DOI:
10.1039/C5RA27332C
(Paper)
RSC Adv., 2016,
6, 34354-34363
Biological tooth root reconstruction with a scaffold of swine treated dentin matrix†
Received
21st December 2015
, Accepted 22nd March 2016
First published on 24th March 2016
Abstract
Treated dentin matrix (TDM) is an ideal scaffolding material with odontogenic ability, which is important for supporting cell growth and regeneration of dental tissue. The source of TDM could be a key point for clinical application in the future. Xenogenic TDM from swine (sTDM) may be an ideal alternative because of its high similarities with human anatomy and structure. However, due to species-specific differences, it remains unclear whether TDM fabrication methods can be extended to sTDM. In the present study, we have optimized the fabrication method to fabricate a sTDM scaffold material with odontogenic ability. Furthermore, dental follicle cells from swine (sDFCs) were combined with sTDM and were implanted into the alveolar fossa of swine to reconstruct the biological tooth root in vivo. The results showed the step-by-step process of demineralization by EDTA was effective and necessary. The treatment times with 17% EDTA, 10% EDTA and 5% EDTA should be at least 15 min, 15 min, and 5 min, respectively. Under the induction of sTDM, the growth and viability of seeding cells were significantly improved. Most importantly, sTDM in vivo could induce and support the regeneration of complete tooth root tissues. The cells within and surrounding the regenerated tissues were positive for green fluorescent protein (GFP), indicating that sDFSCs are responsible for the regenerated tooth root tissues. In conclusion, sTDM, which was fabricated by an optimized method, could be suitable for reconstruction of tooth roots in vivo in swine.
1. Introduction
Repairing and replacing damaged and lost tooth tissues is of clinical relevance and importance as many people suffer damaged and lost tooth tissues, which adversely affects their mouth's functions and food-eating ability. Tooth regeneration has long been the dental profession's aspiration. However, it was not until the recently that advances in cell biology and bioengineering made experimental approaches for tooth regeneration possible.1 Stem cell-mediated tissue engineering could offer a prospective strategy for repairing and replacing damaged and lost tooth tissues.2,3 Tooth regeneration encompasses not only regeneration of an entire tooth as an organ, but also biological restoration of individual components of the tooth tissues, including enamel, dentin, dental pulp, or cementum.4 However, due to the complexity of human tooth growth and development, more research was needed to regenerate the whole tooth structure, including enamel, dentin/pulp complex, and periodontal tissues as a functional entity. A tooth root that can support a natural or an artificial crown is the most important part of the tooth for maintaining tooth functions.5,6 Recently, a number of previous studies have reported successful regeneration of tooth roots in rats with a structure similar to natural roots, including periodontal ligaments (PL), cementum-like tissues, and pulp–dentin complexes.2,7–10
Suitable scaffolding materials play important role in tooth tissue engineering and are capable of regenerating tooth structures such as dentin and periodontal tissues.3,11 To date, scaffolding materials commonly used include ceramic bovine bone (CBB), the copolymer of poly-DL-lactide and glycolide (PLG), hydroxyapatite/tricalcium phosphate (HA/TCP), polyglycolic acid (PLGA) and polysaccharide.4 These materials typically only provide structural support for cells and support biomineralization; none provide an environment conducive to cell differentiation relevant to tooth root regeneration. To provide an odontogenic inductive microenvironment, a number of bioactive factors have been added into these scaffolding biomaterials.12 Recently, dentin matrix was found to contain a number of bioactive molecules, factors, and proteins related to dentin and pulp tissue formation.13 According to our previous study, by a step-by-step protocol with 17%, 10% and 5% ethylenediaminetetraacetic acid (EDTA) and ultrasonic cleaning, a number of important proteins that play significant roles during tooth development could be released from dentin matrix.3 EDTA could loosen dentin structure and effectively remove the smear layer of organic and inorganic debris created during root canal therapy.14,15 The combined use of EDTA and ultrasonic cleaning has been shown to be particularly effective for smear layer and debris removal during the fabrication of human dentin matrix.16 The protocol of dentin matrix fabrication in terms of EDTA concentrations and treatment time has been optimized and its effect was previously explored in smear layer removal and dentin tubule exposure.3,8 Our fabrication methods for dentin matrix compared to those for other scaffolding materials may be satisfactory because excessive demineralization can destroy the structure of dentin and also affect the containment and functionality of odontogenic factors, whereas insufficient demineralization generates a less odontogenic scaffold. Our fabrication method could protect these odontogenic factors as much as possible, which could benefit the regeneration of tooth root.
Furthermore, dentin matrix is also a scaffold suitable for the prefabrication of the shapes relevant to tissue regeneration.3,8 TDM may be a suitable scaffold and serve as an inductive microenvironment for the reconstruction of tooth tissue. Thus, the source of TDM could be an essential aspect for clinical application in the future. Xenogenic TDM may be an ideal alternative for tooth root reconstruction due to the reason that autologous or allogeneic TDM could not be impractical.
Swine share high similarities with humans in anatomy and structure, so swine have been extensively used as a large animal model in many biomedical experiments,17,18 especially in dental and orofacial research.19 Swine have both deciduous and permanent dentitions. The initiation of tooth development and eruption in swine are quite similar to those in humans.19 Thus, swine could be used as an excellent translational model for testing the concept of regeneration of functional tooth roots and the feasibility of using autologous stem cells for tooth transplantation. Thus, successful reconstruction of tooth roots in swine may be of importance and have clinical relevance. Recently, a bio-root has been successfully regenerated in swine in situ using swine dentin matrix and dental follicle stem cells (sDFSCs).20 This indicates that sTDM might also be an ideal biological scaffold for tooth root reconstruction in swine. However, studies about TDM scaffold from swine (sTDM) are rare. Whether the method of fabrication of human TDM is suitable for sTDM is not clear. Considering the similarity of human and swine TDM, xenogenic TDM derived from swine may be a good option for further clinical application in human. Therefore, the protocol of sTDM fabrication should be highly optimized and unified to obtain the best results for clinical application.
