DOI:
10.1039/C5RA26271B
(Paper)
RSC Adv., 2016,
6, 22436-22446
A comprehensive study on the improvement of oxidation stability and NOx emission levels by antioxidant addition to biodiesel blends in a light-duty diesel engine
Received
9th December 2015
, Accepted 23rd January 2016
First published on 26th January 2016
Abstract
Moringa oleifera oil, a non-edible biodiesel feedstock with high unsaturated fatty acid content, was used in this study. MB20 (20% Moringa oil methyl ester and 80% diesel fuel blend) was mixed with three antioxidants, namely, N,N′-diphenyl-1,4-phenylenediamine (DPPD), N-phenyl-1,4-phenylenediamine (NPPD) and 2-ethylhexyl nitrate (EHN), at a concentration of 1000 ppm. The effects of these antioxidants on the oxidation stability of biodiesel as well as on the exhaust emission and performance of a single-cylinder diesel engine were analysed. After the Rancimat test, oxidation stability was enhanced by the antioxidants in the order of DPPD > NPPD > EHN. Results also showed that DPPD-, NPPD- and EHN-treated blends reduced NOx emissions within 5.9–8.80% compared with those in the untreated blend because of suppressed free radical formation. Antioxidant-treated blends contained high amounts of carbon monoxide and hydrocarbon and showed improved smoke opacity, thereby indicating that emissions were below the diesel fuel emission levels. Results demonstrated that antioxidant addition to MB20 improves engine performance characteristics. This study shows that MB20 blends with antioxidants can be used in diesel engines without any modification.
1. Introduction
Significant increase in energy demand in power generation and transport sectors, inadequate fossil fuel accessibility and negative environmental effects have boosted research on alternative renewable fuels for conventional fuels.1 Fossil fuel combustion is the primary cause of global increment of carbon dioxide (CO2) emission every year and intensifies air pollution and global warming issues.2 Diesel vehicles emit significant amounts of nitrogen oxides (NOx) and particulate matter (PM),3 which confer lung problems, neurodegenerative dysfunction and cardiovascular diseases.4,5 NOx emission not only affects human health but also the environment by causing acid rain, which severely harms aquatic and terrestrial ecosystems. Amongst all unconventional sources of renewable energy, biodiesels have received increased attention because of their renewability, nontoxicity and biodegradability.6,7 Biodiesels can be derived from renewable feedstocks, which basically lack sulphur and aromatic contents. These energy sources are less toxic and offer positive energy balance and other chemical properties superior to those of fossil-based diesel fuels. Moringaceae is a single-genus family of oilseed trees and comprise 14 known species. Moringa oleifera is the most widely known and utilised among these species.8 M. oleifera is indigenous to India, Africa, Arabia and Southeast Asia but can also be found in Cambodia, the Philippines and North America.9 The plant optimally grows in tropical insular weather, exhibits drought tolerance and can be sustained in soil with poor quality and a wide range of rainfall intensity (25 cm to 300 cm per year).10 M. oleifera seeds contain 33% to 41% (w/w) vegetable oil. Several researchers investigated the composition and fatty acid profile of M. oleifera and concluded that the oil contains high amounts of oleic acid (>70%).11–13 Therefore, M. oleifera is a potential source of biodiesel. The present study focuses on 20% blend of Moringa biodiesel with diesel because previous studies suggest that this blend provides the optimal performance among biodiesel–diesel blends.14,15
Low oxidation and storage stability are a major limitation that restricts biodiesel application and can be resolved by adding antioxidants.16,17 Antioxidants significantly hinder oxidation and increase biodiesel stability. Biodiesel becomes corrupted primarily because of its autoxidative nature in the presence of atmospheric air.18,19 Antioxidants may play an important role in preventing biodiesel oxidation without negatively influencing fuel properties. Antioxidants are categorised based on their activity into free radical terminators, metal ion chelators, agents catalysing lipid oxidation or oxygen scavengers, which react with oxygen in closed systems. These antioxidants are known as primary antioxidants.20 The stability of biodiesel increases because of the reaction of antioxidants with high-energy lipid radicals and its transformation into stable products. Commonly used antioxidants are phenolic antioxidants, which are categorised as free-radical terminators and amine antioxidants. The stabiliser factor is an important parameter that defines the efficiency of an antioxidant. This parameter may be calculated using the formula F = IPx/IPo, where IPx and IPo are the induction periods in the presence and absence of antioxidant, respectively.21 The reactivity of phenoxyl radical (R_O˙) tends to weaken and restrict oxidation because of scavenging reactive radicals, including peroxyl radicals (ROO˙), in the –OH group of the antioxidant. Generally, phenolic antioxidants, such as TBHQ, BHT and BHA, are used to protect biodiesel from degradation. These antioxidants can effectively control free radicals at room temperature but show rapid reduction in reactivity at high temperatures. A quantum-chemical study of an aromatic amine, namely, N,N′-diphenyl-p-phenylenediamine (DPPD) demonstrated that this compound maintains its antioxidant reactivity even at high temperatures.22 Amines contain a couple of p-electrons on nitrogen molecules and exhibit less electron affinity compared with O2. Hence, amines can serve as electron-donor reactants in a charge-transfer complex with oxygen-containing atoms and radicals. In addition, the hydrogen atom from the N–H bond of aromatic amines can be separated more easily than that from the O–H bond of phenols because the N–H hydrogen bond is not as strong as the O–H hydrogen bond.23
Numerous studies have been conducted to determine the effect of antioxidants on oxidation stability, engine combustion, performance and emission characteristics of biodiesel.24–30 IIeri et al.26 investigated the influences of four antioxidants at proportions of 500, 750 and 1000 ppm on 20% canola biodiesel and diesel blends to determine the performance and emission of a direct injection (DI) diesel engine. As an antioxidant, the molecular structure of 2-ethylhexyl nitrate (EHN) contains nitrogen and hence reduces NOx emission by 4.63% on the average; CO emission is enhanced for all antioxidants used, and TBHQ shows the maximum reduction. Varathrajan et al.27 added two aromatic amine antioxidants, namely, DPPD and N-phenyl-1,4-phenylenediamine (NPPD), to soybean biodiesel in a single-cylinder diesel engine; biodiesel treated with 20% DPPD antioxidant showed decreased NO emission by 9.35%, with increments of CO and hydrocarbon (HC) levels at 9.09% and 10.52%, respectively. In another study by Varatharajan et al.,29 four antioxidants were used at a proportion of 0.025%-m in Jatropha biodiesel. The results showed NOx emission in sample with p-phenylenediamine reduced by 43.55% compared with that in pure biodiesel, whereas HC and CO emissions increased. Palash et al.28 studied the DPPD antioxidant at a proportion of 0.15%-m with Jatropha biodiesel and found that NOx was reduced at 3.503–16.54%, BP and BSFC were only slightly reduced, and HC and CO were retained or became lower. Ryu et al.30 incorporated five different antioxidants in soybean biodiesel and reported that the stability of TBHQ was superior among the other antioxidants but did not significant change smoke, HC and NOx emissions. However, the effect of AO on the oxidation stability of Moringa methyl ester as well as the performance and exhaust emission of biodiesel with and without AO have not been investigated. Despite the use of synthetic antioxidants in diesel–biodiesel blends,31,32 problems on biodiesel related to NOx emission persist.
