DOI:
10.1039/C5RA24328A
(Paper)
RSC Adv., 2016,
6, 11631-11636
Equilibrium and kinetic studies on MB adsorption by ultrathin 2D MoS2 nanosheets†
Received
17th November 2015
, Accepted 14th January 2016
First published on 18th January 2016
Abstract
MoS2 sheets, graphene-like two-dimensional materials, show application potential in optoelectronics and biomedicine due to their unique properties. However, the environmental influence of such 2D materials remains to be unveiled. Here we report that MoS2 ultrathin nanosheets synthesized by a hydrothermal process display remarkable selective removal of Methylene Blue (MB) from aqueous solution, showing the maximum adsorption capacity reached up to 146.43 mg g−1 within only 300 seconds. The kinetics and equilibrium of the adsorption process were found to follow the pseudo-second-order kinetic and Freundlich isotherm models, respectively. More importantly, MoS2 nanosheets can be readily cleaned for reuse by washing with deionized water. This work demonstrates that the resulting MoS2 nanosheets may be considered as highly efficient and green adsorbents for eliminating MB from aqueous solution.
Introduction
Currently, global water contamination has become one of the serious environmental problems and has attracted increasing public concern with the development of industrialization and urbanization.1,2 In particular, different organic dyes mainly discharged from industrial processes may bring detrimental effects to human health and animals. Therefore, various technologies including adsorption, photo-degradation, chemical oxidation and electrochemical processes3–7 have been studied and applied to remove dyes from aqueous systems. Among all the proposed techniques, the adsorption process as an effective, economical and convenient approach is extensively employed in water treatment, in which a variety of materials have been studied as adsorbents, such as activated carbon, zeolites, clays and polymers.8–11 Nevertheless, these adsorbents normally suffer from either low adsorption rates or undesirable regeneration. Therefore, it is of great significance to design and seek new generation adsorbents with high efficiency and durability.
It has been recently reported that sheet-like inorganic layered-compounds have potential advantages in dye removal applications due to their large specific surface area and the increased interfacial area between adsorbates and adsorbents, structural tunability, good chemical stability and well-defined structure. For example, Portehault et al.12 synthesized highly porous layered BN nanosheets with superior adsorption performance for MB, solvents and oils. Zeng et al.13,14 reported the hydrothermal synthesis of hierarchical three-dimensional (3D) porous BiOI and NiO with lamellar structures and demonstrated such architectures have strong adsorption capability for organic pollutants in wastewater. Wong et al.15 prepared layered protonated titanate nanosheets (LPTN) with extremely large surface area, displaying excellent removal ability for MB and Pb2+. Therefore, investigation on sheet-like inorganic layered-compounds, which are applied as adsorbents should be paid increasing attention.
Transition metal sulfide MoS2, with a layered structure consisting of covalently bonded S–Mo–S, has experienced a rapid growth and been found attractive in many applications. For example, the weak van der Waals interactions between layers facilitate the intercalation/deintercalation of lithium ions without initiating large volume expansion, which make MoS2 nanosheets as anode for lithium ion batteries.16 The few-layer/single-layer MoS2 as potential alternatives to Pt-based catalysts in hydrogen evolution reaction have attracted enormous research interest.17,18 Also, single-layer MoS2 nanosheets as phototransistor19 and sensors20 were also reported. Be inspired by the excellent adsorption performance of two-dimensional (2D) lamellar materials, MoS2 is expected to be used as a novel adsorbent. In this paper, we synthesized the MoS2 ultrathin nanosheets through the one-step hydrothermal method and first studied the adsorption performance in the whole scope of the absorbing process, including capacity, kinetics, recovery, regeneration and cycling. The results show that the obtained fewlayer MoS2 show excellent adsorption performance towards MB dyes. We also demonstrates that the saturated materials can be cleaned for reuse by simply washing, which further demonstrates the great application prospects of MoS2 nanosheets for removing organic dyes from aqueous solution.
Experimental
Synthesis of MoS2 nanosheets
Hexaammonium heptamolybda tetetrahydrate ((NH4)6Mo7O24·4H2O) and thiourea (CH4N2S) were purchased from Sigma-Aldrich. Methylene blue (MB), Rhodamine B (RhB) and Methylene Orange (MO) were purchased from Tianjin Kermel Chemical Reagent Co. Ltd. All of the reagents employed in this study were of analytical grade and used as received, without any further purification. Distilled water was used in all of the experiments. MoS2 ultrathin nanosheets were synthesized by a convenient and controllable hydrothermal procedure. In a typical synthesis process, 1 mmol of hexaammonium heptamolybda tetetrahydrate ((NH4)6Mo7O24·4H2O) was dissolved in 35 mL deionized water. After stirring for 5 minutes at room temperature, 30 mmol of thiourea (CH4N2S) was added to the above solution with stirring to form wathet blue homogeneous solution. The mixture was stirred for 30 minutes at room temperature and transferred into a 50 mL Tefion-lined stainless steel autoclave. The autoclave was sealed and maintained at 160 °C for 24 hours. After the solution was cooled to room temperature naturally, the obtained black products were collected by centrifugation with a speed of 8000 rpm, washed three times with deionized water and ethanol alternatively, and then dried at 60 °C under air for 8 h.
