DOI:
10.1039/C5RA25485J
(Paper)
RSC Adv., 2016,
6, 23233-23241
Phosphate adsorption on novel hydrogel beads with interpenetrating network (IPN) structure in aqueous solutions: kinetics, isotherms and regeneration
Received
1st December 2015
, Accepted 22nd February 2016
First published on 24th February 2016
Abstract
Adsorption has attracted much attention for its effectiveness, low cost and the possibility of regeneration among many phosphorus removal methods. In this study, a novel interpenetrating network (IPN) structural hydrogel based on N-isopropylacrylamide and aluminum alginate was synthesized and applied for phosphate adsorption. SEM, FTIR and XPS were applied to characterize the poly N-isopropylacrylamide/aluminum alginate (PNIPAM/AA) IPN hydrogel beads before and after adsorption. It was found that the maximum adsorption capacity was reached at pH = 3, adsorption capacity decreased with adsorbent dosage increasing, and SO42− had much more negative effects on phosphate adsorption compared with Cl− and NO3−. A pseudo-second-order kinetic model and intra-particle kinetic model fitted well with the experimental data, indicating chemical adsorption and intra-particle diffusion was the rate-controlling step of phosphate adsorption. Moreover, equilibrium isotherm experimental data were well described by the Freundlich and Slips model, suggesting heterogeneous multilayer adsorption was dominant during adsorption process. The regeneration study indicated that the adsorption capacity of adsorbent remained at a relatively high level after five times of regeneration. Column adsorption and regeneration results showed PNIPAM/AA hydrogels had strong phosphate adsorption capacity and had potential in large scale application. Hence, PNIPAM/AA hydrogel beads could be a promising adsorbent for phosphate recycle in the future.
Introduction
Phosphorus is an essential element for the development and sustainability of modern human society. However, if wastewater containing phosphate is directly discharged into natural waters without elimination or recovery, it will cause undesirable eutrophication, algae blooms and further deterioration of water quality.1 Therefore, it is necessary to remove or recycle phosphorus from wastewater before discharge.
At present, many technologies have been developed to remove phosphate from wastewater, including biological treatment,2 chemical precipitation3 and membrane filtration.4 Compared with these methods, adsorption has been considered as a promising technique because it provides higher treatment efficiency and faster removal rate.5 Also, recycling of valuable phosphate and regeneration of adsorbent could be achieved. Therefore, a variety of materials have been applied as phosphate adsorbents, including graphene,6 activated carbon,7 zirconium oxide,8 layered double hydroxides,9 Fe–Cu binary oxides,10 and amine functionalized epichlorohydrin-grafted cellulose.11 Most of these adsorbents rely on introducing metal ions or amine group to increase phosphate adsorption capacity. Although nanomaterial-based adsorbents have attracted the attention of many researchers, the difficulties of separation and reusability prevent their application. Moreover, residual nanomaterials in water have also brought concerns about security.12 Bulk materials have advantage over nanomaterials in terms of separation as adsorbents in water treatment, however, they also possess drawbacks of low adsorption capacity or slow adsorption rate.13
Recently, hydrogels with three-dimensional network have been used as adsorbents in water treatment.14 Hydrogels' unique feature of high swelling in water is good for foreign ions and anions get access into hydrogels easily, and this property makes hydrogel a promising adsorbent.15 Traditional single-network hydrogels are actually weak in mechanical properties, however, interpenetrating network (IPN) hydrogels with multicomponent networks have been designed to have an advance in mechanical strength, and they could exhibit a nice regeneration behavior as adsorbents.16 In addition, some low-cost and environment-friendly hydrogels based on natural polymers have been developed for phosphate adsorption, such as sodium alginate (SA), polyvinyl alcohol (PVA)17 and chitosan.18 Among them, alginate is a biological material and can interact with polyvalent cations by ion exchange to form stable hydrogels in a temperate condition.19,20 Besides, as a widely used polymer containing amine groups, N-isopropylacrylamide (NIPAM) possesses the potential to bind with phosphate in aqueous solutions. Our group has developed some novel hydrogels based on NIPAM in water treatment.21,22 Considering the fact that aluminum is a trivalent metal, and aluminum alginate (AA) is more favorable compared with calcium alginate for phosphate adsorption, the combination of AA and NIPAM with IPN structure could be a novel adsorbent with a relatively nice adsorption performance. For these reasons, PNIPAM/AA IPN hydrogel beads may be used as a new phosphate removal adsorbent, and have a potential application in phosphate adsorption and recycles.
In this work, PNIPAM/AA IPN hydrogel beads were prepared for phosphate adsorption. And to the best of our acknowledgement, few studies about phosphate adsorption on PNIPAM/AA hydrogel beads were reported.17,18 Here, the preparation, characterization, batch and column adsorption experimental parameters, as well as regeneration behaviors of PNIPAM/AA IPN hydrogel beads were investigated. This work is expected to expand the application field of IPN hydrogel into phosphate adsorption in water treatment, and provide a new method for phosphate recycles as well as theoretical basis.
