the shoulder emission in toluene was come from locally excited state (LE).58–60 Obviously, halogen substituent affects UV-vis absorption and fluorescence emission properties of boron complexes.61
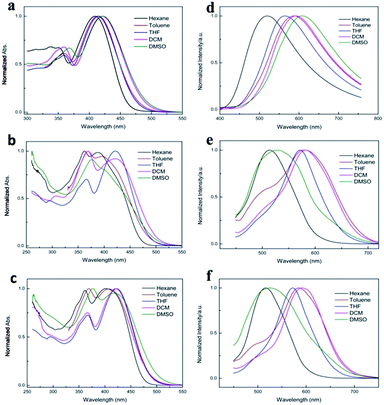 |
| Fig. 1 Normalized UV-vis absorption spectra of 2a (a) and 2b (b), and 2c (c) (1.0 × 10−5 mol L−1), and normalized fluorescent emission spectra of 2a (d) and 2b (e), and 2c (f) excited at 420 nm in different solvents (1.0 × 10−5 mol L−1). | |
AIE properties
As illuminated with a UV light, neither of their THF solutions emitted any observable light, but light was emitted strongly from their solid samples indicating that aggregate formation has an AIE characteristic and turned on their light emission processes. In order to further determine the 2a is AIE in character, the fluorescent behaviours of 2a in a mixed solvent of varying composition were studied. (Note all spectra were measured as soon as possible on mixtures fresh preparation.) In the THF–H2O mixtures, where 2a could easily dissolve in THF, and water was used because it is a non-solvent of 2a: the dye molecules must aggregate in the aqueous mixtures with high water fractions (fw), and simple mixing of solvents could not change the chemical structure of three dyes, which is confirmed by checking the TLC plates. As shown in Fig. 2, extremely weak fluorescence intensity are recorded at fw < 60% because the 2a molecules are fully dissolved in these mixtures, and the fluorescence intensity decreased progressively with increasing fraction of water in the mixtures, due to the increase in the polarity of the medium. While the fluorescence intensity begin to increase for fw > 60%, where the solvating capacity of the mixture is poor to such an extent that the dye molecules starts to aggregate, and the emission wavelength (568 nm) of dye molecules almost no shifted with the increased of water fraction. From the molecular solution in pure THF to the aggregate suspension in 90% aqueous mixture, the fluorescence peak intensity of 2a at 568 nm increases ca. 20-fold. The fluorescence quantum yields (ΦF) of 2a were measured using quinine sulfate in 0.1 M sulfuric acid solution (ΦF = 0.54) as the standard, the ΦF values of 2a in pure THF are as low as 0.12%, similar AIE behaviour was observed for 2c and 2b (Fig. S1 and S2†), and their ΦF values was 0.12% and 0.08% in pure THF, respectively. Clearly, the light emission of all dyes was induced by aggregate formation, that is, all the dyes are AIE active. Moreover, the fluorescent quantum yields of different solid state of three dyes were tested. Surprisingly, the initial crystal of 2a, 2b and 2c gave high ΦF of 99.62%, 99.68% and 89.75%, respectively, which was higher than that in the ground powder of 2a (87.04%), 2b (75.10%) and 2c (67.88%). Notably, the high ΦF of the initial crystal and obtained ground powder were the rare example for the β-diketonate boron complexes.
 |
| Fig. 2 (a) PL spectra of 2a in THF and THF/water mixtures. (b) Plot of PL peak intensity at 568 nm vs. water fraction (fw) of the aqueous mixture. Luminogen concentration: 1 × 10−5 mol L−1; excitation wavelength: 420 nm. Inset: solution of 2a in THF (fw = 0%) and its suspension in a THF/water mixture with fw = 90%; photographs taken under UV illumination. | |
It is come to conclusion that our design strategy of attaching the TPE peripheral groups to the β-diketonate boron complexes has worked well. It has not only totally suppressed the adverse ACQ effect of the target dyes but also conferred novel AIE attributes on the resultant dyes.