Therefore, in this study, we aimed to develop a fabrication method based on previous studies for sTDM with proper odontogenic ability2,3 and studied the odontogenic characteristics of sTDM. This study also assessed whether sTDM can act as a suitable scaffold for tooth root reconstruction in swine.
2. Materials and methods
2.1. Animals
Four swine (12 month old) were obtained from the Laboratory Animal Research Centre of Zhengzhou University and were used to obtain TDM from their upper incisors and to isolate dental follicle cells (sDFSCs). All animal experiments were approved by the Animal Care and Use Committee and conducted in accordance with the committee guidelines of the Zhengzhou University for animal experiments, which also met the NIH guidelines for the care and use of laboratory animals.
2.2. Fabrication and detection of treated dentin matrix (sTDM) of swine
2.2.1 Fabrication of sTDM. The purpose of sTDM fabrication is to sufficiently expose dentinal tubules and loosen fiber bundles of intertubular and peritubular dentin, which could release dentinogenesis-related proteins. According to our previous study, a step-by-step demineralization with ethylenediaminetetraacetic acid (EDTA, Sigma, US) and ultrasonic cleaning could effectively remove the smear layer and inorganic debris of hTDM.3The upper incisors from swine were extracted under proper anesthesia. The outer cementum and part of the dentin was removed by grinding along the tooth profile. Most of the dental pulp tissues and pre-dentin were mechanically removed. The same parts of multiple incisors were obtained and analyzed. As excessive demineralization by EDTA can destroy the structure of dentin and also affect the containment and functionality of odontogenic factors, insufficient demineralization generates a less odontogenic scaffold. Based on previous studies on TDM treatment,3,21,22 we added two groups with different treatment times and tried to optimize the fabrication method (Table 1). Each groups included five treated dentin matrices.
Table 1 Different groups with different treatment times
|
17% EDTA |
10% EDTA |
5% EDTA |
Group 1 (n = 5) |
5 min |
5 min |
10 min |
Group 2 (n = 5) |
10 min |
10 min |
5 min |
Group 3 (n = 5) |
15 min |
15 min |
5 min |
Group 4 (n = 5) |
15 min |
15 min |
10 min |
Group 5 (n = 5) |
20 min |
20 min |
10 min |
In addition to the EDTA treatment times, other steps were similar. In brief, dentin matrix was soaked in deionized water for 5 h and mechanically cleaned for 20 min every hour using an ultrasonic cleaner before demineralization. The deionized water was changed every hour. After each demineralization step, the dentin matrix was washed in deionized water for 20 min in an ultrasonic cleaner. Finally, treated dentin matrices from swine were placed in sterile PBS (Hyclone, US) with 100 units per mL penicillin (Hyclone, US) and 100 mg mL−1 streptomycin (Hyclone, US) for 72 h, washed with sterile deionized water for 20 min in an ultrasonic cleaner, and stored in DMEM (Hyclone, US) at 4 °C. Using this treatment, miniature treated dentin matrix was obtained, with a similar shape to the upper incisor and 2 mm in thickness.
Finally, each group was visualized using a scanning electron microscope (SEM) (Inspect F, FEI, Netherlands). In brief, sTDM was washed in PBS three times, fixed with 2.5% glutaraldehyde at 0 °C for 30 min, dehydrated and dried in a critical-point dryer, and finally visualized under SEM.
2.2.2 Effects of sTDM-mediated induction on cell viability. We made sTDM extraction fluid as described in our previous study.3 To verify the induction of sTDM, we used dental follicle stem cells (sDFSCs) induced in sTDM extraction fluid as experimental group. The sDFSCs cultured under normal DMEM were used as the control group.A cell count kit-8 (CCK-8, Dojindo, Japan) was used to quantitatively evaluate the viability of sDFSCs according to the manufacturer's instructions. After sDFSCs were induced in sTDM extraction fluid for 1, 2, 3, 4, 5, 6 and 7 days, the original culture medium was replaced by 500 mL of DMEM with 10% FBS containing 50 mL of CCK-8. After being incubated at 37 °C for 3 h, 100 mL of cultured medium was taken from each sample mentioned above and added to one well of a 96 well plate. Six parallel replicates were prepared. The absorbance at 450 nm was determined using a spectrophotometer (Thermo VARIOSKAN FLASH, Thermo, USA).
2.3. Isolation, culture, transduction and characteristics of dental follicle stem cells from swine (sDFSCs)
2.3.1 Isolation and culture of sDFSCs. SDFSCs were isolated from the dental follicles of un-erupted molars from swine and cultured using the protocols described previously23 (details in ESI†).
2.3.2 Transduction of sDFSCs with green fluorescent protein (GFP). For monitoring the in vivo implantation of the sDFSCs, we transduced the GFP gene as a labeling marker into sDFSCs following the manufacturer's instruction. In brief, a total of 1 × 105 sDFSCs were seeded into each well of a six-well plate. At 80% confluence, cells in each well were incubated with 2.3 × 107 eGFP lentivirus (Neuron Biotech, China) and 1 μL polybrene (Neuron Biotech, China) in 2 mL FBS-free α-MEM media for 8 hours. Then, the media containing lentivirus was replaced by complete α-MEM containing 10% FBS. 48 hours later, the effect of eGFP transduction was examined under a fluorescence microscope (Leica Optical, Germany).
