DOI:
10.1039/C5RA25559G
(Paper)
RSC Adv., 2016,
6, 11773-11785
DNA templated self-assembly of gold nanoparticle clusters in the colorimetric detection of plant viral DNA using a gold nanoparticle conjugated bifunctional oligonucleotide probe
Received
1st December 2015
, Accepted 9th January 2016
First published on 13th January 2016
Abstract
The DNA templated self-assembly of gold nanoparticles clustered in different configurations (n = 2–∞) was investigated in the colorimetric detection of ToLCNDV DNA using a gold nanoparticle conjugated bifunctional oligonucleotide probe. The AuNP-bifunctional oligonucleotide probe conjugate was prepared using citrate capped AuNPs (∼19 nm) and virus specific ssoligo probes 1 and 2. Each conjugate consisted of ∼105 ssoligo probes 1 and 2, specific for the forward and reverse strands of the PCR amplified ToLCNDV dsDNA target. The intensity of the UV-visible absorbance spectra of AuNP-bifunctional oligonucleotide probes decreased gradually after hybridization with increasing ratios of dsDNA targets (0.0–1.0). Hybridized AuNP-bifunctional oligonucleotide probes showed gradual resistance against salt induced aggregation with increasing concentrations of dsDNA target, up to the ratio of 1.0. The color of solution remained red even after hybridization of the AuNP-bifunctional oligonucleotide probe with the dsDNA target. These hybridized AuNP-bifunctional oligonucleotide probes were found as clusters with different configurations (n = 2–11) and defined interparticle distances (1.3–2.1 nm). This target DNA guided the self-assembly of the AuNP-bifunctional oligonucleotide probes which is the reason for the different optical absorbance properties of hybridized solutions before and after the salt treatment. An exciting finding of this investigation is that the AuNP-bifunctional oligonucleotide probes were anchored on the center core particle, tending to form a AuNP cluster that incorporated from 1–6 Au-NP probes and extended to 8 and 10 with an increasing size of core particle diameter. The assembly of three dimensional DNA templated AuNP clusters in flower and pyramid shapes was possible with a dsDNA target and a AuNP-bifunctional oligonucleotide probe but not with a AuNP-monofunctional oligonucleotide probe. The limit of detection of dsDNA target in this bifunctional nanoprobe assay was ∼7.2 ng. Also, this AuNP-bifunctional oligonucleotide probe could reduce the concentration of target DNA required for colorimetric detection by half, as it could recognise both strands in the dsDNA target simultaneously. The proof of this concept will be used for further development of ultra sensitive nanoassay methods and will also be applicable for materials science applications.
Introduction
Nanotechnology emphasizes materials with 10−9 meter dimensions, and involves biotechnology, materials science and engineering applications.1 In recent decades, metal nanoparticles have been widely used in the fields of biosensors,2,3 nanoassembly,4,5 and nanoelectronics.6,7 In particular, gold nanoparticles (AuNPs) are extensively used in molecular diagnosis8–10 because of their interesting physicochemical properties.11–13 The discovery of nanoparticle conjugated oligonucleotide/DNA (AuNPs-DNA) was a significant development in the field of clinical diagnosis and has been applied to the colorimetric detection of target DNA,8–10,14 single base mismatches,15–18 deletions and insertions,19 E.coli genomic DNA,20 White spot syndrome virus21 detection and discrimination of Mycobacterium tuberculosis.22–24 The AuNPs act as an optical sensing element and the oligonucleotide probes serve as a recognition unit. Recently, AuNP conjugated oligonucleotide probes have been used for the detection of specific DNA sequences in PCR amplified DNA.25 The hybridization of AuNP conjugated oligonucleotide probes with their target DNA leads to the self-assembly of plasmonic nanoparticles in defined three dimensional (3D) structures and/or configurations. This DNA templated self-assembly of AuNPs has been used to prepare new types of biomaterials. Also, it is a new area of research in the field of bio-nanotechnology because it merges the research fields of DNA and metal colloid chemistries.8,26 DNA mediated self-assembled binary nanoparticle network materials were prepared with defined geometry using two different thiol modified oligonucleotide probes functionalized with two different sizes of AuNPs and DNA linkers.27 Recently, the triangular and square cyclic assembly of AuNPs was achieved using DNA as a template.28 Different sizes of nanomaterials in self-assembled nanonetworks, possess different surface plasmon resonance (SPR) and surface enhanced Raman spectroscopy (SERS) signal characteristics which have been used in the development of biosensors.29,30 So far two different sizes of AuNPs and oligos with mono-functionality have been used to prepare DNA templated assembled nanoclusters for the detection of target DNA. Monofunctional AuNP oligonucleotide probes are used to recognize a target sequence from any one strand of a dsDNA target and the second strand is left free. In order to detect the second strand of the dsDNA target, another monofunctional AuNP-oligonucleotide probe conjugate is required. To address this, we hypothesized that a AuNP-oligonucleotide probe conjugate with bifunctionality could be used for the detection of both strands of dsDNA at the same time because the selected probes would be able to hybridize both strands of the dsDNA target and would tend to form defined geometry nanoclusters. This could reduce the concentration of target DNA and the types of nanomaterials required for the development of detection methods because the AuNP conjugated bifunctional oligonucleotide probe incorporates two different oligo probes which are specific for both strands of a dsDNA target.
Moreover, the application of nanotechnology in agriculture is still at an initial stage. Specifically, nanosensor based detection of plant pathogens has not yet been reported. Plant viruses are major causative agents for many diseases in commercial crops as well as other plants. For example, white-fly transmitted single strand circular DNA Tomato leaf curl virus (ToLCV) belongs to the family of Geminivirdeae and the genus of Begomovirus, and causes severe leaf curl disease in tomato plants throughout the world including in India where it leads to 80–100% yield loss.31,32 Existing conventional methods like PCR and immunoassay have their own disadvantages.2,25,33,34 Therefore, the development of a cost effective detection method is to be considered as very important for the early detection of these viruses to control the disease. Herein, we demonstrate our hypothesis of DNA templated 3D cluster assembly of AuNP conjugated bifunctional oligonucleotide probes in the sequence specific colorimetric detection of Tomato leaf curl New Delhi virus (ToLCNDV) dsDNA targets (Fig. 1).
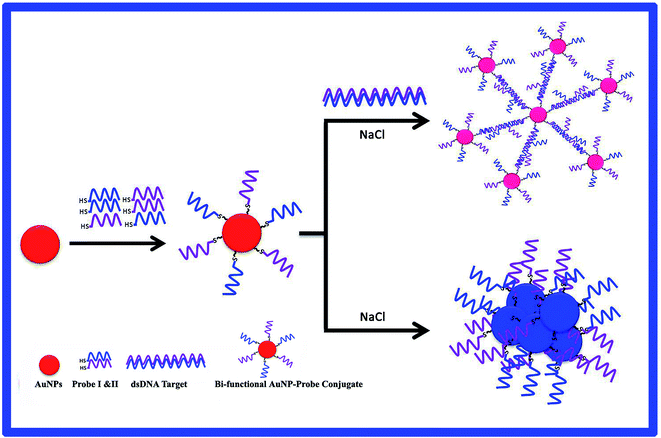 |
| Fig. 1 Schematic representation of the colorimetric detection of ToLCNDV DNA using AuNP conjugated bifunctional oligonucleotide probes and the self-assembly of gold clusters. | |
Materials and methods
Materials
Trisodium citrate, hydrogen tetrachloroaurate trihydrate (HAuCl4⋅3H2O), disodium hydrogen phosphate (Na2HPO4), monosodium dihydrogen phosphate (NaH2PO4), and sodium chloride (NaCl) were obtained from Loba Chemie (Mumbai, India). Ethylene diamine tetraacetic acid (EDTA) and agarose were purchased from HiMedia Laboratories Pvt. Ltd. (Mumbai, India). The primers encoding the coat protein (CP) gene (ToLCVFP/ToLCVRP) were synthesized commercially (Eurofins MWG Operon, India Pvt. Ltd., Bangalore, India). Red dye PCR Master Mix was purchased from Ampliqon A/S (Odense, Denmark). Polymerase Chain Reactions (PCR) were performed in a L1996GGD Peltier Model Thermocycler purchased from Lark Innovative Fine Teknowledge Pvt. Ltd. (India). Thiol modified oligonucleotide probes were purchased from VBC-Biotech Service GmbH (Vienna, Austria).