Mechanofluorochromic properties
Considering AIE dyes with ICT emission usually gave tuned emission in response to mechanical stimuli, we investigated the mechanochromic properties of 2c, 2b and 2a. As we predicted, three dyes displayed obvious reversible mechanochromic luminescent (ML) behaviour, as depicted in Fig. 3. It could be seen that the emitting colour of three dyes either in the as-synthesized crystal or in ground powders, which were obtained by means of mechanical grinding, were quite different under natural illumination. Meanwhile, the emission switch could be achieved by repeating the treatment of mechanical grinding, fuming with DCM or heating, without displaying fatigue, nearly (Fig. S3–S5†). In order to study such morphology-dependent and molecular structure-dependent solid emission properties, the fluorescent emission spectra of three dyes in different solid states are given in Fig. 4. It was clear that the emission band of as-synthesized crystals of 2a located at 562 nm with yellowish-red light, the emission band red-shifted to 582 nm with orange light and broadened after thoroughly grinding in an agate mortar for 20 min. And it almost recover to initial emitting light after fumed with DCM at room temperature, which might be due to the permeation of the solvent molecules into the ground powders would lead to the rearrangement of molecules, and heated treatment also make it return back to the origin phase. It should be noted that the yellowish-red powder could be gained via recrystallization of ground, fumed or heated samples. In order to well understand the mechanism of such ML phenomenon, the fluorescent lifetimes (τ) and UV-vis solid spectra measured in a reflection way of 2a in different solid states were performed (Fig. S6 and S7†). In the case of the unground crystals of 2a, the analysis of the data by single exponential curves shows that the τ values for the emission at ca. 560 nm was 8.6645 μs with the mean square deviation χ2 (CHISQ) values was 1.001, which was longer than that in ground powder which τ values was 7.0279 μs (χ2 = 1.007). It could be deduced that there was no excimer formed and the π–π interactions turned to weaker in amorphous states due to loose packing pattern in the amorphous solid state.62 Meanwhile, the solid absorption of ground powder of 2a was not red-shifted but broadened compared with those of molecules in unground crystalline state. It was indicated that the ML phenomenon was not affected by planar conformation, but most likely due to strong intermolecular interactions and delocalization of π-electrons among neighbouring molecules in the solid state.63 However, it has a larger difference for 2c. It was clearly shows that the emission band of as-synthesized crystals of 2c located at 547 nm with bright yellow light, after fully grinding, the as-synthesized crystals were turned into ground powders with orange-red light, and the emitting colour drastic red-shifted to 617 nm, which has a more high contrast of colour than 2a, that is, the introduction of halogen endow prominent ML behaviour to the dyes. Additionally, it has a slight different response to the organic solvent, when the ground powders of 2c fumed with DCM at room temperature for ca. 6 min, the emission band blue-shifted to 554 nm, which could not fully recovered to the pristine state, and heating treatment for ground sample also could not fully recovered to the initial state. The different emitting wavelength for the fumed and heated samples might be due to the slight difference in molecular stacking. Far more interesting, the solids with dark yellow emission centred at 565 nm could be formed spontaneously after the ground powders were placed 3 days at room temperature, which is responsible by the partial crystallinity of the ground samples. We also observed similar behaviour in the case of aggregates mixture with 90% water fraction of 2c which the orange light emitting aggregation (named AOr-form) could converted back to the yellow light emitting aggregation (named AY-form), shown in Fig. S13.† Moreover, we also observed that the emission wavelength of AOr-form was between the solid state of as-synthesized and ground powder, it might be due to the AOr-form aggregation was consist of yellow order crystalline and red amorphous powder which can be confirmed by the microscope images of the dry solid come from AOr-form aqueous mixture (Fig. S13†). It was mean that the ground powder was metastable formation and the AOr-form aggregate state in aqueous mixture of 2c exist metastable formation too. The dye of 2b also exhibit large emitting wavelength shift after ground and other similar behaviour to 2c, but the ground powder of 2b could not recovers spontaneously to initial state at room temperature even within one week like 2a, only converted back to the initial state via heated treatment. After analysing the results of these three examples, we deduce that it likely formed slip planes (weak interaction planes) which are generally found in molecular crystals when the weakly interacting functional groups such as t-Bu, -OMe, -SMe, -Cl etc. are organized in such a way that the molecules interact only via dispersive and nonspecific van der Waals (vdW) interactions across a crystallographic plane.63 It should be noted that why 2c showed better reversible ML behaviours, we deemed that the reason was that the size of Br atom bigger than Cl and H which would decrease the stability of amorphous state such as the long carbon chain.62,64 To verify that the spontaneous ML phenomenon was led by atom size, we further synthesized iodine-substituted boron diketonate molecules, denoted as 2d, however, it was not show spontaneous recovery property at room temperature, and the ML behaviour was similar to 2a and 2b (Fig. S14†). So, the spontaneous recovery property of 2c could be due to the inter- and intramolecular interaction make 2c possess better crystallizability. The ML behaviour chloro- and bromo-substituted boron diketonate showed larger emission shifts than those of other materials. The intermolecular halogen bond could influence these large, anomalous emission shifts. So the emission of difluoroboron β-diketonate complexes could be tuned by introduction of halogen, and the spontaneous recovery behaviour of these dyes can also controlled by the same way. Although some dyes exhibit mechanochromic fluorescence, most of them have to treated with solvent or heating to revert back to the initial state, and only a few of them can return to the original emission colour spontaneously.65 The spontaneous recovery of 2c makes it a promising self-healing optical material.