1.1. Study objectives
Considerable research has been conducted on the effect of antioxidants on the oxidation stability of biodiesel, as well as their effect on engine performance and emission characteristics. However, the effect of aromatic amine antioxidants, such as DPPD, NPPD and synthetic antioxidant EHN, has not yet been conducted on the oxidation stability of Moringa biodiesel. No reports are available on engine performance and emission characteristics of a single-cylinder diesel engine fuelled with Moringa biodiesel treated with DPPD, NPPD and EHN. Thus, this study aims to examine the effect of two highly promising aromatic amine antioxidants (DPPD and NPPD) and synthetic antioxidant EHN on the oxidation stability of Moringa biodiesel in terms of engine emission and performance in a single-cylinder diesel engine.
2. Materials and methods
2.1. Feedstock and antioxidants
Crude M. oleifera oil (CMOO) was purchased from the Kanta Enterprise, India; its physico-chemical properties are shown in Table 1. The biodiesel was produced from CMOO. In this study, DPPD, NPPD and EHN were chosen as test antioxidant (AO) with the selected biodiesel. DPPD, NPPD and EHN antioxidants were obtained from Sigma-Aldrich (India). Sulphuric acid (H2SO4), anhydrous sodium sulphate (Na2SO4), methanol (CH3OH), potassium hydroxide (KOH) and filter paper were used to produce biodiesel.
Table 1 Properties of crude Moringa oleifera oil
Properties |
Value |
Calorific value (MJ kg−1) |
38.050 |
Density (kg m−3) |
897.5 |
CFPP (°C) |
18 |
Kinematic viscosity at 40 °C (mm2 s−1) |
43.337 |
Dynamic viscosity at 40 °C (mPa s) |
38.897 |
Flash point (°C) |
268.5 |
Pour point (°C) |
11 |
Cloud point (°C) |
10 |
Acid value (mg KOH per g oil) |
8.62 |
% of free fatty acid (FFA%) |
4.33 |
2.2. Production process of biodiesel
Crude M. oleifera (CMO) oil is highly acidic (Table 1) and thus presents a problem during separation. Hence, a two-step process (acid–base catalyst) was recommended to convert M. oleifera oil into biodiesel (methyl ester). Biodiesel production was performed at the vitality lab of University Malaya by using 1 L clump reactor with a reflux condenser, magnetic stirrer, thermometer and sampling outlet.
2.2.1. Acid catalysis (esterification). For production of biodiesel, the acid-catalysed procedure was applied prior to transesterification to decrease the high acidity of unrefined oils. In this regard, a molar proportion of 12
:
1 (methanol to CMOO) and 1% (v/v) of sulphuric acid (H2SO4) were added to the preheated oil at 60 °C for 3 h and 600 rpm blending velocity. After the fulfilment of the reaction, the resultant mixture was exchanged to an isolating pipe to discrete the esterified oil (lower layer) from the upper layer, which incorporates an overabundance of alcohol, sulphuric acid and debasements. The lower layer was then delivered into a control turning evaporator (IKA) and warmed at 60 °C under vacuum conditions for 1 h to expel methanol and water from the esterified oil.
2.2.2. Alkaline catalysis (transesterification). In base-catalysed procedures, a molar proportion of 6
:
1 of methanol and 1% (m/m) KOH were added to the preheated esterified M. oleifera oil at 60 °C for 2 h and 600 rpm blending rate. Upon reaction completion, the delivered methyl ester was saved in a detachment pipe for 16 h to separate glycerol from methyl ester. The lower layer, which contained glycerol and pollution, was depleted.
2.2.3. Post-treatment process. Excess methanol was removed by pouring methyl ester into a rotating evaporator. This step was followed by cleaning with hot refined water to uproot the entrained contaminations and glycerol. In this procedure, 50% (v/v) of refined water at 60 °C was splashed over the surface of the ester and mixed gently. This procedure was rehashed for few times until the pH of biodiesel became impartial. The lower layer was tossed, and the upper layer was placed into a flask and dried utilising Na2SO4 and further dried utilising a control revolving evaporator (IKA). Finally, the created biodiesel was filtered using filter paper to obtain pure biodiesel.
2.3. Fatty acid composition
The fatty acid composition of M. oleifera methyl ester (MOME) was measured through gas chromatography (GC) (Agilent 6890 model, USA). Briefly, 1 μL of the biodiesel sample was placed into the GC column equipped with a FID (flame ionisation detector) and BPX70 capillary column (dimensions 30 m × 0.25 μm × 0.32 mm inner diameter). The primary temperature was maintained at 140 °C for 2 min, increased at a rate of 8.0 °C min−1 until 165–192 °C and lastly, increased at a rate of 8.0 °C min−1 to 220 °C, which was maintained for 5 min. During the operations, the temperatures were set at 140.0, 240.0 and 260.0 °C for the oven, injector and detector ports, respectively. Helium was used as carrier gas, and the linear velocity, column flow rate and head pressure were 24.4 cm s−1, 1.10 mL min−1 and 56.9 kPa, respectively. The fatty acid composition of M. oleifera biodiesel is shown in Table 2.