2.3.3 Transmission electron microscopy (TEM) of sDFSCs. SDFSCs were seeded onto each well of a six-well plate at a concentration of 2 × 104 cells per cm2. After being cultured with stem cell growth medium for 7 days, the cells were trypsinized and centrifuged at 3000 g, 4 °C for 5 min to form pellets. The cells were then fixed in 2% glutaraldehyde in 0.1 M cacodylate buffer (pH 7.3) for 1 h at room temperature and postfixed in aq. 2% (v/v) osmium tetroxide for an additional 1 h. The fixed cells were then consecutively dehydrated with an ethanol gradient series (50, 70, 95 and 100%) and embedded in Epon 812 resin. Ultrathin sections were made and stained with uranyl acetate and lead citrate. The stained samples were viewed with a JEM 100 SX electron microscope (Jeol, Tokyo, Japan).
2.3.4 Characteristics of stem cells in sDFSCs.
2.3.4.1 Immunofluorescent cell staining. One day prior to being stained, sDFSCs were released and seeded onto 0.8 cm × 0.8 cm coverslips in a 24 well plate for further culture. SDFSCs were fixed with 4% polyoxymethylene for 30 min. Subsequent steps were performed according to the manufacturer's recommendations. Antibodies used for immunofluorescent staining included those for vimentin and CK-14. Vimentin antibody was obtained from Santa Cruz Biotech Inc. (Santa Cruz, USA). The antibody for CK-14 was obtained from Abeam, USA. All the immunostained samples were examined under a fluorescence microscope (Leica Optical, Germany).
2.3.4.2 Multi differentiation potential of sDFSCs. Osteogenic conditions are described in the ESI.† After being cultured for 15 days, 0.1% alizarin red solution (Sigma, USA) was employed to analyze the osteogenic differentiation under a light microscope (Nikon, Japan).Primary adipogenic conditions were prepared as described previously.24 After four weeks, the cells growing under adipogenic conditions were analyzed by Oil Red O (Sigma, USA). Staining was performed as described previously (details in ESI†).
At 80% confluence, sDFSCs were cultured in neurogenic medium (details in ESI†). After 4 h, cells were analyzed by immunocytofluorescence for expression of the neural cell marker, βIII-tubulin (Abcam, USA). Cells were fixed and analyzed under a fluorescence microscope (Leica Optical, Germany).
2.3.4.3 Characterization of surface molecules. Approximately 5 × 105 sDFSCs were incubated with anti-CD146 (1
:
100), CD31 (1
:
100), CD90 (1
:
100), CD29 (1
:
100), and CD45 (1
:
100; BD) (all from Becton Dickinson (BD), USA), and CD44 (1
:
100) (R&D Systems, Inc., USA), according to the manufacturer's instructions. FITC-conjugated and isotype-matched immunoglobulins were used to determine non-specific staining. The secondary reagents included IgG-FITC (Santa Cruz, USA). Cells were analyzed on a FACS Calibur (BD, USA) and the data were analyzed using CellQuest software (BD, USA).
2.4. Creation of alveolar fossa microenvironment
Fresh alveolar fossa was used as an inductive microenvironment for the regeneration of the periodontal tissues, such as cementum–periodontal ligament complex. The 12 month old swine were anesthetized and their oral cavities were disinfected. A gingival separator was used to isolate the soft tissues surrounding the mandibular premolars. Using surgical sutures, the soft tissues were fixed near the buccal mucosa and tongue tissue. Furthermore, the alveolar ridges were removed using spherical drills. The mandibular premolars were removed to expose the alveolar fossa. Finally, the septum interalveolare in the alveolar fossa was removed.
2.5. Generation of biological tooth roots in vivo
Reconstruction of tooth roots has been studied previously, among which tissue engineering has shown its superiority.2,6 The stem cell and scaffold-based bio-root could be regenerated by integrating several approaches together, including stem cell techniques, biomaterials and bioactive factors. In general, a root-shaped implantation site was generated and the combination of scaffold and stem cells was implanted for a period of time to be re-integrated into the jaw and perform the normal functions of a natural tooth, including regenerative ability in case of injury.6 We have optimized the shape and size of treated dentin matrix scaffolds used for tooth root regeneration according to a previous study.22 An optimized scaffold seeded with dental stem cells was transplanted to regenerate tooth root in swine in vivo. In brief, sDFSCs were seeded onto sTDMs at a density of 1 × 104 cells per scaffold. The sTDMs with sDFSCs were transferred to a culture dish and incubated at 37 °C, 5% CO2 for 72 h before implanted in the pre-constructed alveolar fossa. The operation was performed under general anesthesia. Eight weeks later, all samples were harvested from the swine and 3-D images of grafts were obtained using micro-CT (Enhanced Vision Systems Model MS-8 In Vitro Micro-CT Scanner; GE Healthcare, Canada) to estimate the effect of periodontal tissue formation and remodeling around scaffolds.
All implants were harvested, fixed with 4% paraformaldehyde overnight at 4 °C, demineralized using 10% EDTA (pH 8.0) and embedded in paraffin. Paraffin sections (5 μm) were stained with hematoxylin and eosin (H & E) and Masson.