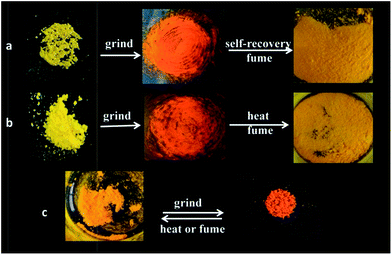 |
| Fig. 3 Photos of 2c (a), 2b (b) and 2a (c) in different solid states under natural light. | |
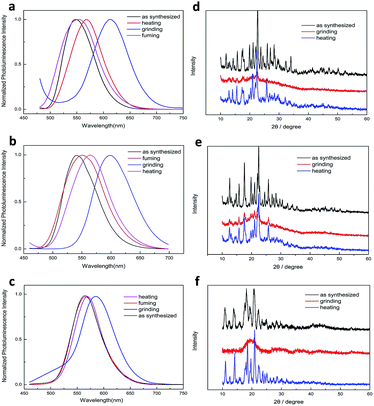 |
| Fig. 4 Fluorescence emission spectra of 2c (a) 2b (b) and 2a (c) in different solid states (λex = 365 nm), and XRD patterns of 2c (d) 2b (e) and 2a (f) in different solid states. | |
It is well known that the molecular packing modes in different solid states would lead to different emission of organic conjugated compounds. Therefore, to verify the MFC phenomenon was induced by the morphology, XRD patterns of three dyes in different solid states are shown in Fig. 4. We found that the as-synthesized crystal gave sharp and intense reflection peaks due to the ordered organization of 2a molecules. In contrast, the XRD profile of the ground solid gives no noticeable diffraction; only present a broad peak, suggesting the disordered molecular packing or amorphous feature in this state. Upon fuming the ground powders with DCM vapour or heating several minutes diffraction peaks emerged again and coincide with the initial unground solid state, indicating that the heating and fuming treatment can convert the amorphous phase to crystalline state through molecular repacking. Thus, the mechanofluorochromic mechanism can be illustrated as a phase transition process: grinding disrupts the ordered crystalline solid into an amorphous state, in which molecular repacking occurred by a single heating treatment. Similarly, 2c and 2b gave similar XRD profile of different solid phase. Differential scanning calorimetry (DSC) analyses could further confirm the different molecular arrangement in different solid states. Therefore, the DSC was performed for the dyes (show in Fig. 5). The unground sample of 2a melts at 266 °C as indicated by the endothermic peak. While the DSC thermograms of the ground powders appeared two weak exothermic transition peaks at 113 °C and 136 °C, which could be attributed to the transformation from amorphous to crystalline, it is revealed that the dye molecular experienced clear cold-crystallization transition, which was absent in the thermograms of crystals sample, and then melted at the similar temperature to that of crystals sample. These results showed the 2a in the amorphous phase was a metastable state, which became a crystalline phase. Therefore investigations on the molecular packing of the dyes are very helpful for further understanding the present MFC behaviour.
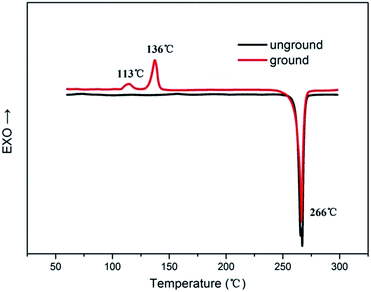 |
| Fig. 5 DSC thermograms of 2b in different state. | |
It is also known that in some cases of organoboron complexes the same fluorophores are present in the different polymorphs and consequently crystal-to-crystal inter-conversion with change in colour emission can take place.29,64 We could not observe the different packing type of the molecular in the aqueous mixture with different water fraction, so we attempt to prepare the crystal of these dyes through the common method of slow evaporation of organic solvent. Fortunately, we successfully prepared the different crystals both of 2b and 2c, but no preferably crystal form for 2a. Crystals of 2c with plate-like shape (named Yc, Fig. 6) can be obtained easily by slow evaporation of a dichloromethane/n-hexane solution, and the XRD spectra (Fig. S11†) of Yc indicated that it was a little different from the as synthesized samples of 2c. Needle-like single crystals Gc (Fig. 6) formed on the inner wall of the bottle via similar way of formation of Yc. The XRD pattern of Gc was not obtained due to a small amount of the samples, but the SEM images of Gc and fluorescence micrograph could confirm it was a crystal (as shown in Fig. 6 and S10†). The fluorescence emission spectra of Yc shows a main band peaked at 558 nm which match approximately with those of suspensions of AY-form, the fluorescence emission spectra of Gc exhibit a main peak at 544 nm which has a 14 nm blue-shift compared with Yc, it was indicated that this type of crystal was another new packing pattern of 2c. The similar polymorphs of 2b were shown in Fig. S12.† According to the reported literature,64 the distinct crystal structure can be transform mutually after heated or annealed treatment, but they could not transformed even the heating temperature exceed the corresponding temperature of cold-crystallization transition peak no matter in the solid state or in the aggregated state of them, mean that all these different type of solid states were stable structures. And these two different crystals can transformed to the same amorphous state after grinding. The DSC analytes of 2b and 2c in different solid states were also tested. As show in Fig. S8 and S9,† the pristine samples of 2b and 2c emerged only a single endothermal peak at ca. 287 °C and 289 °C, respectively, corresponding to their melting points, which was increased compared with 2a probably due to the intermolecular interaction of C–X–H (X = Cl, Br). And the ground sample of 2b and 2c exhibited a single exothermic peak at around 134 °C and 133 °C, respectively, which related to the phase transition of ground powders to the initial state, and then both of them melted at a similar temperature to the unground samples.