Materials and methods
Materials
N-Isopropylacrylamide (NIPAM, 97%), sodium alginate (SA), N,N,N′,N′-tetramethylethylenediamine (TEMED, 99%) and N,N′-methylenebisacrylamide (BIS, 99%) were purchased from Aladdin, Shanghai, China. Ammonium persulfate (APS), potassium dihydrogen phosphate (KH2PO4), hydrochloric acid (HCl), sodium hydrate (NaOH) and aluminum chloride (AlCl3) were purchased from Sinopharm Chemical Reagent Co., Ltd, China. All the other chemical agents were analytical purity. The deionized (DI) water applied in the experiment was prepared by an ultrapure water system (Pcdxj-10, Pincheng, China).
Preparation of hydrogel beads
The PNIPAM/AA hydrogel beads were prepared according to the method proposed previously with a few modifications,23 as shown in Scheme 1. Briefly, 3.84 g NIPAM, 1 g SA, 0.2616 g BIS (5 mol% to NIPAM) and 0.5 mL TEMED were dissolved in 50 mL DI water overnight with a magnetic stirring at 600 rpm, and then the solution was left undisturbed for one hour to remove the air bubbles. The above mixed viscous solution was then dropped into 3% (w/v) AlCl3 and 0.5% (w/v) APS mixed solution, which was previously nitrogen purged for thirty minutes. The hydrogel beads were formed instantaneously and cured for 24 hours under nitrogen atmosphere with a magnetic stirring at 100 rpm. Finally, the hydrogel beads were washed several times and then immersed in deionized water to remove the unreacted monomers. The obtained hydrogel beads were then freeze-dried and stored for further use.
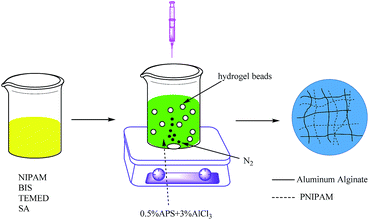 |
| Scheme 1 Schematic diagram of PNIPAM/AA hydrogel beads preparation. | |
Characterization of hydrogel beads
The morphology of the hydrogel beads were examined by a scanning electron microscopy (SU8010, HITACHI, Japan). The functional groups of these hydrogel beads were characterized with Fourier-transform infrared spectroscopy (FTIR) (VERTEX 70, BRUKER, Ettlingen, Germany). The X-ray Photoelectron Spectroscopy (XPS, AXIS-ULTRA DLD-600W, Kratos Corporation, Japan) was applied to detect the binding energy of elements of hydrogel beads before and after adsorption.
Batch adsorption experiment
Batch experiments were conducted to examine the adsorption behavior. For the adsorption kinetics, 0.05 g dry hydrogel beads and 20 mL KH2PO4 solution (initial phosphorus concentration = 100 mg L−1, 50 mg L−1 and 10 mg L−1, with pH = 3) were put into a 30 mL serum bottle. The sealed bottles were placed in a constant temperature bath oscillator to vibrate at 35 °C at 120 rpm. At regular time intervals, the bottles were taken out for phosphate concentration analysis. The adsorption capacity of the hydrogel beads were evaluated as eqn (1), where qt is the adsorption capacity (mg-P per g) of the hydrogel beads at t (min) time, C0 and Ct represent phosphorus concentration (mg L−1) before and after the adsorption of t (min) time, V is the volume of phosphate aqueous solution, and m is the mass of dry hydrogel beads (g). As for adsorption isotherms, different initial phosphate concentrations ranging from 0 to 200 mg L−1 with pH = 3, were in contact with hydrogel beads for 24 h. For adsorbent dosage on adsorption capacity, 0.05 g to 0.25 g hydrogel beads were performed with same phosphate aqueous solution (concentration = 100 mg L−1, pH = 3). With respect to the pH effect on adsorption capacity, KH2PO4 solutions were adjusted from pH = 2 to 10 by 0.1 M NaOH and HCl. For the coexisting anion competing effect study, the initial phosphorus concentration was 100 mg L−1, and concentrations of Cl−, SO42− and NO3− were 0, 50 and 100 mM. The concentration of phosphate in aqueous solution was measured by the ammonium molybdate spectrophotometric method,24 after appropriate dilution. All the experiments were performed three times and the average value was used. Data analysis and model fitting were implemented with Origin 8 Software (OriginLab Corporation). |
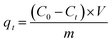 | (1) |
Regeneration of hydrogel beads
For the batch regeneration experiments, phosphate desorption were first carried out with 0.1 M HCl for 12 hours. Then, the hydrogel beads were washed with deionized water and finally put into 3% AlCl3 solution and kept for another 24 hours. After that, the regenerated hydrogel beads were obtained.