Table 2 Fatty acid composition of Moringa oil methyl ester
Sl. no. |
Name of fatty acid |
Mass of molecular |
Structure |
Name of systematic |
Chemical Formula |
MOME |
1 |
Caprylic |
144 |
8 : 0 |
Octanoic |
C8H16O2 |
N/D |
2 |
Capric |
172 |
10 : 0 |
Decanoic |
C10H20O2 |
N/D |
3 |
Lauric |
200 |
12 : 0 |
Dodecanoic |
C12H24O2 |
0 |
4 |
Myristic |
228 |
14 : 0 |
Tetradecanoic |
C14H28O2 |
0.1 |
5 |
Palmitic |
256 |
16 : 0 |
Hexadecanoic |
C16H32O2 |
7.9 |
6 |
Palmitoleic |
254 |
16.1 |
Hexadec-9-enoic |
C16H30O2 |
1.7 |
7 |
Stearic |
284 |
18 : 0 |
Octadecanoic |
C18H36O2 |
5.5 |
8 |
Oleic |
282 |
18 : 1 |
cis-9-Octadecenoic |
C18H34O2 |
74.1 |
9 |
Linoleic |
280 |
18 : 2 |
cis-9-cis-12 Octadecadienoic |
C18H32O2 |
4.1 |
10 |
Linolenic |
278 |
18 : 3 |
cis-9-cis-12 Eicosanoic |
C18H30O2 |
0.2 |
11 |
Arachidic |
312 |
20 : 0 |
C20H40O2 |
2.3 |
12 |
Eicosanoic |
310 |
20 : 1 |
cis-11-Eicosenoic |
C20H38O2 |
1.3 |
13 |
Behenic |
340 |
22 : 0 |
Docosanoic |
C22H44O2 |
2.8 |
From the fatty acid composition it has been shown that Moringa biodiesel contains 10 fatty acids, including five saturated fatty acid esters (FAE, 18.6%), three monounsaturated FAE (77.1%) and two polyunsaturated FAE (4.3%).
2.4. Property analysis of the tested fuel
The physico-concoction properties of the delivered biodiesel were determined by ASTM D6751 and EN 14214 models. Cetane number (CN), iodine value (IV) and saponification value (SV) were measured using the following mathematical statements:15 |
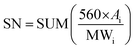 | (1) |
|
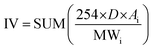 | (2) |
|
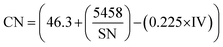 | (3) |
where Ai = the percentage of each component, D = number of double bonds, MWi = molecular weight of each component.
The properties of the biodiesel its blend were measured with and without antioxidants (DPPD, NPPD and EHN) and then compared with ASTM standards. The measured properties of MOME and its blend with and without the addition of antioxidant were found acceptable according to ASTM standards. Table 3 presents the equipment used to determine the properties of pure biodiesel and its blends with and without addition of antioxidants (DPPD, NPPD and EHN). No variation in density or pour point was notable when antioxidant additives were added. On the other hand, the calorific value and flash point were enhanced for blends supplemented with antioxidant. Engine performance was enhanced with increasing calorific value. Hence, biodiesel and its blends possessed lower calorific values compared with diesel. In the engine, oxygen content is vital for proper and complete combustion. After adding antioxidant or without antioxidant (DPPD, NDDP and EHN), we noted that the flash point of each biodiesel and its blend is higher and hence affords secured storage compared with biodiesel. Furthermore, by adding DPPD, NPPD and EHN antioxidants in biodiesel, we improved the oxidation stability and lessened the cloud point. The properties of the tested aromatic amine antioxidants DPPD, NPPD and EHN are given in Table 4.
Table 3 List of instrument details
Equipment |
Property |
Test method |
ASTM D6751 |
Accuracy |
C2000 basic calorimeter (IKA, UK) |
Caloric value |
ASTM D240 |
Report |
±0.1% of reading |
SVM 3000 (Anton Paar, UK) |
Density |
ASTM D7042 |
— |
±0.1 kg m−3 |
SVM 3000 (Anton Paar, UK) |
Kinematic viscosity |
ASTM D7042 |
1.9–6.0 |
±0.35% |
Pensky-martens flash point – automatic NPM 440 (Norma lab, France) |
Flash point |
ASTM D93 |
130 minimum |
±0.1 °C |
873 Rancimat (Metrohm, Switzerland) |
Oxidation stability |
EN ISO 14112 |
3 h minimum |
±0.01 h |
Cloud and pour point tester – automatic NTE 450 (Norma lab, France) |
Cloud and pour point |
ASTM D2500 ASTM D97 |
— |
±0.1 °C |
Cold filter plugging point tester – automatic NTL 450 (Norma lab, France) |
Cold filter plugging point |
ASTM D6371 |
— |
— |
Table 4 Properties of antioxidant
Antioxidant |
Chemical structure |
Molecular weight (g mol−1) |
Chemical formula |
CAS number |
Melting point (°C) |
Assay |
N,N′-Diphenyl-1,4-phenylenediamine (DPPD) |
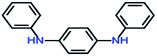 |
260.34 |
C18H16N2 |
74-31-7 |
144 |
97 |
N-Phenyl-1,4-phenylenediamine (NPPD) |
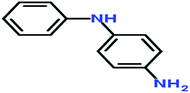 |
184.24 |
C12H12N2 |
101-54-2 |
68 |
98 |
2-Ethylhexyl nitrate (EHN) |
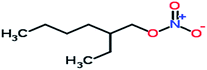 |
175.225 |
C8H17NO3 |
27247-96-7 |
75 |
97 |
2.5. Diesel–biodiesel blends
Moringa biodiesel was mixed with diesel at 20% by volume using a magnetic stirrer (model: IKA® C-MAG HS 7) at 2000 rpm for 30 min and a shaker (model: IKA® KS 130 fundamental) at 400 rpm for 30 min. The physical properties of the biodiesel and its blends were assessed according to ASTM standards.
2.6. Engine test procedure
In this study, single-cylinder, direct-injection and water-cooled diesel engines were employed. The tests were performed in the heat engine laboratory of the Mechanical Engineering department in the University of Malaya. The engine specifications are given in Table 5, and the experimental setup is shown in Fig. 1. All tests were conducted with variation in engine speed starting from 1200 rpm to 2400 rpm at an interval of 200 rpm and full throttle opening condition. The eddy current dynamometer was connected to the test engine, and a positive displacement type flow meter was employed to measure fuel flow during engine operation. Meanwhile, k-type thermocouples were used to measure cooling-water, exhaust-gas, engine-oil and inlet temperatures. For data collection, a DASTEP8 controller was connected through a computer to the tested engine. For each of the tested fuel blends, the data acquisition system was started after few minutes to ensure the removal of diesel residue. The AVL DiCom 4000 gas analyser was adopted to measure exhaust gas parameters, such as HC, CO and NOx. The equipment details of AVL DiCom 4000 gas analyser are shown in Table 6. The engine was running fuel by diesel for at least 15 min to warm up. Then, biodiesel sample was used. Before recording data, all diesel fuel from the fuel flow line was ensured to be cleaned, and the biodiesel was delivered into the engine as fuel. In each case, data were obtained after the engine stabilised. Before engine shutdown, the engine was run by diesel to ensure that the engine was free from biodiesel. Basing on the engine test cell setup (engine and dynamometer), we obtained the accuracies of the measured parameters, such as BP, BSFC and BTE, are ±0.02 kW, ±0.05 g kW h−1 and ±0.5, respectively.