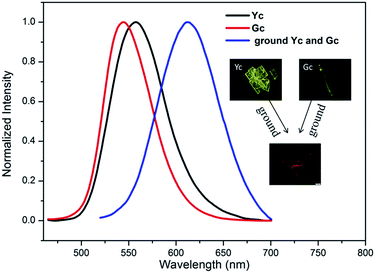 |
| Fig. 6 Fluorescence spectra of two different crystals structure of (Yc) pointed plate-like, (Gc) needle-like structures and ground powder of 2c, inset: fluorescence microscope photos Yc, Gc and ground powder. | |
To sum up, the synergistic effect of phase transition between crystalline state and amorphous state, morphology and the intermolecular interaction of C–X–H (X = Cl, Br) endowed the resulting dye with multicolour emission, and high contrast MCF behaviour with large emission colour/wavelength changes (from green to red and up to 70 nm).
Experimental
Measurement and characterization
1H NMR spectra was obtained with a Varian inova-400 MHz instrument using tetramethylsilane (TMS) as the internal standard. 13C NMR spectra were recorded on a Varian inova-100 MHz spectrometer by using CDCl3 as the solvent in all cases. The UV-vis absorption spectra were obtained on a MaPada UV-3200PCS spectrophotometer. Fluorescent emission spectra were obtained on a Hitachi F-2500 fluorescence spectrophotometer. Fluorescent quantum yields were obtained by FLs980 full-featured steady/transient fluorescence spectrometer (Edinburgh). Glass transition temperature and melting point was determined by DSC measurements carried out using DSC Q2000 (TA, America). ESI/MS spectra were obtained on a Waters GCT Premier. MALDI/HRMS was record on an UltrafleXtreme MALDI-TOF/TOF mass spectrometer (Bruker, Germany). Powder XRD measurements were conducted on D8 Advance (Bruker) with Cu Kα radiation in the range 10° < 2θ < 90°. Digital photographs were taken by Canon 550D (Canon, Japan) digital cameras. Fluorescence lifetimes were obtained on an Edinburgh Instrument FLSP920 fluorescence spectrophotometer, and all the samples were excited at 360 nm. The solid state UV-vis absorption spectra were obtained from Hitachi Limited U-4100. Fluorescence microscopy photos were obtained on OLYMPUS BX53. The scanning electron microscopy (SEM) was obtained on JSM6490LV (Japan, JEOL).
Synthesis
THF and CH2Cl2 were dried according to standardized procedures previously described. All the other chemicals and reagents used in this study were of analytical grade without further purification. In general, all the intermediates and final compounds were purified by column chromatography on silica gel (200–300 mesh), and crystallization from analytical grade solvents. Reactions were monitored by using thin layer chromatography (TLC). The synthetic routes for 2a, 2b and 2c are shown in Scheme 1. Firstly, TPE was synthesized by using benzophenone as the reagent according to the reported procedures; then Friedel–Crafts acylation reaction occurred between TPE and acetyl chloride, to thus give compound Ac-TPE; then, three β-diketonate intermediates and the corresponding complexes were prepared by condensation and boron-complexation reaction, respectively. The target molecules were characterized by 1H NMR, 13C NMR, MALDI-TOF mass spectrometry.
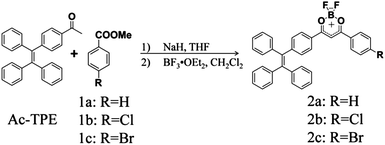 |
| Scheme 1 Synthetic routes of complexes of 2a, 2b and 2c. | |
2,2-Difluoro-4-phenyl-6-(4-(1,2,2-triphenylvinyl)phenyl)-2H-1,3,2-dioxaborinin-1-ium-2-uide (2a)
NaH (60%, 0.13 g, 3.20 mmol) was added quickly dry flask containing a solution of methyl benzoate (0.26, 1.92 mmol) in THF (20 mL) at room temperature. Then, acetyl-TPE (0.60 g, 1.60 mmol) was added. After the mixture was refluxed under an atmosphere of argon for 24 h, it was cooled to room temperature. After that, the mixture was acidified with dilute HCl. The mixture was poured into water and extracted with dichloromethane three times. Then the organic phase was combined and dried over anhydrous Na2SO4. Removing the solvent under reduced pressure and a yellow residue solid was collected. Then, the solid was dried under vacuum followed by dissolving in CH2Cl2 (40 mL). The boron trifluoride diethyl ether complex (0.4 mL, 3.20 mmol) was added to the above solution, which was stirred under an atmosphere of argon at room temperature for 24 h. Water was added in order to quench the reaction. The organic layer was separated and dried over Na2SO4. After removal of the solvent, the crude product was purified by column chromatography (silica gel, petroleum ether/CH2Cl2, v/v = 1/1) to afford 2a (0.34 g) as a yellow solid. Yield 40%; mp 265.0–266.0 °C. 1H NMR (400 MHz, CDCl3) δ/ppm = 8.15 (s, 1H), 8.13 (s, 1H), 7.93 (s, 1H), 7.71 (t, J = 8.0, 1H), 7.57 (t, J = 8.0, 2H), 7.24 (s, 1H), 7.22 (s, 1H), 7.18–7.12 (m, 10H), 7.07 (m, 6H) (Fig. S15†); 13C NMR (100 MHz, CDCl3): δ/ppm = 182.58, 151.92, 143.85, 142.88, 142.65, 139.44, 135.06, 132.15, 131.33, 131.32, 131.24, 129.15, 128.81, 128.47, 128.07, 128.03, 127.77, 127.33, 127.07, 127.04 (Fig. S19†); HRMS (MALDI-TOF): m/z 507.1939 [[M − F]+, calculated 507.1932] (Fig. S23†).