Column adsorption and regeneration experiment
The fixed-bed column adsorption experiment was conducted in a glass with a length of 30 cm and inner diameter of 2 cm. About 3 g dried hydrogel beads were placed into the column, and glass beads were used to close and support the bed, which ensured a favorable liquid distribution. The phosphate solutions (50 mg L−1, pH = 3) were pumped downward using a peristaltic pump at a constant flow rate of 6 mL min−1 into the column. The samples were taken at regular time intervals from effluent for phosphate concentration analysis. After the phosphate concentration of effluent became steady, the column was flushed with DI water for some time. Then the column regeneration experiment was initiated by switching to 0.1 M HCl at same flow rate.
Results and discussion
Preparation and characterization of PNIPAM/AA hydrogel beads
The synthesis of PNIPAM/AA IPN hydrogel was based on the following crosslinking reactions. Upon contacting with the droplets, aluminum ions diffuse and crosslink with carboxyl groups immediately and gradually form spherical skeleton of hydrogel beads by ion exchange processes, as shown in Scheme 2. Simultaneously, NIPAM monomers were polymerized inside the beads, applying BIS, APS and TEMED as cross-linker, initiator and accelerator, respectively, as shown in Scheme 3. The formed PNIPAM and aluminum alginate hydrogels constituted the IPN structure, where the two kinds of hydrogels were entangled with each other.
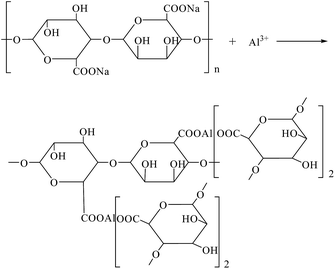 |
| Scheme 2 Chemical reaction of aluminum chloride (AlCl3) and sodium alginate (SA). | |
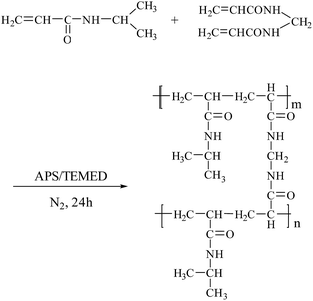 |
| Scheme 3 Chemical reaction of NIPAM, BIS with APS and TEMED. | |
The obtained dry hydrogel beads were in spherical form, as shown in Fig. 1. The average diameter of the hydrogel bead was about 4 mm. The scanning electron microscopy (SEM) of the bead before and after adsorption is shown in Fig. 2. It was clear that the beads exhibited a highly porous inner structure. After adsorption of phosphate, the porous structure became less obvious, and this may be the result that phosphate has been adsorbed and pores inside the hydrogel beads have been blocked.
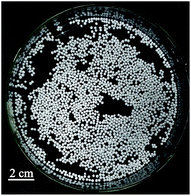 |
| Fig. 1 Photograph of PNIPAM/AA hydrogel beads. | |
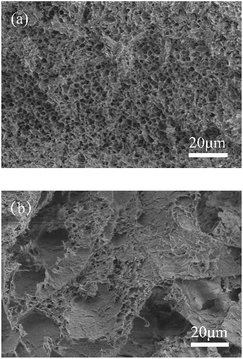 |
| Fig. 2 SEM images of PNIPAM/AA hydrogel beads before (a) and after (b) adsorption of phosphate. | |
Fig. 3 shows Fourier-transform infrared (FTIR) spectra of hydrogels, which were in accordance with literatures.25,26 The spectrum of NIPAM showed stretching vibration at 1618 cm−1 (C
C), 1659 cm−1 (C
O), and 1550 cm−1 (C–N–H), while the spectrum of SA exhibited stretching vibration at 3444 cm−1 (–OH), 1031 cm−1 (C–O), 1616 cm−1 (asymmetric COO−), and 1419 cm−1 (symmetric COO−). In the spectrum of PNIPAM/AA, these peaks were also found except the C
C, illustrating that PNIPAM/AA hydrogels had been synthesized successfully. As for the hydrogel beads after adsorption, the spectrum were similar to PNIPAM/AA, while a new vibration at 1128 cm−1 (P
O) was observed,27 demonstrating that phosphate had been adsorbed onto the hydrogel beads.
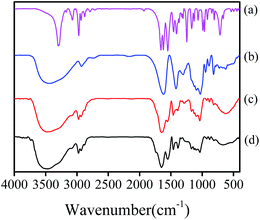 |
| Fig. 3 FTIR spectra of samples: NIPAM (a), SA (b), and PNIPAM/AA hydrogel beads before (c) and after (d) phosphate adsorption. | |
In order to find out the mechanism of phosphate adsorption on hydrogel beads, XPS of hydrogel beads before and after adsorption was conducted, as shown in Fig. 4. It was clear that C, O, N and Al elements existed in both kinds of hydrogel beads, while a new peak whose binding energy was about 134.28 eV appeared after phosphate adsorption. This evidence was due to the P 2p orbital, which implied that phosphate had been adsorbed successfully.