Due to the limited resource of antibodies reacting to mini-pigs, only the antibody for GFP was used for immunohistochemical analysis. Anti-GFP antibody reacted only with cells transduced with GFP gene and was used to determine whether sDFSC seeding cells participated in the newly formed tissues.
The control groups included the following: sTDM without seeding cells and sDFSCs only. The procedures used were the same as those for the experimental group (combination of sTDM and sDFSCs).
2.6. Statistical analysis
All data were expressed as the mean ± standard deviation (SD). Statistical significance was analyzed using SPSS 11.5 software (SPSS, USA). A value of P < 0.05 was considered statistically significant.
3. Results
3.1. Fabrication and detection of sTDM
First, the method used in a previous study3 with human dentin (Group 1) did not work on dentin matrix from swine. Dentinal tubules were not sufficiently exposed and peritubular dentin was not loosened (Fig. 1A and B). In the present study, the EDTA concentration was not changed to alter the demineralization method. However, we extended treatment time for 17% and 10% EDTA, which may mainly contribute to the demineralization of TDM. When the treatment time of 17% EDTA and 10% EDTA (Group 2) were both extended to 10 min, an obvious improvement was observed, with more dentinal tubules exposed (Fig. 1C and D). Furthermore, when the treatment times for 17% EDTA and 10% EDTA were both extended to 15 min, the improvement was not so distinct from Group 2 (Fig. 1E and F). In Group 4, the treatment time for 5% was extended to 10 min and the others were the same as Group 3. The result showed no obvious difference from Group 3 (Fig. 1G and H). Finally, the treatment times for 17% EDTA and 10% EDTA were extended to 20 min and treatment time for 5% EDTA was also 10 min (Group 5). The scaffold obtained was similar to Group 3 and Group 4 (Fig. 1I and J).
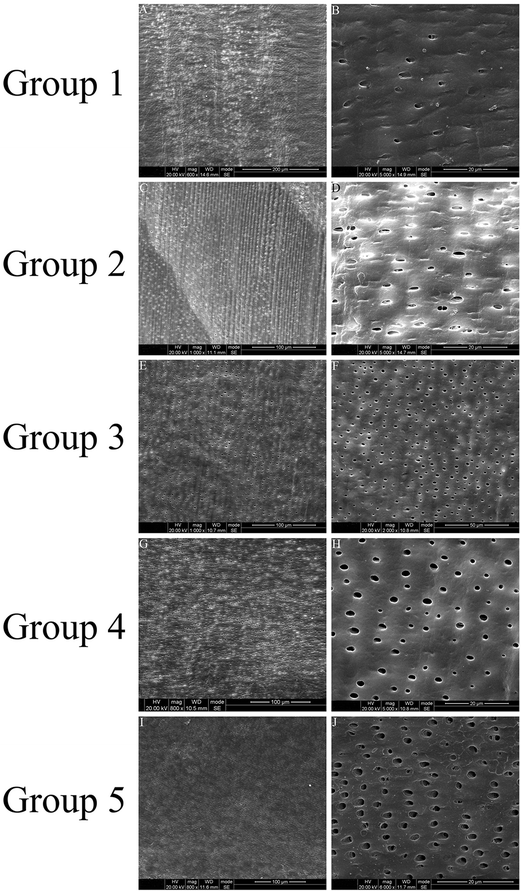 |
| Fig. 1 Structures of sTDM under SEM by EDTA with different treatment times. The results demonstrated the step-by-step process of demineralization by EDTA was shown to be effective and necessary for sTDM. The treatment time of 17% EDTA, 10% EDTA and 5% EDTA should be at least 15 min, 15 min, and 5 min, respectively. | |
Above all, the step-by-step demineralization method by EDTA was shown to be effective and necessary for sTDM, just as for human TDM. To obtain an ideal scaffold, the treatment time of 17% EDTA, 10% EDTA and 5% EDTA should be at least 15 min, 15 min, and 5 min, respectively.
3.2. Biological characteristics of sDFSCs induced by sTDM in vitro
CCK-8 results showed that from day 1 to day 6, the viability of sDFSCs cultured in sTDM liquid extract was significantly higher than that of sDFSCs cultured in normal medium (P < 0.005, Fig. 2), indicating improved cell viability of sDFSCs induced by sTDM and excellent biocompatibility of sTDM.
 |
| Fig. 2 The viability of sDFSCs cultured in sTDM liquid extract or in normal medium from day 1 to day 8. Cell viability in sTDM liquid extract was significantly improved over those cultured in normal medium. | |
3.3. Culture, transduction and characteristics of SDFSCs
Although the growth condition for sDFSCs was the same as that used for human DFSCs (hDFSCs) in previous studies,3 there were some differences in the properties of DFSCs between sDFSCs and hDFSCs. The swine cells were much smaller and presented spindle or polygon, flash slender; their cytoplasm was transparent with a circular or elliptical cell nucleus (Fig. 3A). Cells were arranged into radial and circinate. After passage 10, the growth rate of sDFSCs became slow and cell volume increased gradually (Fig. 3B). After being transduced with the GFP gene, the growth rate of the sDFSCs was not obviously changed and the success rate of transduction was relatively high (nearly 90%) (Fig. 3C). Homogeneous electron-dense granules, identified as marker for sDFSCs, were observed in cultured cells under TEM examination (Fig. 3D).