4-(4-Chlorophenyl)-2,2-difluoro-6-(4-(1,2,2-triphenylvinyl)phenyl)-2H-1,3,2-dioxaborinin-1-ium-2-uide (2b)
Compound 2b was prepared by following the synthetic procedure for compound 2a. The crude product was purified by column chromatography (silica gel, CH2Cl2–petroleum ether, v/v = 7/4), to afford 2b (0.31 g) as a yellow solid. Yield: 35%. mp 286.0–287.0 °C. 1H NMR (400 MHz, CDCl3) δ/ppm = 8.08 (d, J = 12.0, 2H), 7.92 (d, J = 12.0, 2H), 7.55 (d, J = 8.0, 2H), 7.23 (d, J = 8.0, 2H), 7.18–7.13 (m, 9H), 7.07–7.02 (m, 7H) (Fig. S16†); 13C NMR (100 MHz, CDCl3): δ/ppm = 152.23, 143.99, 142.83, 142.60, 141.74, 139.39, 132.20, 131.32, 131.23, 130.55, 130.01, 129.58, 129.39, 128.56, 128.05, 127.78, 127.36, 127.11, 127.07, 93.22 (Fig. S20†); HRMS (MALDI-TOF): m/z 541.1539 [[M − F]+, calculated 541.1542 ] (Fig. S24†).
4-(4-Bromophenyl)-2,2-difluoro-6-(4-(1,2,2-triphenylvinyl)phenyl)-2H-1,3,2-dioxaborinin-1-ium-2-uide (2c)
Compound 2c was prepared by following the synthetic procedure for compound 2a. The crude product was purified by column chromatography (silica gel, CH2Cl2–petroleum ether, v/v = 7/4), to afford 2c (0.37 g) as a yellow solid. Yield: 38%. mp 287.0–289.0 °C. 1H NMR (400 MHz, CDCl3) δ/ppm = 7.99 (d, J = 8.0, 2H), 7.92 (d, J = 12.0, 2H), 7.71 (d, J = 8.0, 2H), 7.23 (d, J = 8.0, 2H), 7.18–7.14 (m, 9H), 7.07–7.03 (m, 7H) (Fig. S17†); 13C NMR (100 MHz, CDCl3): δ/ppm = 152.27, 142.83, 142.60, 139.39, 132.57, 132.21, 131.32, 131.23, 130.54, 130.01, 128.58, 128.08, 128.05, 127.78, 127.36, 127.07, 99.99 (Fig. S21†); HRMS (MALDI-TOF): m/z 585.1034 [[M − F]+, calculated 585.1037] (Fig. S25†).
4-(4-Iodophenyl)-2,2-difluoro-6-(4-(1,2,2-triphenylvinyl)phenyl)-2H-1,3,2-dioxaborinin-1-ium-2-uide (2d)
Compound 2d was prepared by following the synthetic procedure for compound 2a. The crude product was purified by column chromatography (silica gel, CH2Cl2–petroleum ether, v/v = 7/4), to afford 2d (0.37 g) as a yellow solid. Yield: 37%. mp 279.0–280.0 °C. 1H NMR (400 MHz, CDCl3) δ/ppm = 7.92 (t, J = 8.0, 4H), 7.82 (d, J = 8.0, 2H), 7.23 (d, J = 8.0, 2H), 7.18–7.14 (m, 9H), 7.07–7.02 (m, 7H) (Fig. S18†); 13C NMR (100 MHz, CDCl3): δ/ppm = 183.02, 181.40, 152.26, 144.00, 142.84, 142.82, 142.60, 139.41, 138.56, 132.21, 131.54, 131.32, 131.24, 129.77, 129.38, 128.60, 128.09, 128.06, 127.79, 127.37, 127.11, 127.08, 103.56, 93.20 (Fig. S22†); HRMS (MALDI-TOF): m/z 633.0898 [[M − F]+, calculated 652.0882] (Fig. S26†).