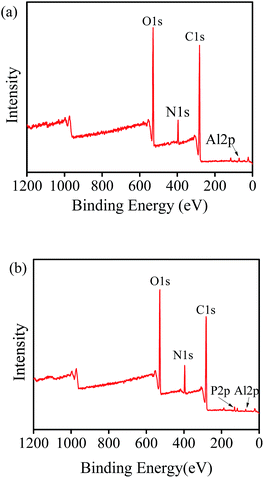 |
| Fig. 4 XPS spectra of PNIPAM/AA hydrogel bead before (a) and after (b) phosphate adsorption. | |
Effect of initial solution pH
The adsorption process is significantly affected by pH, therefore the effect of initial solution pH on phosphate adsorption capacity was investigated. Fig. 5(a) shows the phosphate adsorption at 35 °C with pH varying from 2 to 10. The results showed that the adsorption capacity at pH = 2 was nearly zero and it first increased sharply and then gradually decreased with pH increase, and the maximum adsorption capacity was obtained at pH = 3. Fig. 5(b) illustrates the species distribution of phosphate with variation of pH. At lower pH conditions, H3PO4 is the predominant species of phosphate, and has little attraction to adsorption sites of hydrogel beads. However, phosphate adsorption capacity decreased when pH value was above 3. This might be the result that existing hydroxyl ions can bind with the loaded aluminium ions of PNIPAM/AA hydrogels in alkaline aqueous solutions, thus available adsorption sites for phosphate adsorption decrease and it also leads to lower phosphate adsorption capacity. Dai et al. got similar results by using Cu2+ loaded chitosan hydrogel beads, and found that phosphate adsorption capacity first increased and then decreased with pH value varied from 2 to 12, with maximum adsorption was got at pH = 5.18
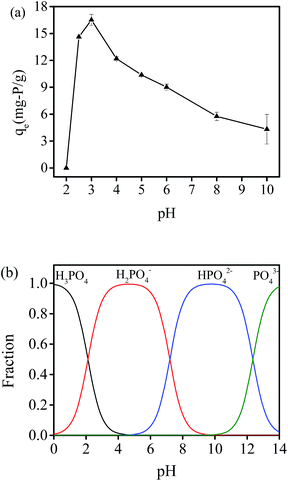 |
| Fig. 5 (a) Effect of pH on phosphate adsorption capacity by PNIPAM/AA hydrogel beads with initial phosphate concentration at 100 mg L−1 (pH = 3, 35 °C, adsorbent dosage = 0.05 g). (b) Species distribution of phosphate with variation of pH. | |
Effect of adsorbent dosage
The effect of adsorbent dosage on adsorption capacity and adsorption extent is shown in Fig. 6. The adsorption capacity decreased from 16.51 mg-P per g to 7.76 mg-P per g, while the adsorption extent increased from 41.07% to 95.37% with dosage of hydrogel beads ranging from 0.05 g to 0.25 g. More amounts of adsorbents can actually provide more adsorption sites, however phosphate adsorption capacity decreased with an increase of adsorbent dosage and a constant initial phosphate concentration. This resulted in phosphate concentration gradient between aqueous solution and adsorbent sites decreased. It is similar to Zhang's result.28 They applied modified sugarcane bagasse in phosphate adsorption, and found that adsorption capacity decreased from 18.73 mg-P per g to 3.99 mg-P per g and adsorption extent increased from 37.1% to 79.8%, with adsorbent dosage rising from 0.1 g to 1.0 g.
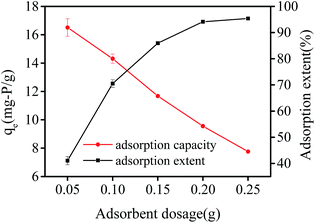 |
| Fig. 6 Effect of adsorbent dosage and adsorption extent on adsorption capacity by PNIPAM/AA hydrogel beads with initial phosphate concentration at 100 mg L−1 (pH = 3, 35 °C). | |
Effect of co-existing anions
In natural waters, some co-existing anions compete with phosphate for adsorption sites. In this study, Cl−, NO3− and SO42− were chosen to study the effect on phosphate adsorption capacity. As shown in Fig. 7, qe stands for phosphate adsorption capacity. The concentrations of Cl− and NO3− increased from 0 to 100 mM, and the phosphate adsorption capacity changed little. However, with the concentration of SO42− varying from 0 to 100 mM, the phosphate adsorption capacity decreased from 16.51 mg-P per g to 6.40 mg-P per g (decreased by 61.2%). It was because SO42− could compete with phosphate for adsorption sites, which might due to the fact that SO42− could bind with Al3+ on the adsorbent more easily and thus prevented phosphate adsorption. These results showed that SO42− had much more negative effect on phosphate adsorption than Cl− and NO3−. Liu et al. got similar results by using zirconium modified chitosan hydrogel beads for phosphate adsorption, and found that phosphate adsorption capacity changed slightly with existence of Cl− and NO3−, while phosphate adsorption capacity decreased by 51.7% when the concentration of existing SO42− increased from 0 to 500 mg L−1.29
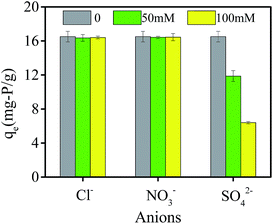 |
| Fig. 7 Effect of co-existing anions on phosphate adsorption capacity by PNIPAM/AA hydrogel beads with initial phosphate concentration at 100 mg L−1 (pH = 3, 35 °C, adsorbent dosage = 0.05 g). | |
Adsorption kinetic
In order to further investigate the mechanism of controlling process of phosphate adsorption, pseudo-first-order (PFO) kinetic model and pseudo-second-order (PSO) kinetic model,30,31 which were in nonlinear form, were applied to fit the adsorption experiments data. The PFO and PSO models are presented as follows:
Pseudo-first-order model:
Pseudo-second-order model:
|
 | (3) |
where
qt is the adsorption capacity of phosphate (mg-P per g) at time
t (min),
qe is the adsorption capacity of phosphate (mg-P per g) at equilibrium,
k1 is the PFO model rate constant (min
−1),
k2 is the PSO model rate constant (g mg
−1 min
−1).