Table 5 Engine specification
Details of engine |
Type |
Single cylinder, WC, 4-cycle diesel engine |
Displacement (cm3) |
638 |
Bore and stroke |
695.5 (L) mm × 348.5 (W) mm × 530 (H) mm |
Continuous rated output |
2400 rpm, 7.7 kW, 10.5 ps |
Maximum power (kW) out put |
2400 rpm, 8.8 kW, 12 ps |
Fuel system |
Distribution type jet pump (indirect injection) |
Lubrication system |
Completed enclosed forced |
Combustion system |
Direct injection |
Cooling system |
Radiator cooling |
Aspiration |
Natural |
![[thin space (1/6-em)]](https://www.rsc.org/images/entities/char_2009.gif) |
Dynamometer details |
Model |
SAJ SE-20 eddy current |
Maximum power |
20 kW |
Maximum speed |
10 000 rpm |
Maximum torque |
80 N m |
Water consumption for maximum power |
14 L min−1 |
Water pressure |
23 lbf in−2 |
Electricity requirement |
220 V, 60 Hz, 0.5 A |
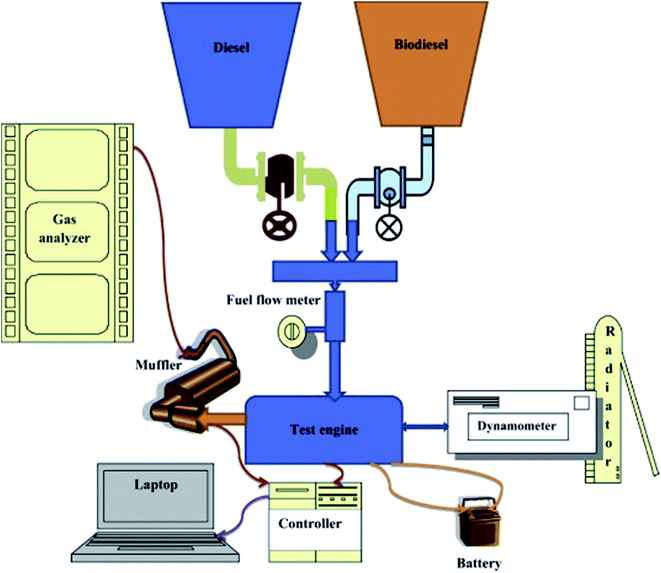 |
| Fig. 1 Experimental setup. | |
Table 6 Exhaust gas analyzer details
Equipment |
Method |
Measurement |
Limit |
Accuracy |
Percentage uncertainties |
AVL DiCom 4000 |
Non-dispersive infrared (NDIR) |
CO |
0–10.00 vol% |
±0.001 vol% |
0.002 vol% |
NDIR |
CO2 |
0–20.00 vol% |
±0.001 vol% |
0.150 vol% |
NDIR |
HC |
0–20 000 ppm |
±l ppm |
2 ppm |
Electro-chemical transmitter |
NOx |
5000 ppm |
±1 ppm |
21 ppm |
3. Results and discussion
3.1. Fuel properties
Table 7 defines the crucial properties of Moringa biodiesel compared with the tested fuels. The kinematic viscosity of diesel is 3.123 mm2 s−1, which is lower than that of MB20 and 12.87% lower than that of MB. The addition of antioxidant to MBD20 increased the kinematic viscosity by about 0.28%. Higher kinematic viscosity implies higher resistance during the flow in the fuel line, which causes a longer delay in the commencement of injection. Moreover, the higher kinematic consistency additionally prompts more poor fuel atomisation.33 As MBD has stearic, oleic and linoleic (C18) (Table 2), the flash point temperature is high, consistent with the ASTM 6751 standards of 130 °C. CN determines the fuel characteristics of auto ignition quality. MBD shows higher CN compared with diesel and satisfied the ASTM 6751 standards at ≥47. The oxidation strength of the neat MBD is comparatively low (4.05 h) because of its high unsaturation rate. Moreover, the 20% blend of MBD with diesel increases oil stability at 6.97 h and satisfies the ASTM 6751 threshold of 3 h. The cloud point decreases with the mixing of biodiesel with diesel.
Table 7 Properties of tested fuels
Properties |
Kinematic viscosity at 40 °C (mm2 s−1) |
Dynamic viscosity (mPa s) |
Calorific value (MJ kg−1) |
Density (kg m−3) |
Flash point (°C) |
Cloud point (°C) |
Pour point (°C) |
Oxidation stability, h |
Iodine value |
Cetane number |
Saponification value |
Viscosity index |
CFPP (°C) |
B0 (diesel) |
3.123 |
2.564 |
45.456 |
826.69 |
67.5 |
7 |
6 |
— |
— |
51 |
— |
90 |
7 |
MB100 |
4.95 |
2.62 |
40.52 |
860.61 |
170.2 |
15 |
16 |
4.05 |
75.2 |
63 |
199 |
190 |
17 |
MB20 |
3.525 |
2.46 |
44.26 |
835.86 |
84.4 |
5 |
5 |
6.97 |
— |
— |
— |
110.56 |
7 |
MB20 DPPD |
3.530 |
2.47 |
44.16 |
836.78 |
87.4 |
7 |
5 |
25.7 |
— |
— |
— |
— |
— |
MB20 NPPD |
3.551 |
2.48 |
44.13 |
836 |
85.3 |
7 |
5 |
18.5 |
— |
— |
|
— |
— |
MB20 EHN |
3.542 |
2.46 |
44.11 |
836.19 |
85.5 |
7 |
5 |
15.7 |
— |
— |
— |
— |
— |
ASTM D6751 |
1.9–6 |
— |
— |
860–900 |
>130 |
— |
— |
3 |
120 max |
47 |
n.s |
— |
— |
EN 14214 |
3.5–5.0 |
— |
— |
860–900 |
>100 |
— |
— |
6 |
— |
51 |
— |
— |
— |
3.2. Effect of antioxidant addition on the oxidation stability of biodiesel
Fig. 2 demonstrates the effect of added antioxidants on the oxidation stability of MB20 for different AO concentrations using Rancimat method. According to the ASTM 6751, the induction period (IP) of MB20 is 6.97 h. The IP of the MB20 fuel increases with increasing proportions of DPPD NPPD and EHN added to the blends. As indicated in Fig. 2, DPPD-treated biodiesel blends exhibit higher stability than the NPPD- and EHN-treated blends in all of the tested concentrations. Hence, four AO concentrations (200, 500, 700 and 1000 ppm) were used in subsequent tests. Amongst these concentrations, 1000 ppm DPPD with MB20 displays the highest stability at 25.7 h. For similar concentrations of NPPD and EHN, the highest stability was noted at 18.5 and 15.7 h, respectively. The stability of 1000 ppm DPPD was 38.9% and 63.69% higher than those of NPPD and EHN, respectively. The hydroxyl gathering of the AO is extremely active; thus, hydrogen transfers from hydroxyl to the oxidised free radical to restrain the oxidation rate in methyl esters.