Synthesis and characterization of citrate capped AuNPs
Citrate capped AuNPs were synthesized based on the previously described boiling method.35 In brief, a 250 mL round-bottom glass flask, a reflux condenser, and a large stir-bar were washed with aqua regia (3
:
1, Conc HCl
:
Conc HNO3 v/v) followed by thorough washing with water. The glassware was assembled on a heating mantle and a magnetic stirrer, and the stir-bar was put into the flask. 100 mL of 1 mM HAuCl4 was added to the flask and boiled to reflux (100 °C) with vigorous stirring. Then, 5 mL of 38.8 mM sodium citrate was rapidly added to the solution and boiling was continued for 20 min. The yellow color of the solution turned to purple and then to deep red which indicated the formation of AuNPs. Then, the solution was cooled to RT and filtered through a 0.45 μm nylon filter. Finally, the solution was stored at RT in a glass container in the dark. The synthesized AuNPs solution was characterized by UV-visible spectrophotometry (ShimadzuUV-1600, Japan).
The size and shape of the synthesized AuNPs were analyzed by HR-TEM (FEI Technai G2 F30 S-TWIN with a 200 kV high-resolution (UHR) pole piece). AuNP solution (2 μL) was dropped on a carbon coated copper grid and dried in air at RT. Then, the copper grid was placed in the sample holder and lattice images were recorded using HR-TEM operating at an accelerating voltage of 200 kV with a high-resolution (UHR) pole piece. The hydrodynamic diameter and size distribution of the synthesized AuNPs were analyzed using a Malvern particle size analyzer (Zetasizer Nano S). AuNP solution (2 mL) was put into a disposable cuvette and placed in a sample holder. The particle size analyzer operated at an accelerating voltage of 100 V, 25 °C, with count rate 232 kcps, and a duration of 60 s. The data were recorded by Zetasizer Ver. 620.
The concentration of the synthesized AuNPs was calculated theoretically using the particles' diameter and the molar concentration of gold salt solution.36 Assuming that NPs were spherical in shape and uniform in size, the average number of gold atoms per nanoparticle could be calculated using the following equation N = (Rcluster/Ratom)3, where Rcluster is the diameter of NPs in nanometers obtained from TEM analysis and Ratom (= 0.137 nm) is the diameter of a gold atom. Next, the number of gold atoms (Natom) was calculated in a given concentration of gold salt solution (1 dm3 or 1 L of 1 mM HAuCl4; i.e. moles of HAuCl4 = 1 x 1 x 10−3 = 1 x 10−3 mol) using the equation Natom = Moles of HAuCl4 x NA, where NA is Avogadro's number (6.022 x 1023). From the calculation of N and Natom, the total number of NPs formed in the given solution was calculated using the equation NNP = Natom/N. Hence, the final concentration of the gold colloid (CNP) was estimated by dividing NNP by Avagadro's number (NA)
:
CNP = NNP/NA in mol dm−3 (1 L).
Preparation of the AuNP conjugated bifunctional oligonucleotide probe
The synthesized AuNP solution was used for the preparation of the AuNP conjugated bifunctional oligonucleotide probe. ToLCNDV specific probe sequences were designed through the multiple sequence alignment of retrieved nucleotide sequences of different isolates of ToLCNDV from NCBI. Identical and complementary sequences were selected from two different regions for the synthesis of probes and positive controls from the results of multiple sequence alignment using BioEdit software. The selected sequences were subjected to self-complementation and dimer formation analyses through online tools. The designed probes (Forward probe 1–26 mer HS-5' -GAATTCATGTCSAAGCGWCCRGCAGA-3′ and Reverse probe 2–26 mer HS-5′-GGTACCATTCTTMACAGTWGCAGTGC-3′) were custom synthesized with a thiol functional group at the 5′ end for the preparation of conjugates.
The AuNP conjugated bifunctional oligonucleotide probe was prepared based on the earlier described method with slight modifications.37,38 In detail, 50 pM of probe 1 for the forward strand and 50 pM of probe 2 for the reverse strand were mixed with 0.1 M of phosphate-buffered saline (PBS) (10 mM of NaCl with 0.01% SDS, pH 7), the volume was made up to 1 mL with citrate capped AuNPs and the mixture was incubated at 50 °C for 24 h. After the incubation, the solution was subjected to an aging process using 1 M of NaCl (final concentration) with phosphate buffer (pH 7) for 24 h at 50 °C. Unbound probes were removed from the solution by centrifugation at 14
000 rpm for 25 min and the supernatant was replaced with 1 mL of phosphate buffer. The centrifugation process was repeated twice. The centrifugate was collected and redispersed into 0.5 mL using phosphate buffer to make a stock solution, which was used for further experiments. The amount of DNA probe immobilized on the AuNP surface was calculated by measuring the optical absorbance at 260 nm using UV-visible spectrophotometry. Moreover, the prepared AuNP-bifunctional probe was characterized by HR-TEM and DLS.
The AuNP conjugated bifunctional oligonucleotide probe was characterized by Raman spectroscopy (Raman-11; Nanophoton, Japan) after coating with a Raman probe (Rhodamine 6G - R6G).39 The conjugated solution was concentrated by centrifugation at 10
000 rpm for 15 min. The supernatant was discarded and the oily red color pellet was diluted with 500 μL of 20 μM R6G and incubated for 4 h at RT. Unbound R6G was removed by centrifugation at high speed for 15 min, and the pellet was collected and diluted with 100 μL ddH2O. The solutions were spotted on a cleaned glass slide and dried in air at RT. The samples were subjected to confocal Raman microscopy analysis by the illumination of laser light (532 nm) at 40 mW for excitation as well as imaging. Finally, scattered light spectra were recorded and images were captured using a CCD camera.
Isolation and amplification of ToLCNDV DNA
Total genomic DNA was extracted from a ToLCNDV infected tomato leaf sample based on the previously described method.40 The isolated DNA was analyzed by 0.8% agarose gel electrophoresis and quantified by UV-visible spectrophotometry. 800 bp of the CP gene fragment was amplified from the ToLCNDV DNA with geminivirus specific common primers using PCR. The reaction mixture was prepared in a total reaction volume of 50 μL by the addition of 25 μL of 2x red dye PCR Master Mix, 22.5 μL of DNase/RNase free deionized water, ToLCV specific primers (100 pM of 0.625 μL forward primer and 0.625 μL reverse primer) and 1.25 μL of template DNA. The amplification program consisted of the following steps: initial denaturation at 94 °C for 5 min; 25 cycles of denaturation at 94 °C for 1 min, annealing at 60 °C for 1 min for the CP gene (ToLCVFP/ToLCVRP), extension at 72 °C for 2 min; and a final extension at 72 °C for 5 min. Amplified PCR products were extracted from the reaction mixture by the addition of 5 μL of sodium acetate solution (pH 4.2) and 100 μL of 95% ethanol to the 50 μL PCR reaction. The mixture was vortexed gently and incubated at −20 °C for 40 min. Then the mixture was centrifuged at 10
000 rpm for 20 min. The supernatant was discarded carefully and the pellet was washed with 70% (v/v) ethanol. After washing, the pellet was air dried at RT and suspended in 50 μL of RNase/DNase free deionized water for further studies. Extracted products were electrophoresed in 1.2% (w/v) agarose gel, visualized under UV light and quantified using UV-visible spectrophotometry. This extracted DNA was used for further experiments.
Hybridization assay and gold nanocluster self-assembly
To optimize the hybridization assay, 10 μL of the AuNP-bifunctional oligonucleotide probe was mixed with different concentrations of NaCl (0–6 M) in the presence of 5 μL of hybridization buffer (10 mM phosphate buffer, 0.1 M NaCl containing 1 mM EDTA, pH 7.2), and the volume was made up to 20 μL using deionized water. The solution was mixed well and kept for 10 min at RT. Subsequently, the absorbance was measured using UV-visible spectrophotometry and the salt concentration was optimized for the hybridization assay. Different concentrations of PCR amplified dsDNA target (0.0, 0.2, 0.4, 0.6, 0.8 and 1.0 ratios v/v) were mixed with the AuNP conjugated bifunctional oligonucleotide probe and incubated initially at 95 °C for 5 min and then at 37 °C for 55 min in the presence of hybridization buffer. After the incubation, the optimized concentration of salt was added to the solution, it was kept at RT for 10 min and the color changes were observed. The optical absorbances of all the solutions were analyzed by UV-visible spectrophotometry before and after the addition of salt. After the hybridization assay, completely hybridized dsDNA (1.0 ratio) and control samples were also analyzed by HR-TEM.