Conclusions
In summary, three difluoroboron β-diketonate complexes containing TPE unit have been synthesized via McMurry coupling, Friedel–Crafts acylation, Claisen condensation and boron-complex reactions. According to a series of test results, including UV-vis absorption spectra, fluorescence emission spectra, AIE and mechanochromic properties, our data indicated that TPE unit endows luminophores AIE activity, the specific structure also endow them extremely high fluorescent quantum yields which was rare reported. Moreover, they exhibit remarkable and reversible mechanochromic properties, while halogen can further improve mechanochromic contrast, affect mechanochromic reversibility. Especially, chlorine-substituted complexes exhibited the remarkable bathochromic shift (70 nm) in the maximum fluorescence emission wavelength before/after grinding, while bromo-substituted complexes can be recovered spontaneously to crystal-state from amorphous state at room temperature. XRD, DSC, solid-state UV-vis absorption and fluorescent lifetime studies suggest that the reversible mechanochromism is due to the change of crystalline to amorphous phase and metastable state formation, rather than molecular planarization, excimer or exciplex formation. In addition, yellow plate-like and green needle-like crystal as well discrepancies of fluorescence emission among pristine crystal and recovery crystal by heating or solvent fumigation show that halogenated complexes have polymorphism, and might be potential multicolour switching.
Acknowledgements
This work was financially supported by the National Natural Science Foundation of China (No. 21566034), National University students' innovative pilot projects (201510759022), the Key Programs for Science and Technology Development of Shihezi University (GXJS2013-ZDGG02, 2014zrkxyq05).
References
- Y. Dong, J. W. Y. Lam, A. Qin, J. Liu, Z. Li, B. Z. Tang, J. Sun and H. S. Kwok, Appl. Phys. Lett., 2007, 91, 011111 CrossRef.
- A. Pucci, F. Di Cuia, F. Signori and G. Ruggeri, J. Mater. Chem., 2007, 17, 783–790 RSC.
- Z. Ning, Z. Chen, Q. Zhang, Y. Yan, S. Qian, Y. Cao and H. Tian, Adv. Funct. Mater., 2007, 17, 3799–3807 CrossRef CAS.
- B. R. Crenshaw, M. Burnworth, D. Khariwala, A. Hiltner, P. T. Mather, R. Simha and C. Weder, Macromolecules, 2007, 40, 2400–2408 CrossRef CAS.
- S. J. Toal, K. A. Jones, D. Magde and W. C. Trogler, J. Am. Chem. Soc., 2005, 127, 11661–11665 CrossRef CAS PubMed.
- M. Kinami, B. R. Crenshaw and C. Weder, Chem. Mater., 2006, 18, 946–955 CrossRef CAS.
- S. Hirata and T. Watanabe, Adv. Mater., 2006, 18, 2725–2729 CrossRef CAS.
- S.-J. Lim, B.-K. An, S. D. Jung, M.-A. Chung and S. Y. Park, Angew. Chem., Int. Ed., 2004, 43, 6346–6350 CrossRef CAS PubMed.
- C. E. Olson, M. J. R. Previte and J. T. Fourkas, Nat. Mater., 2002, 1, 225–228 CrossRef CAS PubMed.
- M. Irie, T. Fukaminato, T. Sasaki, N. Tamai and T. Kawai, Nature, 2002, 420, 759–760 CrossRef CAS PubMed.
- A. Kishimura, T. Yamashita, K. Yamaguchi and T. Aida, Nat. Mater., 2005, 4, 546–549 CrossRef CAS PubMed.
- S. Hirata and T. Watanabe, Adv. Mater., 2006, 18, 2725–2729 CrossRef CAS.
- L. Seon-Jeong, A. Byeong-Kwan, J. S. Don, C. Myung-Ae and P. S. Young, Angew. Chem., Int. Ed., 2004, 43, 6346–6350 CrossRef PubMed.
- M. Irie, T. Fukaminato, T. Sasaki, N. Tamai and T. Kawai, Nature, 2002, 420, 759–760 CrossRef CAS PubMed.
- M. Kinami, B. R. Crenshaw and C. Weder, Chem. Mater., 2006, 18, 946–955 CrossRef CAS.
- Y. Gong, Y. Zhang, W. Z. Yuan, J. Z. Sun and Y. Zhang, J. Phys. Chem. C, 2014, 118, 10998–11005 CAS.
- G. Yongyang, T. Yeqiang, L. Jun, L. Ping, F. Cunfang, Y. Wang Zhang, L. Yawei, S. Jing Zhi, H. Gufeng and Z. Yongming, Chem. Commun., 2013, 49, 4009–4011 RSC.