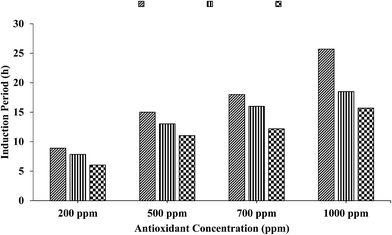 |
| Fig. 2 Biodiesel (MB20) oxidation stability with different concentration of antioxidant. | |
3.3. Performance analysis
3.3.1. Impact of antioxidant addition on engine brake power (BP). The BP results at various speeds with various test fuels at full-throttle condition are indicated in Fig. 3. BP increases consistently up to 2200 rpm and then decreases. The highest BP values are 7.85, 7.49, 7.71, 7.59 and 7.69 kW, respectively, for diesel, MB20, MB20 NPPD, MB20 DPPD and MB20 EHN at 2200 rpm. Therefore, MB20, MB20 NPPD, MB20, DPPD and MB20 EHN produce 4.5%, 1.7%, 3.30% and 2.03% lower maximum output power compared with diesel. Pure biodiesel produces lower power output than diesel because biodiesel possesses higher kinematic viscosity, resulting in less combustion despite the higher oxygen content in molecular structure.34,35 Hence, the average BP for tested blends are 6.37, 6.58, 6.48 and 6.55 kW for MB0, MB20, MB20 + NPPD, MB20 + DPPD and MB20 + EHN, respectively. These values correspond to averages of 5.2%, 2.08%, 3.70% and 2.52% lower power compared with B0. Similar results were found by Rizwanul et al.31 and Kivevele et al.36 The results can be attributed to the lower calorific values and lower kinematic viscosities of the tested blends compared with B0.14 On the other hand, MB20 + NPPD, MB20 + DPPD and MB20 + EHN produce higher BP compared with MB20. The higher viscosity and density of antioxidant-treated biodiesel are attributed to its large injection for the same volume fuel.37,38 Furthermore, less leakage arises from the fuel pump because of the higher viscosity of the blends.39,40 By adding antioxidants in the blends, greater mass flow and lower heat energy are achieved.41
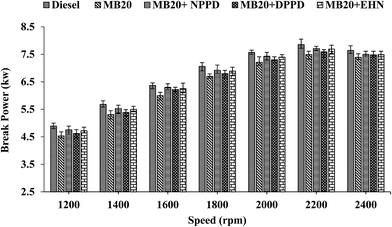 |
| Fig. 3 Brake power variation at different speed for tested fuels. | |
3.3.2. Effect of antioxidant addition on brake specific consumption (BSFC). BSFC varies with changes in speed of the tested blends, as shown in Fig. 4. BSFC decreases linearly up to 1800 rpm with increasing speed and then gradually increases. The maximum BSFC was noted at 1200 rpm. MB20 biodiesel shows higher BSFC for all speeds throughout the experiment. The mean BSFC values are 261.42, 291.39, 283.57, 278.47 and 280.49 g kW h−1 for pure diesel, MB20, MB20 + NPPD, MB20 + DPPD and MB20 + EHN, respectively. From this calculated data, we noted that the mean BSFC of MB20 is 11% higher than that of pure diesel because of low biodiesel heating. By contrast, the addition of NPPD, DPPD and EHN with MB20 shows an average reduction of 2.68%, 4.44% and 3.76% BSFC compared with MB20. Normally, biodiesel possesses lower calorific value because of its fuel-borne oxygen. A previous study showed that addition of antioxidant to B20 lessens the calorific value, and BSFC decreases because of the higher power output.30,36 From this investigation, we demonstrated that the addition of antioxidants (NPPD, DPPD and EHN) to MB20 could significantly decrease the average BSFC. Similar trends were recently found from various biodiesel blends, such as palm and Calophyllum inophyllum, by different authors.31,34 The reduction in BSFC may be due to reduced friction properties of amines.27,28
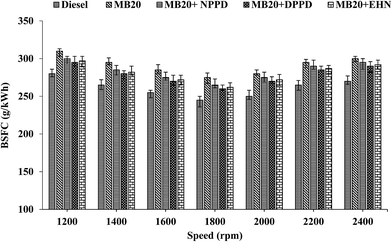 |
| Fig. 4 BSFC variation at different speed for tested fuels. | |
3.3.3. Effect of antioxidant addition on brake thermal efficiency (BTE). BTE fluctuates with varied speed for all the tested blends (Fig. 5). The maximum value of BTE is detected at 1800 rpm. The maximum values of BTE at this speed are 33.05%, 30.26%, 31.48%, 32.10% and 31.87% for B0, MB20, MB20 + NPPD, MB20 + DPPD and MB20 + EHN, respectively. Throughout all speeds tested, MB20 shows the lowest BTE and pure diesel displays the highest BTE. However, the mean BTE values for all tested blends are 31.03%, 28.6%, 29.46%, 30.02% and 29.81% for B0, MB20, MB20 + NPPD, MB20 + DPPD and MB20 + EHN, respectively. Hence, pure diesel produced 7.8%, 5.05%, 3.25% and 3.90%% higher BTE compared with MB20, MB20 + DPPD, MB20 + NPPD and MB20 + EHN, respectively. MB20 generates 3%, 4.9% and 4.20% lower BTE compared with MB20 + DPPD, MB20 + NPPD and MB20 + EHN. Similar trends were found from different biodiesels, such as Calophyllum, Jatropha and soybean.27,28 The low BTE can be attributed to the low heating values and high viscosities.42 The addition of amine antioxidants to biodiesel blend fuels can achieve higher power output and lower BSFC compared with MB20.