Fig. 8 shows the fitting curves of nonlinear pseudo-first and pseudo-second order models of phosphate adsorption onto hydrogel beads at different initial concentrations (100 mg L−1, 50 mg L−1 and 10 mg L−1). It was clear that the adsorption process can be divided into two processes: a rather fast uptake of phosphate during the initial 240 min, and followed by a slow adsorption period until an equilibrium phosphate concentration was reached. With the increase of initial phosphate concentration, the phosphate adsorption capacity increased. The values of predicted equilibrium adsorption capacity, and related parameters of each models are presented in Table 1. For pseudo-first order model, the predicted values of equilibrium adsorption capacities were: 15.38 mg-P per g, 9.83 mg-P per g, and 3.39 mg-P per g, corresponding to initial phosphate concentrations of 100 mg L−1, 50 mg L−1 and 10 mg L−1, respectively, suggesting that the predicted adsorption capacity was not in agreement with the experimental data. Besides, all the correlation coefficient (R2) were just around 0.97, indicating pseudo-first-order model was not favorable for adsorption kinetic fitting, and physical adsorption may not be related to phosphate adsorption by PNIPAM/AA hydrogel beads. With regard to the pseudo-second order model, all the correlation coefficient (R2) were above 0.99 and higher than that of pseudo-first-order model. Meanwhile, the predicted values of equilibrium adsorption capacities with various initial phosphate concentrations were: 17.47 mg-P per g, 11.23 mg-P per g, and 3.79 mg-P per g, which were closer to the experimental data of 16.51 mg-P per g, 10.46 mg-P per g, and 3.68 mg-P per g, indicating kinetic adsorption experimental data could be well described by pseudo-second-order model. Generally, pseudo-second-order model is applied to estimate adsorption behavior based on the whole range, and it is in relation to chemical adsorption mechanism. Therefore, chemical adsorption might be the rate-controlling step of phosphate adsorption.
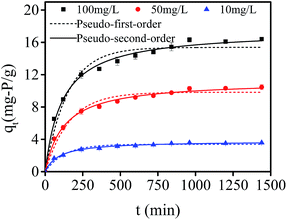 |
| Fig. 8 Fitting curves of pseudo-first-order and pseudo-second-order kinetic model by experimental data with initial phosphate concentration at 10, 50 and 100 mg L−1 (pH = 3, 35 °C, adsorbent dosage = 0.05 g). | |
Table 1 The kinetic model parameters of phosphate adsorption on hydrogel beads at 35 °C and pH = 3
Kinetic model |
Parameter |
C (mg L−1) |
100 |
50 |
10 |
Pseudo-first-order model |
k1 |
0.00668 |
0.00622 |
0.00785 |
qe |
15.38 |
9.83 |
3.39 |
R2 |
0.9680 |
0.9706 |
0.9688 |
Pseudo-second-order model |
k2 |
0.00050 |
0.00072 |
0.00286 |
qe |
17.47 |
11.23 |
3.79 |
R2 |
0.9942 |
0.9952 |
0.9940 |
Intra-particle diffusion model |
kd1 |
0.78138 |
0.48417 |
0.17775 |
R2 |
0.9970 |
0.9975 |
0.9873 |
kd2 |
0.27165 |
0.17673 |
0.05416 |
R2 |
0.9908 |
0.9921 |
0.9910 |
The intra-particle diffusion (IPD) model32 which was in a linear form was used to explain the diffusion process of phosphate adsorption. The IPD model is presented in eqn (4):
where
kd is intra-particle diffusion rate constant (g mg
−1 min
−0.5),
C is the constant on boundary layer thickness. If the plot of
qt versus t0.5 is a straight line, the adsorption process is only controlled by intra-particle model diffusion. However, multi-linear fitting plots of data mean that two or more processes have an influence on adsorption process.