Results and discussion
Synthesis and characterization of citrate capped AuNPs
AuNPs were synthesized according to the previously described method.35 In brief, the yellow color of the reaction solution (HAuCl4) gradually changed to purple and then to red after the addition of sodium citrate in boiling conditions. This indicated the formation of AuNPs as shown in Fig. 2A. The sodium citrate acts as a reducing as well as a capping agent which controls the further nucleation growth of NPs.41,42 The intense red color of the synthesized AuNPs is due to the interaction of incident light with a collective oscillation of free electrons in the particles (localized SPR).43 The synthesized AuNP solution was analyzed by UV-visible spectrophotometry. The maximum absorbance for the red colored AuNP solution was found at 520 nm with the sharp peak shown in Fig. 2B. This is mainly due to the size dependent SPR of metal nanoparticles.44,45
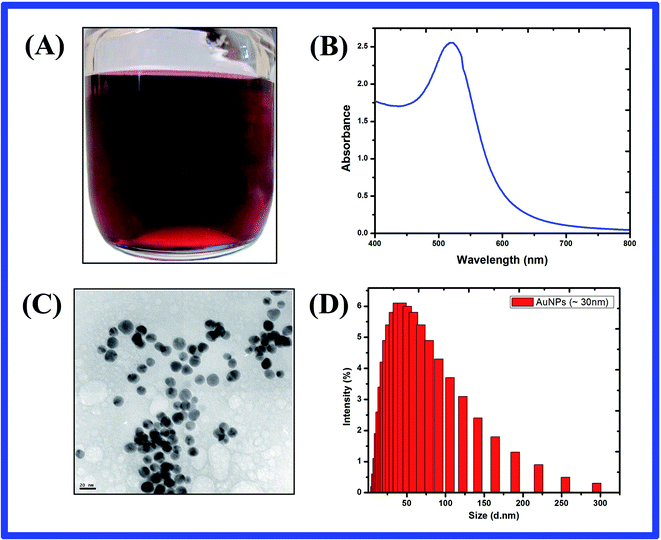 |
| Fig. 2 Synthesis and characterization of citrate capped AuNPs. (A) Deep red color of synthesized citrate capped AuNPs, (B) UV-visible spectrum of citrate capped AuNPs with an absorbance maximum at 520 nm, (C) HR-TEM image of spherical shaped AuNPs with a size of ∼19 nm, and (D) hydrodynamic diameter of citrate capped AuNPs (∼30 nm). | |
The size and shape of the synthesized AuNPs were analyzed by HR-TEM. The HR-TEM image in Fig. 2C shows monodispersed spherical AuNPs with diameters of ∼19 nm. The growth of spherical nanoparticles takes place by the reduction of the gold salt and subsequent deposition of reduced Au0 metal atoms. Initially, the reduced metal forms a cluster, and continuous deposition leads to the growth of particles in a defined shape and size, which is regulated by the capping agent.41,46,47 The hydrodynamic diameter of the synthesized citrate capped AuNPs was also revealed using the DLS method. The average hydrodynamic diameter of the synthesized AuNPs was found to be ∼30 nm as shown in Fig. 2D. The particle size as measured by the DLS method was found to be higher than that measured by the HR-TEM method. This is due to the presence of a layer of hydrogen bonds between the particles' surface and the solvent.48 The molar concentration of the synthesized citrate capped AuNPs was calculated based on the previously described method.36 Initially, the average number of gold atoms per nanoparticle was calculated using the diameter of the synthesized AuNPs (D = ∼19 nm). The number of gold atoms per nanoparticle “N” was found to be 2667418.5. From the result of N, the total number of nanoparticles (NNP) formed in a given molarity of solution (1 x 10−3 mol) of HAuCl4 was calculated (NNP = 2.26 x 1013). The final concentration of AuNPs (CNP) was estimated by NNP/Avagadro's number (NA) and was found to be CNP = ∼3.75 x 10−9 mol dm−3 (1 L) or 3.75 nmol. The AuNP concentration varies according to the diameter of the particles; as the number of gold atoms per nanoparticle increases, the concentration of AuNPs decreases.
Preparation of the AuNP conjugated bifunctional oligonucleotide probe
The AuNP conjugated bifunctional oligonucleotide probe was prepared based on Au-SH chemistry.37,38 The 5′ thiol modified oligonucleotide probes were chemisorbed and formed a thiol monolayer on the AuNP surface as depicted in Fig. 1. Chemisorption of thiol modified oligo probes 1 and 2 on the AuNP surface was enhanced by reducing the intermolecular and interparticle repulsion forces between the ssDNA oligonucleotide probes and AuNPs using PBS.
Oligo thiol attached AuNPs show strong resistance to salt induced aggregation in the aging process whereas unbound AuNPs become aggregated. The photographic images in Fig. 3A show synthesized AuNPs as a reference (a), the AuNP conjugated bifunctional oligonucleotide probe (b), and aggregated AuNPs as a control (c). The intense red color of the AuNPs in aqueous solution is due to the negative repulsion force based monodispersity and SPR.49 After the addition of salt, the complete aggregation of AuNPs was found only in solution ‘c’ and the color of this solution changed to blue. This color change was not observed in solution ‘b’ (AuNP conjugated bifunctional oligo probes) even in the presence of a high salt concentration. Particles in solution ‘b’ were found to be monodisperse and red in color. This proves that ssoligo probes 1 and 2 were attached onto the AuNP surface through Au-SH chemistry and tended to form a thiol monolayer on the NPs because thiol groups show high affinity towards noble metal surfaces, particularly gold.50,51 In addition, surface bound ssDNA oligo probes enhance interparticle electronegative repulsion forces between NPs and help to maintain an appropriate interparticle distance between the conjugates, preventing aggregation.52,53 The optical absorbance properties of the AuNP conjugated bifunctional oligonucleotide probes were analyzed by UV-visible spectrophotometry with control and reference. Fig. 3B shows UV-visible absorbance spectra ‘a’, ‘b’ and ‘c’ for AuNPs (Ref; a), the AuNP conjugated bifunctional oligonucleotide probe (b) and AuNPs after the aging process (control; c), respectively. The AuNPs show different light absorbance properties with and without the salt treatment. In the absence of salt, AuNPs show a sharp peak at 520 nm with higher intensity (Fig. 3B spectrum ‘a’). In the presence of salt, AuNPs show a broadening peak towards the longer wavelength region (above 600 nm) with a small peak at 520 nm (Fig. 3B spectrum ‘c’). This peak broadening could be due to the aggregation, color changes and SPR band shift of AuNPs.54 The absorbance spectrum of the AuNP conjugated bifunctional oligonuclotide probe shows the SPR band at 524 nm even after the salt treatment (Fig. 3B spectrum ‘b’). A red shift of the AuNPs was found from 520 nm to 524 nm after the conjugation of the AuNPs with thiol modified oligonucleotide probes. This could be due to the change of refractive index and the formation of dielectric layers around the AuNPs.55 Further, it implies the successful chemisorption of ssDNA probes onto the surface of the AuNPs.53,56,57 This also significantly increases the electronegativity and stability of the AuNP conjugated probe.58
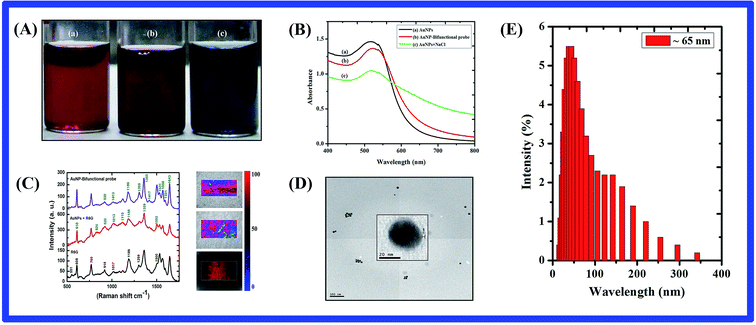 |
| Fig. 3 Preparation and characterization of the AuNP conjugated bifunctional oligonucleotide probe. (A) Photographic image of (a) AuNPs without NaCl treatment, (b) AuNP-bifunctional oligonucleotide probe after salt treatment and (c) aggregated AuNPs after salt treatment. (B) Corresponding UV-visible spectra (a-c) for AuNPs, the AuNP-bifunctional oligonucleotide probe, and aggregated AuNPs. (C) Surface enhanced Raman spectra of R6G, R6G with the AuNP-bifunctional oligonucleotide probe and R6G with AuNPs. The right hand panel shows corresponding Raman images with a scale bar. (D) HRTEM image of AuNP conjugated bifunctional oligonucleotide probes. (E) Average hydrodynamic diameter of the AuNP-bifunctional oligonucleotide probes (∼65 nm). | |
Confocal Raman microscopy was used to analyze the surface of the AuNP-bifunctional oligonucleotide probe using Rhodamine 6G (R6G) as the Raman probe. SERS spectra of R6G, R6G with AuNPs, and R6G with the AuNP conjugated bifunctional oligonucleotide probe are shown in Fig. 3C. The Raman probe R6G showed major peaks at 608, 769, 1186, 1357, 1533, 1565 and 1645 cm−1 which are the characteristic peaks of C–C–C, C–H, C–C, C–N and ring plane assignments of R6G.39 The Raman probe bound AuNPs showed enhanced intensity and slight shifts of the SERS peaks (±2–6 cm−1) at 608 → 610 cm−1, 918 → 920 cm−1, 1017 → 1013 cm−1, 1121 → 1119 cm−1, 1186 → 1188 cm−1, 1299 → 1305 cm−1, 1357 → 1359 cm−1, 1413 → 1417 cm−1, 1500 → 1502 cm−1, 1533 → 1531 cm−1, 1593 → 1591 cm−1, and a new peak appeared at 824 cm−1 (Fig. 3C, AuNPs + R6G spectra). This could be due to the adsorption of R6G+ molecules on the AuNP surface through electrostatic forces. The enhancement of SERS bands from 500–2000 cm−1 may be a result of parallel to inclined orientational changes which can enhance Raman bands from vibrational modes that lie perpendicular to the xanthine's plane mode. This suggests that R6G molecules are found in close proximity to Au metal surfaces, locating 0–4 nm from the substrate surface or the