 |
| Fig. 3 DFSCs of swine of primary culture under light microscope examination (a). The shape of sDFSCs was not obviously changed at passage 5 (b). After being transduced with GFP gene, the growth rate of the sDFCs was not obviously changed and the success rate of transduction was nearly 90% (c). TEM examination revealed homogeneous electron-dense granules, which were identified as the marker for DFSCs (d). | |
Immunofluorescent staining showed that the sDFSCs were positive for vimentin (Fig. 4A) but negative for CK-14 (Fig. 4B). Positive staining for vimentin and negative staining for CK-14 is indicative of mesenchymal cells and epithelial cells, respectively, indicating that the cultured cells are mesenchymal cells without the co-presence of epithelial cells. After being induced with osteogenic medium for 15 days, sDFSCs formed mineralized nodules as indicated by alizarin red staining (Fig. 4C). After being cultured under adipogenic conditions for 14 days, sDFSCs formed lipid droplets, which were stained with Oil Red O (Fig. 4D). Under neurogenic induction, sDFSCs were observed to undergo morphological changes and form neuron-like cells positive for βIII-tubulin, which is regarded as a marker of neurons (Fig. 4E and F). These results clearly demonstrated the multi-potential differentiation of sDFSCs.
 |
| Fig. 4 DFSCs of swine were positive for vimentin (a) a marker for mesenchymal cells but negative for CK-14, an epithelial marker (b). Alizarin red staining showed the mineralized nodules for cells grown under osteogenic conditions (c). After being cultured under adipogenic conditions for 20 days, lipid droplets were observed (d). Under neurogenic conditions, DFSCs underwent morphological changes (e) and expressed βIII-tubulin, the neurogenic maker (f). | |
Cell-surface antigen profiles obtained (Fig. 5) showed that sDFSCs were positive for CD146, CD90 and CD44, which are regarded as markers of mesenchymal stem cells.
 |
| Fig. 5 FCM showed that sDFSCs were positive for CD146, CD90 and CD44, markers of mesenchymal stem cells but negative for CD31, CD45 and CD29. | |
3.4. In vivo regeneration of tooth roots by the combination of sTDM/DFSCs
The alveolar fossa microenvironment was created before the implantation of sTDM and sDFSCs (Fig. 6A and B). All experimental animals recovered quickly and no inflammatory responses were noted with any of the implants, indicating good biocompatibility of sTDM.
 |
| Fig. 6 Creation of the alveolar fossa microenvironment and implantation of sTDMs in combination with sDFSCs. Panels (a) and (b) show the process of alveolar fossa microenvironment fabrication and the implantation of sTDM and sDFCs into the alveolar fossa created before. Micro-CT analysis showed that the growth of the implanted materials was satisfied with clear root profile and no root resorption. After being rehabilitated with metal post, it also had an ideal stability and could act as a natural tooth root (c). Panels (d and e) showed the formation of periodontal tissues, including cementum-like layer and PL-like tissues on the alveolar side. Ossification lacunars were also observed at the newly regenerated cementum-like layer (indicated by arrows). Panels (g and h) showed the formation of distinctive dentin structures (predentin, dentin and odontoblast) and blood vessels on the pulp–dentin side. Most importantly, the regenerated tissues and their surrounding cells in these samples stained positively for GFP (f), indicating that implanted sDFSCs actively participate in regeneration of periodontal tissues. GFP-positive cells were widely distributed in the regenerated pulp–dentin complex tissues (i), implying that the implanted sDFSCs also participate in regeneration of pulp–dentin complex. Note: p: predentin; PL: periodontal ligament; C: cementum-like tissue; arrow: ossification lacunars. | |
Micro-CT analysis revealed the newly regenerated tissues rich in mineralized matrix formation in the group receiving sDFSCs in combination with sTDM after incubation in vivo for 8 weeks (Fig. 6C). A low density belt-like periodontal ligament space existed between the regenerated tooth root and alveolar bone.
Histological examination also showed that sTDM was capable of integrating with host tissues without inducing any inflammatory responses, further demonstrating good biocompatibility. HE and Masson staining showed the generation of a pulp–dentin complex, a cementum-like layer, and a PL-like tissue (Sharpey fiber) inserted into the alveolar bone of the host (Fig. 6D and E). At the side of the inner side (pulp–dentin side) of the scaffold, distinctive dentin–pulp-like structures, including dentinal tubules, predentin, polarizing odontoblast-like structures, collagen fibers, and blood vessels were clearly observed (Fig. 6G and H). More importantly, at the alveolar side, cementum-like layer and PL-like tissues were formed. Ossification lacunars were also observed in the newly regenerated cementum-like layer. The PL-like tissues were arranged vertically at the surface of the cementum-like layer (Fig. 6D and E). To our interest, the shape of the regenerated tissues remained highly consistent with the shape of the original sTDM observed in our previous study,3 indicating that tooth roots with various shapes may be achieved by the means of the TDM shapes.
Labeling experiments showed that GFP-positive cells were widely distributed in the regenerated periodontal tissues, the pulp–dentin complex tissue and the alveolar bone surrounding the grafts (Fig. 6F and I). These results suggest that implanted sDFSCs could participate in cell proliferation and differentiation into the pulp–dentin complex and cementum–PL complexes. In the control groups, the newly regenerated tissues were not found (data not shown). In our study, the sDFSCs were seeded onto sTDM at a relatively low density, which could sufficiently induce odontogenic differentiation of the seeding cells. In addition, the combination of sTDM and sDFSCs were cultured 72 h before implantation to make these seeding cells adhere tightly, or even form a membrane with cells and extracellular matrix, which could act as odontogenic inductive microenvironment. The results have shown that our protocol of sTDM to be used for reconstruction of tooth roots in vivo was successful.