- A. Patra, M. Pan, C. S. Friend, T. C. Lin, A. N. Cartwright, P. N. Prasad and R. Burzynski, Chem. Mater., 2002, 14, 4044–4048 CrossRef CAS.
- Y.-A. Lee and R. Eisenberg, J. Am. Chem. Soc., 2003, 125, 7778–7779 CrossRef CAS PubMed.
- H. Ito, T. Saito, N. Oshima, N. Kitamura, S. Ishizaka, Y. Hinatsu, M. Wakeshima, M. Kato, K. Tsuge and M. Sawamura, J. Am. Chem. Soc., 2008, 130, 10044–10045 CrossRef CAS PubMed.
- Z. Assefa, M. A. Omary, B. G. McBurnett, A. A. Mohamed, H. H. Patterson, R. J. Staples and J. P. Fackler Jr, Inorg. Chem., 2002, 41, 6274–6280 CrossRef CAS PubMed.
- J. Schneider, Y.-A. Lee, J. Perez, W. W. Brennessel, C. Flaschenriem and R. Eisenberg, Inorg. Chem., 2008, 47, 957–968 CrossRef CAS PubMed.
- T. Tsukuda, M. Kawase, A. Dairiki, K. Matsumoto and T. Tsubomura, Chem. Commun., 2010, 46, 1905–1907 RSC.
- S. Mizukami, H. Houjou, K. Sugaya, E. Koyama, H. Tokuhisa, T. Sasaki and M. Kanesato, Chem. Mater., 2005, 17, 50–56 CrossRef CAS.
- J. K. Grey, I. S. Butler and C. Reber, Inorg. Chem., 2003, 42, 6503–6518 CrossRef CAS PubMed.
- T. Abe, T. Itakura, N. Ikeda and K. Shinozaki, Dalton Trans., 2009, 711–715 RSC.
- H. Bi, D. Chen, D. Li, Y. Yuan, D. Xia, Z. Zhang, H. Zhang and Y. Wang, Chem. Commun., 2011, 47, 4135–4137 RSC.
- S. P. Anthony and S. M. Draper, J. Phys. Chem. C, 2010, 114, 11708–11716 CAS.
- Y. Zhao, H. Gao, Y. Fan, T. Zhou, Z. Su, Y. Liu and Y. Wang, Adv. Mater., 2009, 21, 3165–3169 CrossRef CAS.
- A. Yamamoto, M. Matsumoto, T. Hinoue, Y. Mizobe, I. Hisaki, M. Miyata and N. Tohnai, Synth. Met., 2009, 159, 905–909 CrossRef CAS.
- N. S. S. Kumar, S. Varghese, N. P. Rath and S. Das, J. Phys. Chem. C, 2008, 112, 8429–8437 CAS.
- T. Mutai, H. Satou and K. Araki, Nat. Mater., 2005, 4, 685–687 CrossRef CAS PubMed.
- R. Davis, N. P. Rath and S. Das, Chem. Commun., 2004, 74–75 RSC.
- C. Ma, B. Xu, G. Xie, J. He, X. Zhou, B. Peng, L. Jiang, B. Xu, W. Tian, Z. Chi, S. Liu, Y. Zhang and J. Xu, Chem. Commun., 2014, 50, 7374–7377 RSC.
- H. Li, Z. Chi, B. Xu, X. Zhang, X. Li, S. Liu, Y. Zhang and J. Xu, J. Mater. Chem., 2011, 21, 3760–3767 RSC.
- Q. Qi, J. Zhang, B. Xu, B. Li, S. X.-A. Zhang and W. Tian, J. Phys. Chem. C, 2013, 117, 24997–25003 CAS.
- M. P. Aldred, G.-F. Zhang, C. Li, G. Chen, T. Chen and M.-Q. Zhu, J. Mater. Chem. C, 2013, 1, 6709–6718 RSC.
- Y. W. Lu, Y. Q. Tan, Y. Y. Gong, H. Li, W. Z. Yuan, Y. M. Zhang and B. Z. Tang, Chin. Sci. Bull., 2013, 58, 2719–2722 CrossRef CAS.
- W. Z. Yuan, Y. Tan, Y. Gong, P. Lu, J. W. Y. Lam, X. Y. Shen, C. Feng, H. H. Y. Sung, Y. Lu, I. D. Williams, J. Z. Sun, Y. Zhang and B. Z. Tang, Adv. Mater., 2013, 25, 2837–2843 CrossRef CAS PubMed.
- J. Kunzelman, M. Kinami, B. R. Crenshaw, J. D. Protasiewicz and C. Weder, Adv. Mater., 2008, 20, 119–122 CrossRef CAS.
- S.-J. Yoon, J. W. Chung, J. Gierschner, K. S. Kim, M.-G. Choi, D. Kim and S. Y. Park, J. Am. Chem. Soc., 2010, 132, 13675–13683 CrossRef CAS PubMed.
- S.-J. Yoon and S. Park, J. Mater. Chem., 2011, 21, 8338–8346 RSC.