electromagnetic field. These interactions between R6G and the AuNP surfaces cause greater scattering which may lead to changes in the Raman spectra.59–61 Similarly, the spectrum of the AuNP-bifunctional oligonucleotide probe with R6G showed SERS band shifts to 1184, 1355, 1529, 1568 and 1643 cm−1 (Fig. 3C, AuNP-bifunctional oligonucleotide probe). The intensity peaks for the R6G bound AuNP conjugated bifunctional oligonucleotide probe is lower than those for the AuNPs with R6G and higher than those of R6G except for the R6G peaks at 551 and 918 cm−1. Thus the intensity of SERS peaks was gradually increased in the order: R6G < AuNP-bifunctional oligonucleotide probe conjugate + R6G < AuNPs + R6G. The number of R6G molecules bound on the surface of the AuNPs could be the reason for the increase in the intensity of SERS peaks in the above order. The SERS peak intensities at 1500 and 1645 cm−1 are proportional to the number of Raman probes bound on the AuNP surface. The number of R6G molecules bound on the unmodified AuNP surface was found to be very high. This could be a reason for the increase in the peak intensities of the AuNPs + R6G spectrum when compared with the R6G spectrum. SERS peak shifts occurred due to the weaker interactions of R6G on the AuNP surface in an inclined plane. The decreased intensity of SERS peaks of the AuNP conjugated bifunctional oligonucleotide probes with R6G might be due to the presence of a thiol monolayer and nonspecific interactions of oligonucleotide probes on the AuNP surface in a flat plane orientation. This might have interfered with the interaction of R6G molecules with the AuNP surface. The shifts of the SERS peaks and decreased intensity of the AuNP conjugated bifunctional oligonucleotide probe with R6G is due to the chemisorption of ssoligo probes.62,63 The corresponding Raman confocal images of R6G, R6G with AuNPs, and R6G with AuNP conjugated bifunctional oligonucleotide probes are shown in the right hand panel of Fig. 3C with a scale bar. The intense red, pink and blue colors indicate the presence of R6G, AuNPs and clean surface, respectively.
The AuNP conjugated bifunctional oligonucleotide probes were also investigated by HR-TEM. Fig. 3D shows an HR-TEM image of AuNP conjugated bifunctional oligonucleotide probes in two dimensional (2D) arrangements. These 2D arrangements of AuNP-bifunctional oligonucleotide probe conjugates were mainly due to the weaker interactions between the conjugates. The right hand panel of Fig. 3C shows the presence of a thin outer layer of 1.6 nm around the nanoparticles. It also proves that the presence of surface bound oligonucleotide probes favours the 2D assembly of NPs.8,64 Dispersibility and aggregation of AuNP conjugated bifunctional oligonucleotide probes in a high salt concentration were analyzed by measuring the hydrodynamic diameters of particles in aqueous medium, along with control and reference samples, using DLS as shown in Fig. 3E. The average hydrodynamic diameter of AuNP conjugated bifunctional oligonucleotide probes after the salt treatment was found to be ∼65 nm. This clearly shows that AuNP conjugated bifunctional oligonucleotide probes resist the salt induced aggregation. This could be due to the functionalization of the probe which increases the electronegativity and stability of AuNPs in high salt concentration. However, the sizes of the AuNP conjugated bifunctional oligonucleotide probes were two fold higher than the size of the synthesized AuNPs. This could be due to the weaker interactions between AuNP-bifunctional oligonucleotide probe conjugates.
Quantification of surface bound oligonucleotide probes on AuNPs
The number of oligonucleotide probes chemisorbed on the surface of an AuNP (diameter ∼19 nm) was calculated with the aid of UV-visible spectroscopy. AuNP conjugated oligonucleotide probes were treated with β-mercaptoethanol and salt. β-mercaptoethanol is a strong reducing agent which breaks Au−SH bonds between AuNPs and ssoligos. This causes the AuNPs to aggregate and ssoligos to be released to the solution. The optical absorbance at 260 nm of the collected supernatant was measured and it was found to be 1.62 OD. The total weight of ssoligo bound to the AuNP surfaces was calculated to be 32.4 μg (ssoligo: 1 OD260 corresponds to 20 μg). Using this weight, the molar concentration of immobilized oligomers was calculated to be 3.96 x 10−9 M and the number of ssoligo probes present in the given concentration of ssoligo stock solution was calculated to be 23.8 x 1014. Using the molar concentration of AuNPs and the number of AuNPs present in the solution, the number of oligonucleotide probes immobilized on each AuNP was calculated to be ∼105. The number of ssoligo probes immobilized on a AuNP depends on the length of the oligomer, and the size and surface area of the particles. The number of ssoligo probes bound on a NP surface increases with the increasing size of the nanoparticles. Similarly, short oligomers can be conjugated faster and with higher numbers than longer length oligomers. This is due to the inter-strand steric interference between ssoligos and the self-coiled secondary structures of long ssoligos.65–67
Hybridization assay and DNA guided gold nanoparticle cluster self-assembly
The salt concentration was optimized for the complete aggregation of AuNP conjugated bifunctional oligonucleotide probes using different concentrations of NaCl (0−6 M). The photographic image in Fig. 4A shows that the color of AuNP conjugated bifunctional oligonucleotide probes changed from red to purple to blue after the addition of NaCl. The color of the AuNP conjugated bifunctional oligonucleotide probes was purple at 2 and 3 M NaCl and had completely changed to blue at 4 M NaCl and above. The complete aggregation and black precipitation of AuNPs was found at 6 M. From these results, 4 M NaCl was taken as the optimized salt concentration for further hybridization assays, which is approximately four times higher than the NaCl concentration required for the complete aggregation of unfunctionalized AuNPs. The corresponding UV-visible absorbance spectra of AuNP conjugated bifunctional oligonucleotide probes treated with different concentrations of salt (0−6 M) are shown in Fig. 4B. The absorbance maxima of AuNP-bifunctional oligonucleotide probes was found at 525 nm, and gradually decreased in intensity with an SPR band shift towards longer wavelength (above 600 nm) when increasing the concentration of NaCl from 1 M to 6 M. A significant SPR shift occurred from 4 M to 6 M NaCl which indicated the complete aggregation of the AuNP-bifunctional oligonucleotide probe conjugate. This color transition of the AuNP-bifunctional oligonucleotide probe conjugates was mainly due to the salt induced aggregation.68
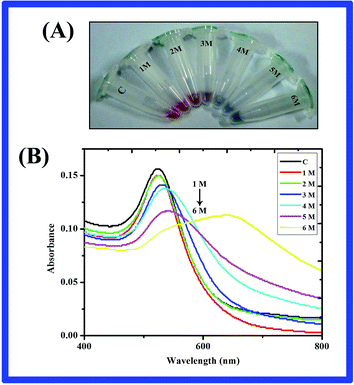 |
| Fig. 4 Optimization of salt concentration for the complete aggregation of AuNP-bifunctional oligonucleotide probes. (A) Photographic image showing the aggregation of AuNP-bifunctional oligonucleotide probes at different salt concentrations (C, 1-6 M) and (B) UV-visible spectra of AuNP-bifunctional oligonucleotide probes with different concentrations of salt (C, 1-6 M). Sample C is the control with no salt added. | |
Double stranded target DNA was amplified from the ToLCNDV infected positive sample using ToLNDV CP gene specific primers (ToLCVFP/ToLCVRP) and analyzed by 1.2% agarose gel electrophoresis. Amplified PCR product was extracted, quantified spectrometrically (180.4 ng μL−1), and 100 times diluted PCR DNA was used for further study (data not shown). The AuNP-bifunctional oligonucleotide probe conjugate was subjected to hybridization with the PCR amplified dsDNA target from the ToLCNDV CP gene in different ratios (0.2, 0.4, 0.6, 0.8 and 1.0 v/v). Fig. 5A shows AuNP-bifunctional oligonucleotide probes hybridized with dsDNA target in different ratios. There were no significant color changes; all were observed to be red in color. However, the corresponding UV-visible spectra showed gradual decreases in the intensity of absorbance at 525 nm with increasing concentrations of dsDNA targets from a ratio of 0.2 to 1.0 (Fig. 5B, spectra ‘a-e’). This is due to the distance dependent SPR of the hybridized AuNP-bifunctional oligonucleotide probes with their dsDNA target.