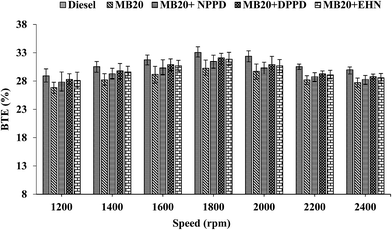 |
| Fig. 5 BTE variation at different speed for tested fuels. | |
3.4. Emission analysis
3.4.1. Effect of antioxidant addition on nitrous oxide (NOx). Engines running on biodiesel sometimes hinder the increase in nitrous oxide (NOx) emissions. Two mechanisms, namely, thermal and prompt mechanisms” dominate NOx formation in biodiesel combustion. At the combustion stage, NOx is a vital parameter that should be controlled. Many researchers reported that parameters, such as physico-chemical properties, adiabatic flame temperature, ignition delay time, biodiesel molecular structure and injection timing, are responsible for higher NOx emissions in the combustion stage.43 However, some researchers found that NOx emission increases when prompt NOx formation increases during diesel engine combustion. The reaction between molecular nitrogen and hydrocarbon radicals (CH, CH2, C2, C and CH2) is crucial in producing prompt NOx. Hence, free-radical concentration is an important element for production of HCN, N and NO. Garner and Brezinsky44 reported that during biodiesel combustion in diesel engine, the production rate of free radicals is high. As such, free radicals are regarded vital to augment NOx levels. Hence, we observed that the presence of 1000 ppm DPPD, NPPD and EHN antioxidants in biodiesel could significantly decrease NOx. Fig. 6 demonstrates variation in NOx level with speed. NOx levels linearly increase throughout the experiment. Therefore, pure biodiesel blends (MB20) clearly produce higher NOx contents compared with other blends, and the maximum NOx amount is generated by MB20 blend. By adding antioxidants (DPPD, NPPD and EHN) to MB20, NOx emission comparatively decreases. The average NOx emissions are 609.57, 678.5, 638.49, 618.5 and 628.51 ppm for B0, MB20, MB20 + NPPD, MB20 + DPPD and MB 20 + EHN, respectively. Moreover, the average increase in NOx emission was 11.31%, 4.74%, 1.46% and 3.10% compared with that of B0. By decreasing the chain length and increasing unsaturation, NOx emission increases.45,46 Addition of 1000 ppm NPPD, DPPD and EHN to MB20 significantly affects NOx, and the mean reduction values of NOx are 5.9%, 8.8% and 7.30%, respectively, compared with MB20. Thus, the addition of antioxidant clearly decreases NOx levels. The important reason underlying the NOx emissions for the fuel–antioxidant mixtures is the reaction with aromatic amines and the formation of peroxyl free radicals. The reaction between p-phenylenediamines and peroxyl free radicals to form primary amine radicals because of high reactivity of amine radicals and produce benzoquinonediimine as well as nitrooxyl radicals. The outcome of these reactions can efficiently trap free radicals.27
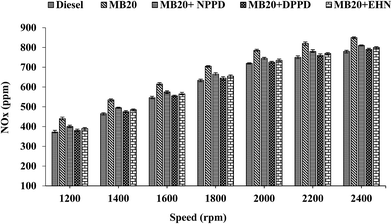 |
| Fig. 6 NOx variation at different speed for tested fuels. | |
3.4.2. Effect of antioxidant addition on HC content. Parameters, such as fuel properties, operating condition and characterisation of fuel spray, are responsible for HC emission.47,48 Fig. 7 demonstrates the fluctuation of HC emission with varied speed for all tested blends. HC emission gradually decreases with increasing speed. The maximum and minimum HC emissions are 1200 and 2400 rpm, respectively. Notably, the mean reduction values of HC emissions are 21.06%, 12.75%, 16.90% and 14.83 for MB20, MB20 + NPPD and MB20 + DPPD, respectively, compared with that for diesel. The average increases in HC in biodiesel added with NPPD, DPPD and EHN are 10.52%, 5.26% and 7.89%, respectively, compared with MB20. Previous studies on Calophyllum, Jatropha and neem biodiesel reported that HC emissions increase with addition of antioxidants.49–51 This increase is due to the reduction in oxidative free-radical formation. For proper HC conversion, higher oxygen content must be adjusted with higher CN. High CN contributes to earlier combustion with several conditions, including post-flame oxidation and larger flame speed.28 HC levels decrease remarkably when borne oxygen enlarges the unburned proportion of oxidised HC at the fuel-rich zone.34 The Fig. 7 shows that HC emission decreases throughout the speed range compared with pure diesel, which increases slightly than MB20, upon antioxidant addition.
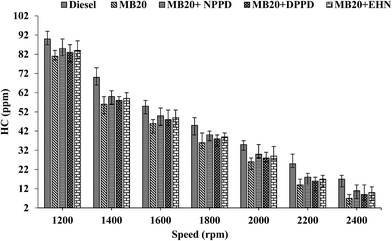 |
| Fig. 7 HC variation at different speed for tested fuels. | |
3.4.3. Effect of antioxidant addition on carbon monoxide levels. During diesel engine combustion, CO is formed, whereas air supply is insufficient at low flame temperatures. Fig. 8 displays variation in CO emission with different speeds for all the tested fuels with and without antioxidant in a single-cylinder diesel engine under the full throttle condition. Accordingly, CO emissions decrease adequately in all of the blends compared with pure diesel. The maximum CO emission was found in pure diesel. The mean decreases in CO emission are 27.1%, 13.16%, 23.43% and 20.75% for MB20, MB20 + NPPD, MB20 + DPPD and MB20 + EHN, respectively, compared with pure diesel. Diesel possesses higher CN and oxygen content than the blends; hence, diesel generates higher CO emissions compared with the other blends. A short ignition period is attained because of higher CN, thereby providing improved engine combustion when the oxygen content of biodiesel reacts. For more efficient, high-temperature, proper combustion, high oxygen content is necessary. However, addition of 1000 ppm DPPD, NPPD and EHN antioxidants to biodiesel adequately enhances CO emission. Average increases in CO upon the addition of AO (NPPD, DPPD and EHN) are 18.96%, 4.89% and 8.56% compared with that of MB20, respectively. The amount of CO emission remains less upon the addition of antioxidants in biodiesel compared with that in pure diesel. Increase in CO emission after antioxidant addition in biodiesel may be attributed to the fact that adding antioxidant can reduce the capability for CO oxidation. When oxidation occurs, hydrogen peroxide (H2O2) and peroxyl (HO2) are enormously generated. However, during combustion, these radicals are converted again into hydroxyl (OH) by absorbing heat from the combustion chamber. For this reason, CO is converted into CO2.28,34,52 When NPPD, DPPD and EHN antioxidants are added to Moringa biodiesel, HO2 and H2O2 levels decrease and negatively affect OH and CO oxidation.