- J. Sun, X. Lv, P. Wang, Y. Zhang, Y. Dai, Q. Wu, M. Ouyang and C. Zhang, J. Mater. Chem. C, 2014, 2, 5365–5371 RSC.
- L. Z. Xie, J. W. Y. Lam, L. Cheng, H. Chen, C. Qiu, H. S. Kwok, X. Zhan, Y. Liu, D. Zhu and B. Z. Tang, Chem. Commun., 2001, 1740–1741 Search PubMed.
- J. Chen, C. C. W. Law, J. W. Y. Lam, Y. Dong, S. M. F. Lo, I. D. Williams, D. Zhu and B. Z. Tang, Chem. Mater., 2003, 15, 1535–1546 CrossRef CAS.
- I. Javed, T. Zhou, F. Muhammad, J. Guo, H. Zhang and Y. Wang, Langmuir, 2012, 28, 1439–1446 CrossRef CAS PubMed.
- G. Zhang, J. P. Singer, S. E. Kooi, R. E. Evans, E. L. Thomas and C. L. Fraser, J. Mater. Chem., 2011, 21, 8295–8299 RSC.
- N. D. Nguyen, G. Zhang, J. Lu, A. E. Sherman and C. L. Fraser, J. Mater. Chem., 2011, 21, 8409–8415 RSC.
- Z. Guoqing, L. Jiwei and C. L. Fraser, Inorg. Chem., 2010, 49, 10747–10749 CrossRef PubMed.
- J. D. A. Espinoza, V. Sazhnikov, E. C. P. Smits, D. Ionov, Y. Kononevich, I. Yakimets, M. Alfimov and H. F. M. Schoo, J. Fluoresc., 2014, 24, 1735–1744 CrossRef PubMed.
- R. Yoshii, A. Nagai, K. Tanaka and Y. Chujo, Chem.–Eur. J., 2013, 19, 4506–4512 CrossRef CAS PubMed.
- G. Petra, R. C. KorošEc, V. Maja and K. Boris, J. Am. Chem. Soc., 2014, 136, 7383–7394 CrossRef PubMed.
- L. Chia-Wei, R. M. Rajeswara and S. Shih-Sheng, Chem. Commun., 2015, 51, 2656–2659 RSC.
- F. P. Macedo, C. Gwengo, S. V. Lindeman, M. D. Smith and J. R. Gardinier, Eur. J. Inorg. Chem., 2008, 2008, 3200–3211 CrossRef.
- D. R. Yoshii, K. Suenaga, D. K. Tanaka and P. Y. Chujo, Chem.–Eur. J., 2015, 21, 7231–7237 CrossRef PubMed.
- K. Yasuhiro, T. Syunki, F. Kazumasa and M. Masaki, Org. Lett., 2012, 14, 4682–4685 CrossRef PubMed.
- Y. Gong, Y. Tan, J. Liu, P. Lu, C. Feng, W. Z. Yuan, Y. Lu, J. Z. Sun, G. He and Y. Zhang, Chem. Commun., 2013, 49, 4009–4011 RSC.
- S. Nad and H. Pal, J. Phys. Chem. A, 2001, 105, 1097–1106 CrossRef CAS.
- G. Jones II, W. R. Jackson, C. Y. Choi and W. R. Bergmark, J. Phys. Chem., 1985, 89, 294–300 CrossRef.
- F. Loiseau, S. Campagna, A. Hameurlaine and W. Dehaen, J. Am. Chem. Soc., 2005, 127, 11352–11363 CrossRef CAS PubMed.
- J. Sun, X. Lv, P. Wang, Y. Zhang, Y. Dai, Q. Wu, M. Ouyang and C. Zhang, J. Mater. Chem. C, 2014, 2, 5365 RSC.
- Z. Zhang, Z. Wu, J. Sun, B. Yao, G. Zhang, P. Xue and R. Lu, J. Mater. Chem. C, 2015, 3, 4921–4932 RSC.
- G. R. Krishna, R. Devarapalli, R. Prusty, T. Liu, C. L. Fraser, U. Ramamurty and C. M. Reddy, IUCrJ, 2015, 2, 611–619 CAS.
- X. Luo, J. Li, C. Li, L. Heng, Y. Q. Dong, Z. Liu, Z. Bo and B. Z. Tang, Adv. Mater., 2011, 23, 3261–3265 CrossRef CAS PubMed.
- G. Zhang, J. Lu, M. Sabat and C. L. Fraser, J. Am. Chem. Soc., 2010, 132, 2160–2162 CrossRef CAS PubMed.
Footnote |
† Electronic supplementary information (ESI) available: 1H NMR, 13C NMR, MALDI-TOF MS spectra; photophysical data; UV-vis absorption spectra, scanning electron microscopy, XRD spectra, fluorescence lifetimes. See DOI: 10.1039/c5ra27819h |
|
This journal is © The Royal Society of Chemistry 2016 |