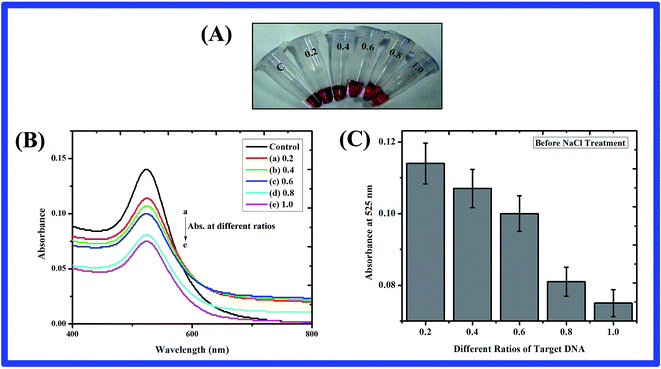 |
| Fig. 5 Hybridization of AuNP-bifunctional oligonucleotide probes with dsDNA targets at different ratios (0.0-1.0). (A) Photographic image of hybridized AuNP-bifunctional oligonucleotide probes with dsDNA targets, (B) corresponding UV-visible absorbance spectra before salt treatment and (C) bar graph of absorbance at 525 nm. Sample C is the control with no dsDNA target added. | |
The control sample and the AuNP-bifunctional oligonucleotide probes with a 0.2 ratio of target dsDNA showed the highest intensity absorbance maxima at 525 nm which indicates that interparticle distances between AuNP-bifunctional oligonucleotide probe conjugates were maximum in these samples. This is because the number of unhybridized AuNP-bifunctional oligonucleotide probes is greater than the number of AuNP-bifunctional oligonucleotide probe conjugates hybridized with dsDNA target, at lower dsDNA concentrations. The unhybridized AuNP-bifunctional oligonucleotide probes repel each other in solution which maintains the maximum interparticle distance. This could be indicated by the maximum absorbance of incident light rather than its scattering. After increasing the concentration of dsDNA target up to a ratio of 1.0, the AuNP-bifunctional oligonucleotide probe conjugates were completely hybridized with their target to form a satellite like gold nanoparticle cluster structure which leads to a significant reduction of interparticle distance.65,69 This could increase the scattering of the incident light rather than its absorbance and as a result, the intensity of absorbance was reduced significantly from the ratio of 0.2 to the ratio of 1.0 (Fig. 5C). Significant color changes were observed after the addition of the optimized salt concentration to the solutions of the AuNP conjugated bifunctional oligo probe hybridized with different ratios of the dsDNA targets. The red color of the AuNP-bifunctional oligonucleotide probe conjugate was completely changed into blue at the ratio 0.0, after the addition of 4 M NaCl as shown in Fig. 6A. This is due to the salt induced aggregation of AuNP-bifunctional oligonucleotide probes in the complete absence of target DNA. The degree of salt induced aggregation was reduced significantly and a light bluish purple color resulted for the AuNP-bifunctional oligonucleotide probe solution at a ratio of 0.2; the complex solutions increasingly retained their original reddish purple color when increasing the concentration of target DNA from a ratio of 0.2 to 1.0 as shown Fig. 6A. The hybridization of AuNP-bifunctional oligonucleotide probes with their targets caused small stretches of double helix structure to be formed between the probes and targets which provided a strong resistance force against salt induced aggregation and maintained significant interparticle distances between the AuNP-bifunctional oligonucleotide probes. This also prevented particle aggregation and overlapping. The optical absorbance properties of the corresponding hybridized solutions (Ref, ratios at 0.0, 0.2, 0.4, 0.6, 0.8 and 1.0) are shown in the UV-vis spectra in Fig. 6B. The intensity of optical absorbance at 525 nm for spectra ‘a-f’ was found to increase gradually as the concentration of dsDNA target increased from a ratio of 0.0 to 1.0 after the salt treatment. The absorbance intensity of the ‘Ref’ solution was found to be very high because it is free from salt treatment. Fig. 6C shows the relationship between the concentration of the dsDNA target and the optical absorbance properties of the AuNP-bifunctional oligonucleotide probe. The absorbance intensity at 525 nm of the AuNP solutions increased with respect to increasing dsDNA targets (0.0–1.0 ratios) at high salt concentration. This is due to the increase in the number of AuNP-bifunctional oligonucleotide probe conjugates hybridized with their target dsDNA which tend to form satellite structures as depicted in the schematic representation in Fig. 1. The distance dependent SPR is a reasonable explanation for the different optical absorbance properties of hybridized AuNP-bifunctional oligonucleotide probe conjugates with target DNA at different concentrations.10,68 The detection sensitivity limit of this bifunctional nanoprobe assay was found to be ∼7.2–18 ng, which is a twofold improvement on the earlier report.20
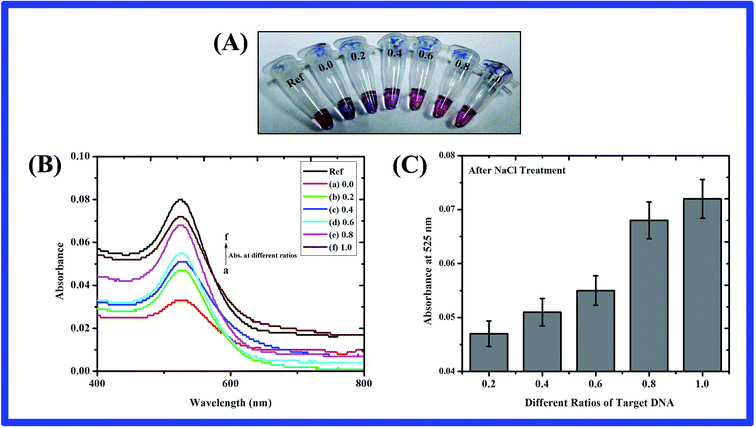 |
| Fig. 6 Colorimetric detection of ToLCNDV DNA using the AuNP-bifunctional oligonucleotide probe. (A) Photographic image of hybridized AuNP-bifunctional oligonucleotide probes with dsDNA targets at different ratios (0.0-1.0) after salt treatment, (B) corresponding UV-visible absorbance spectra and (C) bar graph of degree of absorbance at 525 nm. The ‘Ref’ sample contains AuNP-bifunctional oligonucleotide probe in the absence of both dsDNA target and salt. | |
While nanoparticle cluster assembly of AuNP-bifunctional oligonucleotide probes was found in the presence of target DNA, conjugates were found to be aggregated in the absence of target DNA after salt treatment, as shown in the HR-TEM images in Fig. 7A and B, respectively. A cluster like assembly of AuNP-bifunctional oligonucleotide probes was obtained by sequence specific hybridization with the dsDNA target. The AuNP-bifunctional oligonucleotide probe incorporates probes 1 and 2 which are specific for the 3′ end of the forward and 5′ end of the reverse stands of the dsDNA target. Probe 1 of one conjugate was hybridized with the 3′ end of the dsDNA target and probe 2 of another particle was hybridized with the 5′ end of the dsDNA target. Similarly each AuNP conjugated bifunctional oligonucleotide probe offered additional numbers of probes 1 and 2, which could be hybridized with the dsDNA target to form a very large AuNP cluster through DNA template self-assembly (n = ∞). The inter-particle distance (∼1.3 nm to 2.3 nm) between AuNP-bifunctional oligonucleotide probes was maintained by the double helix form of the dsDNA target and the conjugated probes at high salt concentration. Moreover, different configurations of AuNP-bifunctional oligonucleotide probe cluster assemblies were found through HR-TEM analysis after the hybridization with the dsDNA target; individual clusters comprising 2−11 nanoparticles are shown in Fig. 8. Nanoparticle cluster assembly of AuNP-bifunctional oligonucleotide probes consisting of a single AuNP-bifunctional conjugate core anchored with mono (a), dimer (b), trimers (c & d), tetramer (e), hexamer (f), octamer (g) and decamer (h) AuNP-bifunctional oligonucleotide probe conjugates through hybridization with dsDNA targets are shown in Fig. 8 (a-h, with n = 2–11 configurations, respectively). In addition, a pyramid like assembly of AuNP-bifunctional oligonucleotide probe conjugates (n = 10) was observed in HR-TEM analysis (Fig. 8i). The step-wise assembly of the nanocluster was achieved by anchoring 6 AuNP-bifunctional oligonucleotide probe conjugates with a single core AuNP-bifunctional oligonucleotide conjugate in a defined nano flower-like structure in the presence of dsDNA targets (Fig. 8a–f). However, the number of AuNP-bifunctional oligonucleotide probe conjugates anchored with a single core could be extended to 8 and 10 due to increases in the size of the core from ∼19 nm to 55 nm and 63 nm, respectively. These increased sizes for the AuNP-bifunctional oligonucleotide probe core were found accidently in TEM analysis in the form of self-assembled gold nanoclusters. This accidental finding proves that the increase in the size of the AuNP core also increases the number of AuNPs anchoring on its surface. The defined flower-like nano gold cluster assembly was observed only in the presence of target dsDNA. It confirms that this dsDNA target guides the nanocluster assembly of AuNP-bifunctional oligonucleotide probes through hybridization when there is a perfect complementary match between the AuNP-bifunctional oligonucleotide probe and its targets.27,70 Furthermore, the dsDNA target also guides the pyramid-like structure assembly of AuNP-bifunctional oligonucleotide probes as found in the HR-TEM analysis (Fig. 8i). Moreover, interparticle distances between the core and the anchored AuNP-bifunctional oligonucleotide probe conjugates were found to be 0.9 nm to 1.2 nm in all assembled nanocluster structures and this could be maintained by the presence of the dsDNA targets between the AuNP-bifunctional oligonucleotide probe conjugates after the hybridization.