4. Discussion
Both mouth functions and the esthetics of one's face could be adversely affected by tooth loss. Theoretically, the best option for treating tooth loss may be a biological tooth root with periodontal complex that is made from the patient's own cells and grows in its intended location to replace implant dentures.25 With a tooth root, dentists can make biological or synthetic post/crowns to restore the damaged natural teeth. Recently, great progress has been made in the studies of regeneration of tooth root. Tooth root has been reconstructed with or without scaffolds and odontogenic cells.20,26 However, only PLs or bone-like tissues were formed. This may be due to the reason that most scaffold materials lack odontogenic ability.4,27–29
The organic matrix of dentin is known to contain a number of important proteins, which are necessary for tooth development, mineralization, and regeneration.13 TDM could act not only as an inductive microenvironment for regeneration of pulp–dentin complex, but also as scaffolds for regenerating tooth roots due to high plasticity and strength. Previous studies also have indicated that TDM, regarded as a natural acellular matrix (ACM) scaffold, is an inductive microenvironment suitable for the reconstruction of tooth root.2,3,7,8 Due to its non-immunogenicity, suitable mechanical properties, and richness in potentially dentinogenetic factors, natural acellular dentin matrix may be a suitable scaffold for tooth tissue engineering.8,30 It was reported that the organic matrix of dentin could contain approximately 30% collagen, noncollagenous proteins (NCPs), and growth factors, which have been shown to be important in dentin development, mineralization, and regeneration.31 The novelty of dentin matrix as a scaffold is its odontogenic induction, which plays a key role in regeneration of dental tissues. Recently, we have successfully regenerated tooth root in rat in situ with treated dentin matrix and seeding cells, which has proved our hypothesis.11 In this study, we used sTDM as scaffold to further investigate the odontogenic potential of this biomaterial in big animal model and the result has proved the ideal odontogenic ability of TDM scaffold again. Our results showed that cell viability of sDFSCs induced by sTDM was significantly improved, which was similar with our previous studies.3,8
Swine are species with high similarity to humans in genome and structure and are considered as a resource for biological scaffolds. Therefore, xenogenic TDM derived from swine should be of importance in future clinical application. As in our previous study, we also used EDTA to treat dentin matrix of swine to sufficiently expose dentin tubules, loosen the intertubular and peritubular dentin structures and maintain functional proteins and factors.3 The protocol of dentin matrix fabrication method should be highly optimized. The reasons were as followed: (1) excessive demineralization could destroy the structure of dentin matrix that may damage the functionality of odontogenic proteins and factors; (2) insufficient demineralization could generate a scaffold with less odontogenic ability. However, for swine dentin matrix, dentin tubules could not be exposed sufficiently and intertubular and peritubular dentin structures could not be adequately loosened using the fabrication method developed for human dentin. Because of these species-specific differences in dentin matrix between human and swine, different treatment times were utilized in this study. By prolonging the treatment time, the dentin tubules of sTDM were sufficiently exposed, which allowed the odontogenic factors and proteins to be released. These factors play a key role in odontogenesis and differentiation of seeding cells. Furthermore, they form a network for the formation of regenerative dental tissues and control mineralization during tooth regeneration and development.32–36 Moreover, a step-by-step demineralization is necessary to maintain functional odontogenic proteins and factors. However, more treatment time with EDTA may destroy these factors and proteins, which could reduce the odontogenic ability of the scaffold. Our results indicated that the fabrication protocol was effectively optimized for TDM derived from swine, with dentin tubules sufficiently exposed or intertubular and peritubular dentin structure loosened.
In addition to the odontogenic inductivity of sTDM, the inductive microenvironment also plays a key role in successful tissue regeneration.37–39 Alveolar bone is the sole environment for the development and growth of tooth root under natural conditions. Thus, we hypothesize that a microenvironment of alveolar fossa is necessary for reconstruction of tooth root. This requirement of alveolar fossa may explain why only dentin tissue could be regenerated without formation of any periodontal tissues, when DFCs/TDM are implanted into subcutaneous pocket in mouse. In this study, the highly mineralized alveolar fossa was adopted as the implantation site. The root-like tissues with pulp–dentin complex and a PL–cementum complex were observed, which was in accordance with the observation made in our previous study with a mouse model.2 In addition, it was found that regenerated tissues conformed to the original shape of TDM scaffolds, which demonstrates the potential for generating prefabricated shapes of TDM for suitable tooth root morphology. Furthermore, the GFP-positive cells were widely distributed around the regenerated periodontal tissues, the pulp–dentin complex tissue and the alveolar bone, indicating that the implanted seeding cells were responsible for the regenerated tissues. Therefore, the combination of the alveolar fossa and TDM could be an inductive microenvironment quite suitable for reconstruction of tooth roots in big animal model. Under the dual microenvironment of alveolar fossa and sTDM, both the seeding cells and the host cells could contribute to the formation of dentin–pulp complex, including predentin, odontoblast layer, fibroblasts and blood vessels, as well as PL–cementum complex.
As the precursor cells of periodontal tissues cells, including fibroblasts in the PDL, alveolar bone cells, and cementoblasts,31,40 dental follicle stem cells (DFSCs), are found to be capable to differentiate into odontoblasts and form complete dentin tissues when seeded onto TDM scaffolds.3,8 In addition, only one type of seeding cell was required in our process of tooth root regeneration, and this cell, DFSCs, could differentiate into two types of cells to form both the pulp–dentin complex and the PDL/cementum-like tissue. Therefore, we have identified DFSCs as suitable candidate seeding cells for tooth root regeneration. Our results showed root-like tissues consist of pulp–dentin complex and PL–cementum complex and these seeding cells (sDFSCs) were proven to participate in formation of these newly regenerated tissues. In this study, tooth roots similar to natural tooth roots using a single type of seeding cell (sDFSCs) in combination with sTDM were successfully regenerated.