Intra-particle diffusion fitting curves of experimental data are presented in Fig. 9. It could be seen that there were three lines in the case of phosphate adsorption onto hydrogel beads. The first curve passing through the origin represented that boundary layer diffusion had little contribution, indicating phosphate was bound with the external surface of hydrogel beads. The second step was dominated by intra-particle diffusion, suggesting phosphate gradually diffused into the hydrogel beads and lasted for a period of time. The last curve reflected both adsorption sites and phosphate concentration decreased and adsorption reached equilibrium at last. The related parameters of the model are shown in Table 1. All the correlation coefficient (R2) of phase I and phase II were at a relatively high level (all around 0.99), indicating intra-particle diffusion model fitted the experimental data well. Therefore, intra-particle diffusion process may also be the rate-controlling step of phosphate adsorption by hydrogel beads.
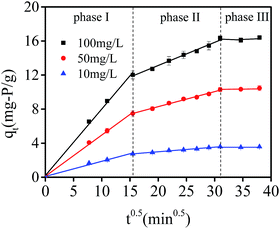 |
| Fig. 9 Intra particle diffusion model fitting curves of experimental data with initial phosphate concentration at 10, 50 and 100 mg L−1 (pH = 3, 35 °C, adsorbent dosage = 0.05 g). | |
Adsorption isotherm
The adsorption isotherms of phosphate are illustrated in Fig. 10. The initial concentration of phosphate varied from 0 to 200 mg L−1. The adsorption capacity increased with an increase in equilibrium phosphate concentrations, while all the isotherms exhibited a concave curve. Langmuir isotherm, Freundlich isotherm, Slips isotherm and Dubinin–Radushkevich (D–R) isotherm33 were applied to fit the experiment data. These nonlinear isotherms are presented as follows:
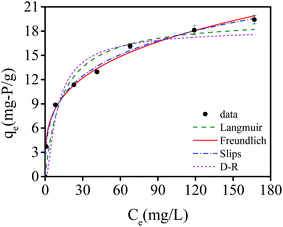 |
| Fig. 10 Equilibrium adsorption isotherms of phosphate by NIPAM/AA hydrogel beads at 35 °C, and fitting isotherm models on experimental data. | |
Langmuir model:
|
 | (5) |
Freundlich model:
Slips model:
|
 | (7) |
Dubinin–Radushkevich (D–R) model:
|
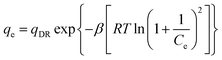 | (8) |
where:
qe is the adsorption capacity of phosphate (mg g
−1) at equilibrium,
Ce (mg L
−1) is concentration of phosphate in aqueous solution at equilibrium,
qm is the maximum adsorption capacity of phosphate in theory, and
Kl is Langmuir constant (L mg
−1),
Kf is Freundlich constant (mg g
−1),
nf is heterogeneity factor,
as and
ns are Slips isotherm constant (L mg
−1),
β is D–R isotherm constant (mol
2 kJ
−2),
R is gas constant (8.314 J mol
−1 K
−1), and
T is temperature (K).
All the isotherm parameters and results of correlation coefficients (R2) based on experimental data are listed in Table 2. Among these models, Langmuir and Freundlich isotherm models are most widely used to evaluate the isotherm character of adsorbents. As Table 2 shows, the correlation coefficients (R2) of Langmuir and Freundlich models were 0.9545 and 0.9950, respectively, indicating Freundlich isotherm fitted experimental data better compared with Langmuir model. Therefore, heterogeneous multilayer adsorption could be dominant during the phosphate adsorption process, which might due to the heterogeneous surface of IPN hydrogels. Slips model is the combination of Langmuir and Freundlich model. The higher value of R2 (0.9967) of Slips model reflected a favorable fitness of experimental data. Besides, the exponent ns (0.38) which was below 0.5, implying that experimental data were better represented by Freundlich model compared with Langmuir model. A low value of R2 (0.9137) showed a low degree of fitness by D–R isotherm model on experimental data. The maximum phosphate adsorption capacity calculated by using Freundlich and Slips model were 19.88 mg-P per g and 19.54 mg-P per g, respectively.
Table 2 The isotherm model parameters of phosphate adsorption on hydrogel beads at 35 °C and pH = 3
Adsorption isotherm |
Parameter |
Langmuir |
Kl |
0.07348 |
qm |
19.68796 |
R2 |
0.9545 |
Freundlich |
Kf |
4.62814 |
nf |
0.28477 |
R2 |
0.9950 |
Slips |
Ks |
4.50162 |
as |
0.08861 |
ns |
0.38182 |
R2 |
0.9967 |
D–R |
qDR |
18.4542 |
β |
0.00158 |
R2 |
0.9137 |
Regeneration
Reusability is a key factor in evaluating the potential practical application of adsorbents. Therefore, adsorption–desorption batch experiments were conducted consecutively five times to evaluate the recycling of hydrogel beads. As shown in Fig. 11, the adsorption capacity were 14.41 mg-P per g, 13.91 mg-P per g, 13.98 mg-P per g, 14.40 mg-P per g and 14.16 mg-P per g from first to fifth recycling experiment. Compared with the adsorption capacity of original hydrogel beads (16.51 mg-P per g), the adsorption capacity decreased by about 15%, and stayed at a relatively stable level. This indicates that PNIPAM/AA hydrogel beads exhibit reusability and maybe a promising phosphate adsorption adsorbent in the future.