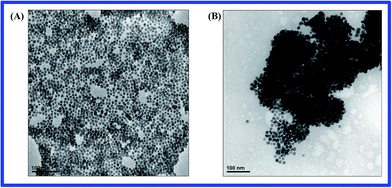 |
| Fig. 7 HR-TEM images of the AuNP-bifunctional oligonucleotide probe (A) hybridized with its dsDNA target to give large AuNP clusters, and (B) aggregated without the dsDNA target, both after salt treatment. | |
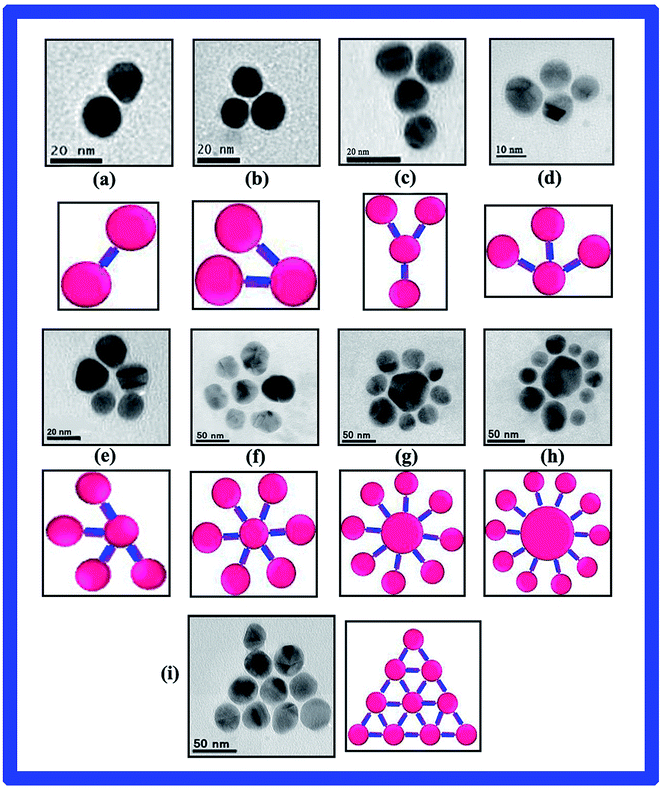 |
| Fig. 8 Flower and pyramid structures formed by the DNA templated self-assembly of AuNP clusters through hybridization of the AuNP-bifunctional oligonucleotide probe with its dsDNA target. (a-h) Individual flower-like clusters comprising 2-11 nanoparticles as petals and consisting of a single AuNP-bifunctional oligonucleotide probe conjugate core anchored with mono (a), dimer (b), trimers (c&d), tetramer (e), hexamer (f), octamer (g) and decamer (h) AuNP-bifunctional oligonucleotide probe conjugates. (i) Pyramid like structure assembly of AuNPs. | |
Conclusion
The present work investigates and discusses the DNA templated 3D self-assembly of AuNP clusters in different configurations in the colorimetric detection of plant viral DNA using AuNP-bifunctional oligonucleotide probes. Citrate capped gold nanoparticles of ∼19 nm were synthesized for the preparation of AuNP-bifunctional oligonucleotide probe conjugates. Equal concentrations of thiolated oligo probes 1 and 2, specific for the ToLCNDV CP gene, were immobilized on AuNPs through Au-SH chemistry. The total number of ssoligo probes 1 and 2 chemisorbed on each nanoparticle was found to be ∼105. The AuNP conjugated bifunctional oligonucleotide probe was used to detect both strands of the dsDNA target simultaneously. AuNP-bifunctional oligonucleotide probes were hybridized with different concentrations of ToLCNDV DNA (ratios 0.0–1.0). The intensity of optical absorbance of the conjugates gradually decreased with increasing dsDNA concentration (ratios 0.0–1.0) after the hybridization. AuNP-bifunctional oligonucleotide probes self-assembled with the dsDNA target in close proximity and with reduced inter-particle distances. This led to changes in optical absorbance properties without significant color changes. After salt treatment, free AuNP-bifunctional oligonucleotide probes aggregated, causing color changes from red to blue. Hybridized AuNP-bifunctional oligonucleotide probes were not aggregated and showed stability in high salt concentrations. The resistance to salt induced aggregation increased gradually with increasing concentration of the dsDNA target from a ratio of 0.2 to 1.0, and the red color was retained accordingly. Furthermore, the dsDNA target guided the 3D self-assembly of gold nanoparticle clusters with different configurations (n = 2–11 and ∞) in flower and pyramid shapes. The number of anchored AuNP-bifunctional oligonucleotide probes on each central core cluster varied from 6 to 8 and 10, due to increases in the size of the core AuNP. The results prove that AuNP-bifunctional oligonucleotide probes can detect both the forward and the reverse strands of a dsDNA target which reduces the concentration of DNA target required for the nanoassay. The limit of detection sensitivity of the bifunctional nanoprobe assay was 7.2 ng, which was a twofold improvement on earlier reports. This method is simpler and more rapid than the existing conventional methods. This work also provides new insight into the preparation of gold nanoparticle clusters in defined shapes and sizes using a single type of AuNPs. This proof of concept will help in the development of an ultra sensitive nanoassay method and in the preparation of gold nanoparticle clusters for materialsscience applications.
Acknowledgements
The authors thank UGC [F. No. 37-440/2009 (SR)] and CSIR [F. No. 9/115(0763)/2013-EMR-I, Government of India for their financial assistance. The authors also thank The Director, NCNS & NT, University of Madras (UNOM) for HR-TEM and Raman 11 characterizations.
References
- W. Cai, T. Gao, H. Hong and J. Sun, Application of Au nanoparticles in cancer nanotechnology, Nanotechnol., Sci. Appl., 2008, 1, 17–32 CAS.
- N. L. Rosi and C. A. Mirkin, Nanostructures in biodiagnostics, Chem. Rev., 2005, 105, 1547–1562 CrossRef CAS PubMed.
- N. C. Tansil and Z. Gao, Nanoparticles in biomolecular detection, Nano Today, 2006, 1, 1–37 CrossRef.
- A. Fu, C. M. Micheel, J. Cha, H. Chang, H. Yang and A. P. Alivisatos, Discrete nanostructures of quantum dots/Au with DNA, J. Am. Chem. Soc., 2004, 126, 10832–10833 CrossRef CAS PubMed.
- E. D. Goluch, J. M. Nam, D. G. Georganopoulou, T. N. Chiesl, K. A. Shaikh, K. Ryu, A. E. Barron, C. A. Mirkin and C. Liu, A bio-barcode assay for on-chip attomolar-sensitivity protein detection, Lab Chip, 2006, 6, 1293–1299 RSC.