5. Conclusion
In summary, the protocol of sTDM fabrication has be highly optimized and unified to get the best results for clinical application. Most importantly, sTDM scaffolds were capable of inducing tooth root regeneration, with a pulp–dentin complex, a cementum-like layer, and a PL-like tissue, in an in vivo implantation. Our study has indicated that the treated dentin matrix could be an ideal scaffold for tooth regeneration once again and provides a new and more viable source of scaffold for tooth regeneration in the future. Although tooth roots similar to natural tooth roots has been successfully regenerated in this study by using a single type of seeding cell in combination with TDM in swine, several scientific problems remain to be answered. Because of limited antibodies available for swine, the identification of regenerated tooth roots has not been accomplished yet. Immune rejection is another major problem to be overcome. Whether TDM obtained from animals (e.g. swine) or other human tissues could be used in repairing and replacing damaged and lost tooth tissues in patients needs further investigation. Moreover, clear definition of the important roles the seeding cells and host cells from the mandible play remains.
5.1. Perspectives
Recent research on TDM and its role in making a tooth root provide a substantial basis upon which we can begin to explore their therapeutic potential at the preclinical level. However, it may be much more complicated to make a tooth root with masticatory function and supportive tissues. Due to species differences, whether TDM from swine could be used on human remains unclear. Avoiding host–graft immunorejection in the jaws is a key in the clinical process. Furthermore, it remains unclear what exactly the shape and size of bioengineered tooth root should be adopted for better retention and function in the human jaw. Although there are many technical barriers to overcome, tissue engineering-based approaches to tooth reconstruction will provide predictable applications for treatment of tooth loss and other dental diseases.
Acknowledgements
This study was supported by the Nature Science Foundation of China (China, 81300843 and 31400839).
References
- M. Yan, Y. Yu, G. Zhang, C. Tang and J. Yu, Stem Cell Rev., 2011, 7, 161–171 CrossRef PubMed.
- W. Guo, K. Gong, H. Shi, G. Zhu, Y. He, B. Ding, L. Wen and Y. Jin, Biomaterials, 2012, 33, 1291–1302 CrossRef CAS PubMed.
- R. Li, W. Guo, B. Yang, L. Guo, L. Sheng, G. Chen, Y. Li, Q. Zou, D. Xie, X. An, Y. Chen and W. Tian, Biomaterials, 2011, 32, 4525–4538 CrossRef CAS PubMed.
- Z. Yuan, H. Nie, S. Wang, C. H. Lee, A. Li, S. Y. Fu, H. Zhou, L. Chen and J. J. Mao, Tissue Eng., Part B, 2011, 17, 373–388 CrossRef CAS PubMed.
- S. E. Eckert, Y. G. Choi, A. R. Sanchez and S. Koka, Int. J. Oral Maxillofac. Implants, 2005, 20, 406–415 Search PubMed.
- F. Wei, T. Song, G. Ding, J. Xu, Y. Liu, D. Liu, Z. Fan, C. Zhang, S. Shi and S. Wang, Stem Cells Dev., 2013, 22, 1752–1762 CrossRef CAS PubMed.
- B. Yang, G. Chen, J. Li, Q. Zou, D. Xie, Y. Chen, H. Wang, X. Zheng, J. Long, W. Tang, W. Guo and W. Tian, Biomaterials, 2012, 33, 2449–2461 CrossRef CAS PubMed.
- W. Guo, Y. He, X. Zhang, W. Lu, C. Wang, H. Yu, Y. Liu, Y. Li, Y. Zhou, J. Zhou, M. Zhang, Z. Deng and Y. Jin, Biomaterials, 2009, 30, 6708–6723 CrossRef CAS PubMed.
- S. P. Ho, M. P. Kurylo, T. K. Fong, S. S. Lee, H. D. Wagner, M. I. Ryder and G. W. Marshall, Biomaterials, 2010, 31, 6635–6646 CrossRef CAS PubMed.
- J. Wang, H. Ma, X. Jin, J. Hu, X. Liu, L. Ni and P. X. Ma, Biomaterials, 2011, 32, 7822–7830 CrossRef CAS PubMed.
- W. Guo, L. Chen, K. Gong, B. Ding, Y. Duan and Y. Jin, Tissue Eng., Part A, 2012, 18, 459–470 CrossRef CAS PubMed.
- O. V. Horst, M. G. Chavez, A. H. Jheon, T. Desai and O. D. Klein, Dent. Clin. North Am., 2012, 56, 495–520 CrossRef PubMed.
- S. Y. Chun, H. J. Lee, Y. A. Choi, K. M. Kim, S. H. Baek, H. S. Park, J. Y. Kim, J. M. Ahn, J. Y. Cho, D. W. Cho, H. I. Shin and E. K. Park, Tissue Eng., Part A, 2011, 17, 181–191 CrossRef CAS PubMed.
- S. Lottanti, H. Gautschi, B. Sener and M. Zehnder, Int. Endod. J., 2009, 42, 335–343 CrossRef CAS PubMed.
- M. Hulsmann, M. Heckendorff and A. Lennon, Int. Endod. J., 2003, 36, 810–830 CrossRef CAS PubMed.