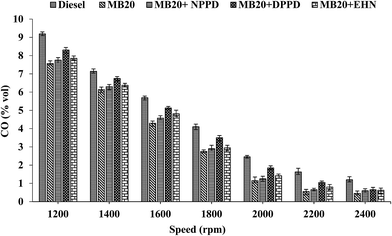 |
| Fig. 8 CO variation at different speed for tested fuel. | |
3.4.4. Effect of antioxidant addition on smoke opacity. Fig. 9 demonstrates variation in smoke opacity of the tested fuel blends at different speeds. The average smoke intensity values for diesel, MB20, MB20 NPPD, MB20 DPPD and MB20 EHN are 16.44, 14.78, 13.18, 12.88 and 12.73 HSU, respectively. Notably, MB20, MB20 NPPD, MB20 DPPD and MB20 EHN exhibit reduced normal smoke opacity by 10.09%, 19.82%, 21.65% and 22.56%, respectively, compared with pure diesel. By adding antioxidants (NPPD, DPPD and EHN), the average reduction values in smoke intensity are 10.82%, 12.85% and 25.10%, respectively, compared with MB20. Similarly, low smoke intensity can be clarified by reduced probability of abundant zone area for high local fuel–air proportion in the activated of fuel borne oxygen and oxidation of residue cores at the time of fuel ignition.31,32 Increase in smoke content could be due to reduction in oxygen availability, increase in C–C bonds and increase in aromatic content as a result of antioxidant addition to fuels. This finding is similar to those recommended in other studies.29
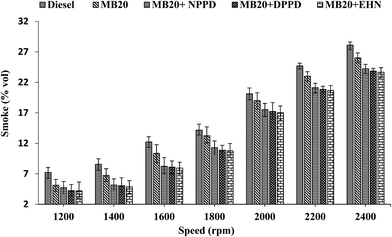 |
| Fig. 9 Smoke intensity variation at different speed for tested fuels. | |
4. Conclusion
In this study, the effects of antioxidant addition (DPPD, NPPD and EHN) on oxidation stability, engine emission and performance of single-cylinder diesel engines fuelled with Moringa biodiesel blends were investigated. The results demonstrated that the addition of antioxidant significantly increases oxidation stability and reduces NOx emission. The following conclusions were established.
(1) DPPD is the most effective antioxidant for oxidation stability in all the tested concentrations of antioxidants tested. Compared with two other antioxidants (NPPD and EHN), DPPD exhibits higher oxidation stability when added to MB20. Antioxidant additives can decrease the calorific value but enhance the kinematic viscosity, density, flash point and oxidation stability of the blends. The blends possess high oxidation stability and therefore can be stored safely.
(2) Addition of antioxidants increases the density and CN of the tested oils. The power output increases by 2.82–5.49% to levels higher than that of the untreated blend (MB20). Antioxidant addition also reduced BSFC by 2.68–4.4% but increases BTE (3–3.42%) relative to those of MB20.
(3) Antioxidant additives (DPPD, NPPD and EHN) combined with MB20 significantly reduces NOx emissions by 5.9–8.80% and smoke opacity by 10.82–25.10%. Meanwhile, the antioxidant–MB20 combination increases CO emissions by 4.89–18.96% and HC emissions by 5.26–10.52% compared with the untreated blend (MB20). However, the increment of HC and CO remains lower in antioxidant-treated MB20 compared with that in pure diesel.
Abbreviations
ASTM | American society for testing and materials |
AO | Antioxidant |
AR | Analytical reagent |
BP | Brake power |
BSFC | Brake specific fuel consumption |
BTE | Brake thermal efficiency |
BHT | 2,6-di-tert-Butyl-4-methylphenol |
BHA | 2(3)-tert-Butyl-4-methoxyphenol |
CAS No | Chemical abstracts service registry number |
CMOO | Crude Moringa oleifera oil |
CO | Carbon monoxide |
DPPD | N,N′-Diphenyl-1,4-phenylenediamine |
EHN | 2-Ethylhexyl nitrate |
FAC | Fatty acid composition |
FFA | Free fatty acid |
HC | Hydrocarbon |
MOME | Moringa oleifera methyl ester |
NOx | Oxides of nitrogen |
NPPD | N-Phenyl-1,4-phenylenediamine |
PM | Particulate matter |
PG | Propyl 3,4,5-trihydroxybenzoate (propyl gallate) |
PY | Benzene-1,2,3-triol (pyrogallol) |
TBHQ | 2-tert-Butylbenzene-1,4-diol (tert-butyl hydroquinone) |
Acknowledgements
The authors would like to thank University of Malaya for financial support through High Impact Research grant titled: “Development of alternative and renewable energy carrier (DAREC)” UM.C/HIR/MOHE/ENG/60.
References
- M. Mofijur, A. Atabani, H. Masjuki, M. Kalam and B. Masum, Renewable Sustainable Energy Rev., 2013, 23, 391–404 CrossRef CAS.
- G. Knothe, L. F. Razon and F. T. Bacani, Ind. Crops Prod., 2013, 49, 568–572 CrossRef CAS.
- M. Mofijur, H. Masjuki, M. Kalam, A. Atabani, M. Shahabuddin, S. Palash and M. Hazrat, Renewable Sustainable Energy Rev., 2013, 28, 441–455 CrossRef CAS.
- F. Maqbool, S. Mostafalou, H. Bahadar and M. Abdollahi, Life Sci., 2015 DOI:10.1016/j.lfs.2015.10.022.
- I. A. Khalek, M. G. Blanks, P. M. Merritt and B. Zielinska, J. Air Waste Manage. Assoc., 2015, 65, 987–1001 CAS.
- J. Storey, S. Curran, A. Dempsey, S. Lewis, N. R. Walker, R. Reitz and C. Wright, Emission Control Science and Technology, 2015, 1, 64–79 CrossRef.
- H. K. Imdadul, H. H. Masjuki, M. A. Kalam, N. W. M. Zulkifli, A. Alabdulkarem, M. M. Rashed, Y. H. Teoh and H. G. How, Energy Convers. Manage., 2016, 111, 174–185 CrossRef CAS.
- M. Mofijur, H. Masjuki, M. Kalam, M. Rasul, A. Atabani, M. Hazrat and H. Mahmudul, Procedia Eng., 2015, 105, 665–669 CrossRef CAS.
- M. Salaheldeen, M. Aroua, A. Mariod, S. F. Cheng, M. A. Abdelrahman and A. Atabani, Energy Convers. Manage., 2015, 92, 535–542 CrossRef CAS.
- D. M. Fernandes, R. M. Sousa, A. de Oliveira, S. A. Morais, E. M. Richter and R. A. Muñoz, Fuel, 2015, 146, 75–80 CrossRef CAS.
- S. Dollah, S. Abdulkarim, S. Ahmad, A. Khoramnia and H. Ghazali, Grasas Aceites, 2015, 66(2) DOI:10.3989/gya.0695141.