- S. J. Park, A. A. Lazarides, C. A. Mirkin, P. W. Brazis, C. R. Kannewurf and R. L. Letsinger, The electrical properties of gold nanoparticle assemblies linked by DNA, Angew. Chem., Int. Ed., 2000, 39, 3845–3848 CrossRef CAS.
- J. M. Nam, C. S. Thaxton and C. A. Mirkin, Nanoparticle-based bio-bar codes for the ultrasensitive detection of proteins, Science, 2003, 301, 1884–1886 CrossRef CAS PubMed.
- C. A. Mirkin, R. L. Letsinger, R. C. Mucic and J. J. Storhoff, A DNA-based method for rationally assembling nanoparticles into macroscopic materials, Nature, 1996, 382, 607–609 CrossRef CAS PubMed.
- G. P. Mitchell, C. A. Mirkin and R. L. Letsinger, Programmed assembly of DNA functionalized quantum dots, J. Am. Chem. Soc., 1999, 121, 8122–8123 CrossRef CAS.
- J. M. Nam, S. I. Stoeva and C. A. Mirkin, Bio-bar-code based DNA detection with PCR-like sensitivity, J. Am. Chem. Soc., 2004, 126, 5932–5933 CrossRef CAS PubMed.
- R. Shukla, V. Bansal, M. Chaudhary, A. Basu, R. R. Bhonde and M. Sastry, Biocompatibility of gold nanoparticles and their endocytotic fate inside the cellular compartment: a microscopic overview, Langmuir, 2005, 21, 10644–10654 CrossRef CAS PubMed.
- L. M. Liz-Marzan, Tailoring surface plasmons through the morphology and assembly of metal nanoparticles, Langmuir, 2006, 22, 32–41 CrossRef CAS PubMed.
- N. L. Rosi, D. A. Giljohann, C. S. Thaxton, A. K. R. Lytton-Jean, M. S. Han and C. A. Mirkin, Oligonucleotide-modified gold nanoparticles for intracellular gene regulation, Science, 2006, 312, 1027–1030 CrossRef CAS PubMed.
- A. P. Alivisatos, K. P. Johnsson, X. Peng, T. E. Wilson, C. J. Loweth, M. P. Bruchez jr and P. G. Schultz, Organization of “nanocrystal molecules” using DNA, Nature, 1996, 382, 609–611 CrossRef CAS PubMed.
- K. Sato, K. Hosokawa and M. Maeda, Rapid aggregation of gold nanoparticles induced by non-cross-linking DNA hybridization, J. Am. Chem. Soc., 2003, 125, 8102–8103 CrossRef CAS PubMed.
- K. Sato, K. Hosokawa and M. Maeda, Non-cross-linking gold nanoparticle aggregation as a detection method for single-base substitutions, Nucleic Acids Res., 2005, 33, e4 CrossRef PubMed.
- G. Doria, R. Franco and P. Baptista, Nanodiagnostics: fast colorimetric method for single nucleotide polymorphism/mutation detection, IET Nanobiotechnol., 2007, 1, 53–57 CrossRef CAS PubMed.
- J. W. Qin and L. Y. L. Yung, Nanoparticle-based detection and quantification of DNA with single nucleotide polymorphism (SNP) discrimination selectivity, Nucleic Acids Res., 2007, 35, e111 CrossRef PubMed.
- J. J. Storhoff, R. Elghanian, C. R. Mucic, C. A. Mirkin and L. R. Letsinger, One-pot colorimetric differentiation of polynucleotides with single base imperfections using gold nanoparticle probes, J. Am. Chem. Soc., 1998, 120, 1959–1964 CrossRef CAS.
- B. Padmavathy, R. Vinoth Kumar and B. M. Jaffar Ali, A direct detection of Escherichia coli genomic DNA using gold nanoprobes, J. Nanobiotechnol., 2012, 10, 8 CrossRef PubMed.
- Y. Seetang-Nun, W. Jaroenrama, S. Sriurairatana, R. Suebsing and W. Kiatpathomchai, Visual detection of white spot syndrome virus using DNA-functionalized gold nanoparticles as probes combined with loop-mediated isothermal amplification, Mol. Cell. Probes, 2013, 27, 71–79 CrossRef CAS PubMed.
- P. Upadhyay, M. Hanif and S. Bhaskar, Visual detection of IS6110 of Mycobacterium tuberculosis in sputum samples using a test based on colloidal gold and latex beads, Clin. Microbiol. Infect., 2006, 12, 1118–1122 CrossRef CAS PubMed.
- P. Costa, A. Amaro, V. Botelho, J. Inacio and P. V. Baptista, Gold nanoprobe assay for the identification of mycobacteria of the Mycobacterium Tuberculosis complex, Clin. Microbiol. Infect., 2010, 16, 1464–1469 CrossRef CAS PubMed.
- Y. Wen, K. C. McLaughlin, K. P. Lo, H. Yang and F. H. Sleiman, Stable Gold nanoparticle conjugation to internal DNA positions: facile generation of discrete gold nanoparticle-DNA assemblies, Bioconjugate Chem., 2010, 21, 1413–1416 CrossRef CAS PubMed.
- L. M. Demers, C. A. Mirkin, R. C. Mucic, R. A. Reynolds, R. L. Letsinger, R. Elghanian, H. Deng, Y. Xu, Y. Liu, Z. Che, H. Guo, S. Shan, Y. Sun, X. Liu, K. Huang, X. Ma, Y. Wu and X. J. Liang, Gold nanoparticles with asymmetric polymerase chain reaction for colorimetric detection of DNA sequence, Anal. Chem., 2012, 84, 1253–1258 CrossRef PubMed.
- H. Zhang, E. W. Edwards, D. Wang and H. Mohwald, Directing the self-assembly of nanocrystals beyond colloidal crystallization, Phys. Chem. Chem. Phys., 2006, 8, 3288–3299 RSC.
- R. C. Mucic, J. J. Storhoff, C. A. Mirkin and R. L. Letsinger, DNA-directed synthesis of binary nanoparticle network materials, J. Am. Chem. Soc., 1998, 120, 12674–12675 CrossRef CAS.
- T. Ohshiro, T. Zako, R. W. Tamaki, T. Tanaka and M. Maeda, A facile method towards cyclic assembly of gold nanoparticles using DNA template alone, Chem. Commun., 2010, 46, 6132–6134 RSC.
- W. Yan, L. Xu, C. Xu, W. Ma, H. Kuang, L. Wang and N. A. Kotov, Self-assembly of chiral nanoparticle pyramids with strong R/S optical activity, J. Am. Chem. Soc., 2012, 134, 15114–15121 CrossRef CAS PubMed.
- C. T. Nguyen, T. H. Tran, X. Lu and R. M. Kasi, Self-assembled nanoparticles from thiol functionalized liquid crystalline brush block copolymers for dual encapsulation of doxorubicin and gold nanoparticles, Polym. Chem., 2014, 5, 2774–2783 RSC.
- M. K. Nakhla and D. P. Maxwell, Epidemiology and management of tomato yellow leaf curl disease, in Plant Virus Disease Control, ed. A. Hadidi, R. K. Khetarpal and H. Koganezawa, The American Phytopathological Society, St. Paul, MN, USA, 1999, pp. 565–583 Search PubMed.
- R. V. Chowda Reddy, J. Colvin, V. Muniyappa and S. Seal, Diversity and distribution of begomoviruses infecting tomato in India, Arch. Virol., 2005, 150, 845–867 CrossRef PubMed.
- S. Rho, S. J. Kim, S. C. Lee, J. H. Chang, H.-G. Kang and J. Choi, Colorimetric detection of ssDNA in a solution, Curr. Appl. Phys., 2009, 9, 534–537 CrossRef.
- Y. Zhang, Z. Tang, J. Wang, H. Wu, A. Maham and Y. Lin, Label-free DNA biosensor based on SERS molecular sentinel on nanowave chip, Anal. Chem., 2010, 82, 6440–6446 CrossRef PubMed.
- K. C. Grabar, R. G. Freeman, M. B. Hommer and M. J. Natan, Preparation and characterization of Au colloid monolayers, Anal. Chem., 1995, 67, 735–743 CrossRef CAS.
- J. Turkevich, P. C. Stevenson and J. Hillier, A study of the nucleation and growth processes in the synthesis of colloidal gold, Discuss. Faraday Soc., 1951, 11, 55–75 RSC.
- P. Zhao, N. Li and D. Astruc, State of the art in gold nanoparticle synthesis, Coord. Chem. Rev., 2013, 257, 638–665 CrossRef CAS.
- F. A. Tanious, B. Nguyen and W. D. Wilson, Biosensor-surface plasmon resonance methods for quantitative analysis of biomolecular interactions, Methods Cell Biol., 2008, 84, 53–77 CAS.