- H. G. Kuah, J. N. Lui, P. S. Tseng and N. N. Chen, J. Endod., 2009, 35, 393–396 CrossRef PubMed.
- I. A. Polejaeva, S. H. Chen, T. D. Vaught, R. L. Page, J. Mullins, S. Ball, Y. Dai, J. Boone, S. Walker, D. L. Ayares, A. Colman and K. H. Campbell, Nature, 2000, 407, 86–90 CrossRef CAS PubMed.
- N. H. Staunstrup, J. Madsen, M. N. Primo, J. Li, Y. Liu, P. M. Kragh, R. Li, M. Schmidt, S. Purup, F. Dagnaes-Hansen, L. Svensson, T. K. Petersen, H. Callesen, L. Bolund and J. G. Mikkelsen, PLoS One, 2012, 7, e36658 CAS.
- S. Wang, Y. Liu, D. Fang and S. Shi, Oral Dis., 2007, 13, 530–537 CrossRef CAS PubMed.
- W. Sonoyama, Y. Liu, D. Fang, T. Yamaza, B. M. Seo, C. Zhang, H. Liu, S. Gronthos, C. Y. Wang, S. Wang and S. Shi, PLoS One, 2006, 1, e79 Search PubMed.
- G. Chen, J. Chen, B. Yang, L. Li, X. Luo, X. Zhang, L. Feng, Z. Jiang, M. Yu, W. Guo and W. Tian, Biomaterials, 2015, 52, 56–70 CrossRef CAS PubMed.
- X. Luo, B. Yang, L. Sheng, J. Chen, H. Li, L. Xie, G. Chen, M. Yu, W. Guo and W. Tian, Biomaterials, 2015, 57, 59–72 CrossRef CAS PubMed.
- S. Tsuchiya, S. Ohshima, Y. Yamakoshi, J. P. Simmer and M. J. Honda, Connect. Tissue Res., 2010, 51, 197–207 CrossRef CAS PubMed.
- Y. Y. Jo, H. J. Lee, S. Y. Kook, H. W. Choung, J. Y. Park, J. H. Chung, Y. H. Choung, E. S. Kim, H. C. Yang and P. H. Choung, Tissue Eng., 2007, 13, 767–773 CrossRef CAS PubMed.
- J. Yu, J. Shi and Y. Jin, Tissue Eng., Part B, 2008, 14, 307–319 CrossRef CAS PubMed.
- M. Oshima, M. Mizuno, A. Imamura, M. Ogawa, M. Yasukawa, H. Yamazaki, R. Morita, E. Ikeda, K. Nakao, T. Takano-Yamamoto, S. Kasugai, M. Saito and T. Tsuji, PLoS One, 2011, 6, e21531 CAS.
- F. J. Rodriguez-Lozano, C. Bueno, C. L. Insausti, L. Meseguer, M. C. Ramirez, M. Blanquer, N. Marin, S. Martinez and J. M. Moraleda, Int. Endod. J., 2011, 44, 800–806 CrossRef CAS PubMed.
- S. E. Duailibi, M. T. Duailibi, W. Zhang, R. Asrican, J. P. Vacanti and P. C. Yelick, J. Dent. Res., 2008, 87, 745–750 CrossRef CAS PubMed.
- T. Ohara, T. Itaya, K. Usami, Y. Ando, H. Sakurai, M. J. Honda, M. Ueda and H. Kagami, J. Biomed. Mater. Res., Part A, 2010, 94, 800–805 Search PubMed.
- K. U. Zaman, T. Sugaya, O. Hongo and H. Kato, J. Periodontol., 2000, 71, 1094–1099 CrossRef CAS PubMed.
- M. Jagr, A. Eckhardt, S. Pataridis, Z. Broukal, J. Duskova and I. Miksik, Physiol. Res., 2014, 63(suppl. 1), S141–S154 CAS.
- A. Piattelli, C. Rubini, M. Fioroni, D. Tripodi and R. Strocchi, Int. Endod. J., 2004, 37, 114–119 CrossRef CAS PubMed.
- C. C. Reed and R. V. Iozzo, Glycoconjugate J., 2002, 19, 249–255 CrossRef CAS PubMed.
- Y. Yamaguchi, D. M. Mann and E. Ruoslahti, Nature, 1990, 346, 281–284 CrossRef CAS PubMed.
- E. Schonherr, M. Broszat, E. Brandan, P. Bruckner and H. Kresse, Arch. Biochem. Biophys., 1998, 355, 241–248 CrossRef CAS PubMed.
- Z. Ferdous, V. M. Wei, R. Iozzo, M. Hook and K. J. Grande-Allen, J. Biol. Chem., 2007, 282, 35887–35898 CrossRef CAS PubMed.
- S. F. Badylak and R. M. Nerem, Proc. Natl. Acad. Sci. U. S. A., 2010, 107, 3285–3286 CrossRef CAS PubMed.
- F. Berthiaume, T. J. Maguire and M. L. Yarmush, Annu. Rev. Chem. Biomol. Eng., 2011, 2, 403–430 CrossRef PubMed.
- J. Ringe, G. R. Burmester and M. Sittinger, Nat. Rev. Rheumatol., 2012, 8, 493–498 CrossRef CAS PubMed.
- M. J. Honda, M. Imaizumi, S. Tsuchiya and C. Morsczeck, J. Oral Sci., 2010, 52, 541–552 CrossRef PubMed.
Footnote |
† Electronic supplementary information (ESI) available. See DOI: 10.1039/c5ra27332c |
|
This journal is © The Royal Society of Chemistry 2016 |