- A. Azad, M. Rasul, M. Khan, S. C. Sharma and R. Islam, Procedia Eng., 2015, 105, 601–606 CrossRef CAS.
- S. Zullaikah, I. Saputra, G. Prihandini and M. Rachimoellah, IPTEK, 2015, 1, 571–574 Search PubMed.
- M. Rashed, M. Kalam, H. Masjuki, M. Mofijur, M. Rasul and N. Zulkifli, Ind. Crops Prod., 2016, 79, 70–76 CrossRef CAS.
- M. Mofijur, H. Masjuki, M. Kalam, A. Atabani, I. R. Fattah and H. Mobarak, Ind. Crops Prod., 2014, 53, 78–84 CrossRef CAS.
- M. Serrano, A. Bouaid, M. Martínez and J. Aracil, Fuel, 2013, 113, 50–58 CrossRef CAS.
- A. Obadiah, R. Kannan, A. Ramasubbu and S. V. Kumar, Fuel Process. Technol., 2012, 99, 56–63 CrossRef CAS.
- M. Rashed, M. Kalam, H. Masjuki, H. Rashedul, A. Ashraful, I. Shancita and A. Ruhul, RSC Adv., 2015, 5, 36240–36261 RSC.
- H. Sulistyo, M. F. Almeida and J. M. Dias, Fuel Process. Technol., 2015, 132, 133–138 CrossRef.
- F. Shahidi, P. Janitha and P. Wanasundara, Crit.
Rev. Food Sci. Nutr., 1992, 32, 67–103 CrossRef CAS PubMed.
- S.-K. Loh, S.-M. Chew and Y.-M. Choo, J. Am. Oil Chem. Soc., 2006, 83, 947–952 CrossRef CAS.
- A. Gatial, J. Polovková and M. Breza, Acta Chim. Slovaca, 2008, 1, 72–84 Search PubMed.
- E. T. Denisov and I. B. Afanas' ev, Oxidation and antioxidants in organic chemistry and biology, CRC press, 2005 Search PubMed.
- G. Karavalakis, S. Stournas and D. Karonis, Fuel, 2010, 89, 2483–2489 CrossRef CAS.
- S. Jain and M. Sharma, Fuel, 2011, 90, 3014–3020 CrossRef CAS.
- E. İleri and G. Koçar, Energy Convers. Manage., 2013, 76, 145–154 CrossRef.
- K. Varatharajan and M. Cheralathan, Fuel Process. Technol., 2013, 106, 526–532 CrossRef CAS.
- S. Palash, M. Kalam, H. Masjuki, M. Arbab, B. Masum and A. Sanjid, Energy Convers. Manage., 2014, 77, 577–585 CrossRef CAS.
- K. Varatharajan, M. Cheralathan and R. Velraj, Fuel, 2011, 90, 2721–2725 CrossRef CAS.
- K. Ryu, Bioresour. Technol., 2010, 101, S78–S82 CrossRef CAS PubMed.
- I. M. Rizwanul Fattah, H. H. Masjuki, M. A. Kalam, M. A. Wakil, A. M. Ashraful and S. A. Shahir, Energy Convers. Manage., 2014, 83, 232–240 CrossRef CAS.
- I. M. Rizwanul Fattah, M. H. Hassan, M. A. Kalam, A. E. Atabani and M. J. Abedin, J. Cleaner Prod., 2014, 79, 82–90 CrossRef CAS.
- C. Haşimoğlu, M. Ciniviz, İ. Özsert, Y. İçingür, A. Parlak and M. S. Salman, Renewable Energy, 2008, 33, 1709–1715 CrossRef.
- I. M. Rizwanul Fattah, H. H. Masjuki, M. A. Kalam, M. Mofijur and M. J. Abedin, Energy Convers. Manage., 2014, 79, 265–272 CrossRef CAS.
- H. Aydin and H. Bayindir, Renewable Energy, 2010, 35, 588–592 CrossRef CAS.
- T. T. Kivevele, L. Kristóf, Á. Bereczky and M. M. Mbarawa, Fuel, 2011, 90, 2782–2789 CrossRef CAS.
- M. Gumus and S. Kasifoglu, Biomass Bioenergy, 2010, 34, 134–139 CrossRef CAS.
- H. Rashedul, H. Masjuki, M. Kalam, Y. Teoh, H. How and I. R. Fattah, Energy Convers. Manage., 2015, 106, 849–858 CrossRef CAS.
- M. I. Al-Widyan and G. Tashtoush, Fuel Process. Technol., 2002, 76, 91–103 CrossRef CAS.
- M. Gürü, A. Koca, Ö. Can, C. Çınar and F. Şahin, Renewable Energy, 2010, 35, 637–643 CrossRef.
- H. Hazar and H. Aydin, Appl. Energy, 2010, 87, 786–790 CrossRef CAS.
- P. Devan and N. Mahalakshmi, Fuel Process. Technol., 2009, 90, 513–519 CrossRef CAS.
- H. Imdadul, H. Masjuki, M. Kalam, N. Zulkifli, M. Rashed, H. Rashedul, I. Monirul and M. Mosarof, RSC Adv., 2015, 5, 67541–67567 RSC.
- S. Garner and K. Brezinsky, Combust. Flame, 2011, 158, 2289–2301 CrossRef CAS.
- G. Knothe, Fuel Process. Technol., 2005, 86, 1059–1070 CrossRef CAS.
- S. K. Hoekman and C. Robbins, Fuel Process. Technol., 2012, 96, 237–249 CrossRef CAS.
- O. S. Valente, V. M. D. Pasa, C. R. P. Belchior and J. R. Sodré, Sci. Total Environ., 2012, 431, 57–61 CrossRef CAS PubMed.
- A. Sanjid, H. Masjuki, M. Kalam, S. A. Rahman, M. Abedin and I. R. Fattah, RSC Adv., 2015, 5, 13246–13255 RSC.
- H. K. Rashedul, H. H. Masjuki, M. A. Kalam, Y. H. Teoh, H. G. How and I. M. Rizwanul Fattah, Energy Convers. Manage., 2015, 106, 849–858 CrossRef CAS.
- A. Prabu and R. Anand, Front Energ., 2015, 1–8 Search PubMed.
- G. Balaji and M. Cheralathan, Renewable Energy, 2015, 74, 910–916 CrossRef CAS.
- H. Rashedul, H. Masjuki, M. Kalam, A. Ashraful, M. Rashed, I. Sanchita and T. Shaon, RSC Adv., 2014, 4, 64791–64797 RSC.
|
This journal is © The Royal Society of Chemistry 2016 |