- S. Link and M. A. El-Sayed, Size and temperature dependence of the plasmon absorption of colloidal gold nanoparticles, J. Phys. Chem. B, 1999, 103(21), 4212–4217 CrossRef CAS.
- X. Huang and M. A. El-Sayed, Gold nanoparticles: optical properties and implementations in cancer diagnosis and photothermal therapy, J. Adv. Res., 2010, 1, 13–28 CrossRef.
- D. Philip, Synthesis and spectroscopic characterization of gold nanoparticles, Spectrochim. Acta, Part A, 2008, 71, 80–85 CrossRef PubMed.
- S. K. Sivaraman, S. Kumar and V. Santhanam, Monodisperse sub-10 nm gold nanoparticles by reversing the order of addition in Turkevich method-the role of chloroauric acid, J. Colloid Interface Sci., 2011, 361, 543–547 CrossRef CAS PubMed.
- H. Liu, N. Pierre-Pierre and Q. Huo, Dynamic light scattering for gold nanorod size characterization and study of nanorod–protein interactions, Gold Bull., 2012, 45, 187–195 CrossRef CAS.
- D. J. Lewis, T. M. Day, J. V. MacPherson and Z. Pikramenou, Luminescent nanobeads: attachment of surface reactive Eu(III) complexes to gold nanoparticles, Chem. Commun., 2006, 1433–1435 RSC.
- T. A. Taton, Preparation of gold nanoparticle–DNA conjugates, DNA nanotechnology: current protocols in nucleic acid chemistry, John Wiley and Sons, Inc, 2002, pp. 12.2.1–12.2.12, DOI:10.1002/0471142700.nc1202s09.
- P. Hazarika, T. Giorgi, M. Reibner, B. Ceyhan and C. M. Niemeyer, Synthesis and characterization of deoxyribonucleic acid-conjugated gold nanoparticles, Methods in molecular biology- bioconjugation protocols: strategies and methods, ed. C. M. Niemeyer, Humana Press Inc, Totowa, NJ, 2004, pp. 295–304 Search PubMed.
- R. Wilson, The use of gold nanoparticles in diagnostics and detection, Chem. Soc. Rev., 2008, 37, 2028–2045 RSC.
- J. C. Love, L. A. Estroff, J. K. Kriebel, R. G. Nuzzo and G. M. Whitesides, Self-assembled monolayers of thiolates on metals as a form of nanotechnology, Chem. Rev., 2005, 105, 1103–1169 CrossRef CAS PubMed.
- R. A. Sperling and W. J. Parak, Surface modification, functionalization and bioconjugation of colloidal inorganic nanoparticles, Philos. Trans. R. Soc., A, 2010, 368, 1333–1383 CrossRef CAS PubMed.
- C. A. Widrig, C. Chung and N. D. Porter, The electrochemical desorption of n-alkanethiol monolayers from polycrystalline Au and Ag electrodes, J. Electroanal. Chem., 1991, 310, 335–359 CrossRef CAS.
- S. Y. Lin, Y. Tsai, C. C. Chen, C. M. Lin and C. H. Chen, Two-step functionalization of neutral and positively charged thiols onto citrate-stabilized Au nanoparticles, J. Phys. Chem. B, 2004, 108, 2134–2139 CrossRef CAS.
- H. X. Li and L. J. Rothberg, Colorimetric detection of DNA sequences based on electrostatic interactions with unmodified gold nanoparticles, Proc. Natl. Acad. Sci. U. S. A., 2004, 101, 14036–14039 CrossRef CAS PubMed.
- M.-P. N. Bui, T. J. Baek and G. H. Seong, Gold nanoparticle aggregation-based highly sensitive DNA detection using atomic force microscopy, Anal. Bioanal. Chem., 2007, 388, 1185–1190 CrossRef PubMed.
- K. G. Witten, J. C. Bretschneider, T. Eckert, W. Richtering and U. Simon, Assembly of DNA-functionalized gold nanoparticles studied by UV-visible spectroscopy and dynamic light scattering, Phys. Chem. Chem. Phys., 2008, 10, 1870–1875 RSC.
- C. Thiruppathiraja, S. Kamatchiammal, P. Adaikkappan, D. J. Santhosh and M. Alagar, Specific detection of Mycobacterium sp. genomic DNA using dual labeled gold nanoparticle based electrochemical biosensor, Anal. Biochem., 2011, 417, 73–79 CrossRef CAS PubMed.
- Q. Dai, X. Liu, J. Coutts, L. Austin and Q. Huo, A one-step highly sensitive method for DNA detection using dynamic light scattering, J. Am. Chem. Soc., 2008, 130, 8138–8139 CrossRef CAS PubMed.
- Y. X. Zhang, J. Zheng, G. Gao, Y. F. Kong, X. Zhi, K. Wang, X. Q. Zhang and D. X. Cui, Biosynthesis of gold nanoparticles using chloroplasts, Int. J. Nanomed., 2011, 6, 2899–2906 CrossRef CAS PubMed.
- T. Yajima, Y. Yu and M. Futamata, Closely adjacent gold nanoparticles linked by chemisorption of neutral rhodamine 123 molecules providing enormous SERS intensity, Phys. Chem. Chem. Phys., 2011, 13, 12454–12462 RSC.
- T. Yajima, Y. Yu and M. Futamata, Steric hindrance in cationic and neutral rhodamine 6G molecules adsorbed on Au nanoparticles, J. Raman Spectrosc., 2013, 44, 406–411 CrossRef CAS.
- S. Kalmodia, J. Harjwani, R. Rajeswari, W. Yang, C. J. Barrow, S. Ramaprabhu, S. Krishnakumar and S. Velchuri, Synthesis and characterization of surface enhanced Raman scattered gold nanoparticles, Int. J. Nanomed., 2013, 8, 4327–4338 CrossRef PubMed.
- D. K. Lim, K. S. Jeon, J. H. Hwang, H. Kim, S. Kwon, Y. D. Suh and J. M. Nam, Highly uniform and reproducible surface-enhanced Raman scattering from DNA tailorable nanoparticles with 1 nm interior gap, Nat. Nanotechnol., 2011, 6, 452–460 CrossRef CAS PubMed.
- M. M. Harper, K. S. McKeatingw and K. Faulds, Recent developments and future directions in SERS for bioanalysis, Phys. Chem. Chem. Phys., 2013, 15, 5312–5328 RSC.
- C. S. Weisbecker, M. V. Merritt and G. M. Whitesides, Molecular self-assembly of aliphatic thiols on gold colloids, Langmuir, 1996, 12, 3763–3772 CrossRef CAS.
- L. M. Demers, C. A. Mirkin, R. C. Mucic, R. A. Reynolds, R. L. Letsinger, R. Elghanian and G. Viswanadham, A fluorescence based method for determining the surface coverage and hybridization efficiency of thiol-capped oligonucleotides bound to gold thin films and nanoparticles, Anal. Chem., 2000, 72, 5535–5541 CrossRef CAS PubMed.
- E. Y. Kim, J. Stanton, R. A. Vega, K. J. Kunstman, C. A. Mirkin and S. M. Wolinsky, A real-time PCR-based method for determining the surface coverage of thiol-capped oligonucleotides bound onto gold nanoparticles, Nucleic Acids Res., 2006, 34(7), 1–7 CrossRef PubMed.
- H. D. Hill, J. E. Millstone, M. J. Banholzer and C. A. Mirkin, The role of radius curvature plays in thiolated oligonucleotide loading on gold nanoparticles, ACS Nano, 2009, 3(2), 418–424 CrossRef CAS PubMed.
- J. J. Storhoff, R. Elghanian, R. C. Mucic, C. A. Mirkin and R. L. Letsinger, One-pot colorimetric differentiation of polynucleotides with single base imperfections using gold nanoparticle probes, J. Am. Chem. Soc., 1998, 120, 1959–1964 CrossRef CAS.
- T. T. Tsai, S. W. Shen, C. M. Cheng and C. F. Chen, Paper-based tuberculosis diagnostic devices with colorimetric gold nanoparticles, Sci. Technol. Adv. Mater., 2013, 14, 1–7 CrossRef.
- J. J. Storhoff, A. A. Lazarides, R. C. Mucic, C. A. Mirkin, R. L. Letsinger and G. C. Schatz, What Controls the Optical Properties of DNA-Linked Gold Nanoparticle Assemblies?, J. Am. Chem. Soc., 2000, 122, 4640–4650 CrossRef CAS.
- S. L. Dellaporta, J. Wood and J. B. Hicks, Experimental protocols; a plant mini preparation: version II, Plant Mol. Biol. Rep., 1983, 1, 19–21 CrossRef CAS.
|
This journal is © The Royal Society of Chemistry 2016 |