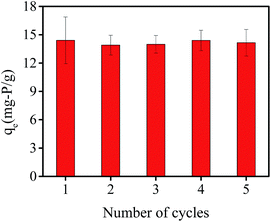 |
| Fig. 11 Influence of desorption cycles on equilibrium phosphate adsorption capacity. | |
Column adsorption and regeneration experiment
The column breakthrough study results are shown in Fig. 12. A widely used Thomas model was used to analyze the column adsorption experimental data. The equation of Thomas model34 is listed as follows: |
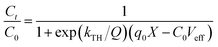 | (9) |
where kTH is the Thomas rate constant (mL mg−1 min−1), q0 is the maximum solid-phase concentration of the solute (mg g−1), Veff stands for the effluent volume (mL), X is the mass of adsorbent (g), and Q is the flow rate (mL min−1).
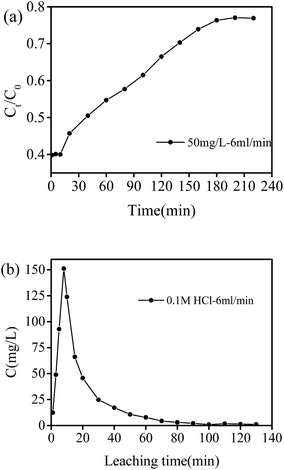 |
| Fig. 12 Phosphate adsorption experiment in fixed-bed column: (a) phosphate breakthrough curve, and (b) phosphate desorption with 0.1 M HCl. | |
As shown in Fig. 12(a), the breakthrough curve exhibited a typical S-shaped curve, and the breakthrough time was about 180 min. The fitting results are shown in Table 3, and the breakthrough curve data was well fitted by Thomas model with R2 = 0.984. The obtained kTH and qTH values were 0.000168 mL mg−1 min−1 and 4515.6 mg g−1, respectively. Fig. 12(b) illustrates the recycling behavior of PNIPAM/AA hydrogels in a flow mode. Concentration of phosphate in effluent reached a high as 151.14 mg L−1 and the decreased to be as low as 0.89 mg L−1 after leaching of 100 min. These results indicated that PNIPAM/AA got strong phosphate adsorption capacity, and can be used as an adsorption material in flowing water for phosphate removal and recycling as well.
Table 3 Parameters of column adsorption for phosphate on hydrogel beads
C0 (mg L−1) |
Flow rate (mL min−1) |
Thomas model |
R2 |
kTH (mL mg−1 min−1) |
qTH (mg g−1) |
50 |
2 |
0.000168 |
4515.6 |
0.984 |
Conclusions
In sum, PNIIPAM/AA IPN hydrogel beads were prepared and applied for phosphate adsorption. The adsorbent exhibited a relatively high adsorption capacity due to the introduction of trivalent aluminum ions. In addition, effect of pH, adsorbents dosage and co-existing anions were investigated and it was found that the maximum adsorption capacity reached at pH = 3, the adsorption capacity decreased with adsorbent dosage increasing, and SO42− had much more negative effects on phosphate adsorption that Cl− and NO3−. Furthermore, results of adsorption kinetics indicated that chemical adsorption and intra-particle diffusion were rate-controlling steps, and results of adsorption isotherms suggested that heterogeneous multilayer adsorption was dominant during adsorption process. Besides, the regeneration study demonstrated the generated hydrogel beads could be reused for a few cycles. More importantly, column adsorption experiment showed PNIPAM/AA hydrogel possessed strong phosphate adsorption capacity in flowing water. However, more further work are needed to improve the performance of adsorbent, such as increasing the adsorbent capacity combined with other multivalent metal ions or graphene, and improving the selectivity of hydrogel for phosphate adsorption and recycle by introducing ion-imprinted technique. This work have studied the phosphate adsorption behavior and mechanisms by PNIPAM/AA IPN hydrogel, indicating it is a nice adsorbent and possesses the potential in a large scale phosphate removal and recycle application. It will also broaden the application of IPN hydrogels in water treatment and phosphate recycles.
Acknowledgements
We would like to thank the Natural Science Foundation of China (NSFC, No. 51308239) and Natural Science Foundation of Hubei Province (No. 2015CFB276) for the financial support. We also thank the Analytical and Testing Center of Huazhong University of Science and Technology for related analysis.
Notes and references
- B. E. Rittmann, B. Mayer, P. Westerhoff and M. Edwards, Chemosphere, 2011, 84, 846–853 CrossRef CAS PubMed.
- L. Zhang, S. Zhang, S. Wang, C. Wu, Y. Chen, Y. Wang and Y. Peng, Chemosphere, 2013, 91, 635–640 CrossRef CAS PubMed.
- D. Mohan, A. Sarswat, Y. S. Ok and C. U. Pittman, Bioresour. Technol., 2014, 160, 191–202 CrossRef CAS PubMed.
- Y. Yang, J. Lohwacharin and S. Takizawa, Water Res., 2014, 65, 177–185 CrossRef CAS PubMed.
- W. Chouyyok, R. J. Wiacek, K. Pattamakomsan, T. Sangvanich, R. M. Grudzien, G. E. Fryxell and W. Yantasee, Environ. Sci. Technol., 2010, 44, 3073–3078 CrossRef CAS PubMed.
- S. Vasudevan and J. Lakshmi, RSC Adv., 2012, 2, 5234–5242 RSC.
- J. Liu, Q. Zhou, J. Chen, L. Zhang and N. Chang, Chem. Eng. J., 2013, 215, 859–867 CrossRef.
- Y. Su, H. Cui, Q. Li, S. Gao and J. K. Shang, Water Res., 2013, 47, 5018–5026 CrossRef CAS PubMed.
- L.-g. Yan, K. Yang, R.-r. Shan, T. Yan, J. Wei, S.-j. Yu, H.-q. Yu and B. Du, J. Colloid Interface Sci., 2015, 448, 508–516 CrossRef CAS PubMed.
- G. Li, S. Gao, G. Zhang and X. Zhang, Chem. Eng. J., 2014, 235, 124–131 CrossRef CAS.
- T. S. Anirudhan, T. A. Rauf and S. R. Rejeena, Desalination, 2012, 285, 277–284 CrossRef CAS.
- J. Zhao, Z. Wang, J. C. White and B. Xing, Environ. Sci. Technol., 2014, 48, 9995–10009 CrossRef CAS PubMed.
- G. Zhou, J. Luo, C. Liu, L. Chu, J. Ma, Y. Tang, Z. Zeng and S. Luo, Water Res., 2016, 89, 151–160 CrossRef CAS PubMed.
- G. Jing, L. Wang, H. Yu, W. A. Amer and L. Zhang, Colloids Surf., A, 2013, 416, 86–94 CrossRef.
- S. E. Kudaibergenov and A. Ciferri, Macromol. Rapid Commun., 2007, 28, 1969–1986 CrossRef CAS.
- E. S. Dragan, Chem. Eng. J., 2014, 243, 572–590 CrossRef CAS.
- B. Hui, Y. Zhang and L. Ye, Chem. Eng. J., 2014, 235, 207–214 CrossRef CAS.
- J. Dai, H. Yang, H. Yan, Y. Shangguan, Q. Zheng and R. Cheng, Chem. Eng. J., 2011, 166, 970–977 CrossRef CAS.
- M. S. Lencina, N. A. Andreucetti, C. G. Gómez and M. A. Villar, in Advances in Natural Polymers, Springer, 2013, pp. 193–254 Search PubMed.
- D. Leal, W. De Borggraeve, M. V. Encinas, B. Matsuhiro and R. Müller, Carbohydr. Polym., 2013, 92, 157–166 CrossRef CAS PubMed.
- H. Luo, Q. Wang, T. Tao, T. C. Zhang and A. Zhou, J. Environ. Eng., 2014, 140, 04014044, DOI:10.1061/(asce)ee.1943-7870.0000875.
- A. Zhou, H. Luo, Q. Wang, L. Chen, T. C. Zhang and T. Tao, RSC Adv., 2015, 5, 15359–15365 RSC.
- J. H. Choi, H. Y. Lee and J. C. Kim, J. Appl. Polym. Sci., 2008, 110, 117–123 CrossRef CAS.
- State Environmental Protection Administration of China (SEPAC), Monitoring and analysis methods of water and wastewater, China Environmental Science Press, Beijing, 4th edn, 2002 Search PubMed.
- R. P. Dumitriu, G. R. Mitchell and C. Vasile, Polym. Int., 2011, 60, 222–233 CrossRef CAS.
- Q. Zhou, X. Lin, B. Li and X. Luo, Chem. Eng. J., 2014, 256, 306–315 CrossRef CAS.
- S.-Y. Yoon, C.-G. Lee, J.-A. Park, J.-H. Kim, S.-B. Kim, S.-H. Lee and J.-W. Choi, Chem. Eng. J., 2014, 236, 341–347 CrossRef CAS.
- J. Zhang, W. Shan, J. Ge, Z. Shen, Y. Lei and W. Wang, J. Environ. Eng., 2011, 138, 252–258 CrossRef.
- X. Liu and L. Zhang, Powder Technol., 2015, 277, 112–119 CrossRef CAS.
- Y.-S. Ho, J. Hazard. Mater., 2006, 136, 681–689 CrossRef CAS PubMed.
- Y. Ho and G. McKay, Process Saf. Environ. Prot., 1998, 76, 332–340 CrossRef CAS.
- W. J. Weber and J. C. Morris, J. Sanit. Eng. Div., Am. Soc. Civ. Eng., 1963, 89, 31–60 Search PubMed.
- K. Foo and B. Hameed, Chem. Eng. J., 2010, 156, 2–10 CrossRef CAS.
- X. Li, Y. Qi, Y. Li, Y. Zhang, X. He and Y. Wang, Bioresour. Technol., 2013, 142, 611–619 CrossRef CAS PubMed.
|
This journal is © The Royal Society of Chemistry 2016 |