Materials characterizations
The crystal structure of the products were characterized by X-ray diffraction (XRD, Rigaku Ultima IV) with Cu Kα radiation (λ = 0.15418 nm). Full diffraction patterns were taken in the 2θ range from 5° to 80° in a step model with a 0.02° step size. The morphologies and structures of the samples were characterized by field emission scanning electron microscopy (FESEM, JEOL-JSM-7500F; accelerating voltage: 10 kV) equipped with energy dispersive X-ray spectroscopy (EDS) and transmission electron microscopic (TEM, JEOL-JEM-2100F, accelerating voltage: 200 kV). X-ray photoelectron spectroscopic (XPS) was performed using an ESCALAB 250 with a monochromatic Al Kα X-ray source. The Brunauer–Emmett–Teller (BET) specific surface area was determined via N2 adsorption using Gold APP V-sorb 2800 system at the liquid nitrogen temperature of 77 K. The pore size distribution was calculated from the desorption branch of the nitrogen isotherm by the Barrett–Joyner–Halenda (BJH) method. UV-vis spectrophotometer (Shimadzu UV-vis 2550 spectrophotometer, Japan) with an integrating sphere using BaSO4 as the reference was used for determination of concentration of MB, RhB and CR. The surface charge of the nanoparticles was determined through zeta-potential measurements using a ZetaSizer Nano ZS-90 (ZEN 3690, Malvern Instruments Inc., UK) with a HeNe light source (632 nm) and all data were averaged over three measurements. All pH measurements were carried out with an ISTEK-720P pH meter.
Adsorption experiments
In this study, we evaluate the selective adsorption experiments of the as-prepared MoS2 using three model pollutant dyes, namely, MB, RhB and MO. The maximum absorption peak of MB, RhB and MO at 664 nm, 553 nm, 463 nm were used to measure the concentration of dyes in the solution. All experiments were conducted at 25 °C. 5 mg of the MoS2 sample was added to 20 mL of MB aqueous solution with a fixed concentration under stirring in dark condition. The concentrations of dyes left in supernatant solutions after different time intervals (10–300 s) were determined using a UV-vis spectrophotometer at λmax. The effect of pH was studied by adjusting the pH of the dye solution in the range of 1–14 with 0.1 M NaOH or HCl solution. The amount of MB adsorbed per unit mass of MoS2 nanosheets (qt) at equilibrium was calculated by: |
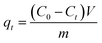 | (1) |
where qt (mg g−1) is the amount adsorbed per gram of adsorbent at time t (min), C0 is the initial concentration of MB in the solution (mg L−1), Ct is the concentration of MB at time t of adsorption (mg L−1), m is the total addition amount of the MoS2 sample (g), and V is the volume of pollutant solution (L).
The percent removal of dyes by the hereby adsorbent is given by:
|
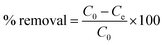 | (2) |
where
C0,
Ce is denoted the initial and equilibrium concentration (mg L
−1) of the dyes, respectively.
The effect of pH was performed by mixing 5 mg of MoS2 nanosheets into 20 mL MB solution of 20 mg L−1 in the condition of 25 °C and different initial pH of MB solutions. The initial pH of MB solution was adjusted to values in the range of 1–14 by dropwise adding 0.1 mol L−1 NaOH or 0.1 mol L−1 HCl solutions. To prepare the samples for these measurements, MoS2 (0.6 mg) were deagglomerated in 20 mL of purified water by sonicating for 20 min using an ultrasonic bath. Then 0.5 mL of the MoS2 suspension was added to 1.5 mL of aqueous solutions with different pH values and sonicated for an extra 5 min. The solutions were measured in a folded capillary cell (DTS1060) made from polycarbonate with goldplated beryllium/copper electrodes. After 300 s of contact time, the concentrations of MB left in supernatant solutions were determined to study the effect of pH. Effects of contact time and initial MB concentration were conducted at different MB concentrations, and other conditions were the same as kinetic experiments.
Results and discussion
Characterization of the adsorbent
The products obtained by hydrothermal method was characterized by powder X-ray diffraction (XRD). All the diffraction peaks (Fig. 1a) were readily indexed to hexagonal MoS2 (JCPDS no. 73-1508). No signals from other impurities were observed. Compared with pristine MoS2, the (002) diffraction peak of MoS2 nanosheet was slightly shift to a lower 2θ value, illustrating an enlarged interlayer spacing. The space distance (d) of 0.67 nm, calculated from Bragg's equation, is larger than that of 0.62 nm in pristine hexagonal MoS2, which may be caused by the oxygen incorporation.21 It is noteworthy that the diffraction peaks are weak, probably because the crystallinity of MoS2 nanosheets is poor. Moreover, XRD pattern of the MoS2 obtained at 220 °C (Fig. S1†) was better crystallized and the result confirmed that pure MoS2 can be obtained under our experiment condition.
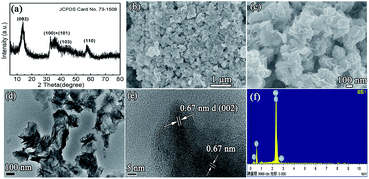 |
| Fig. 1 (a) XRD pattern of MoS2 nanosheets; (b, c) FESEM images and (d, e) TEM and HRTEM images of MoS2 nanosheets; (f) EDS spectra of MoS2 nanosheets. | |
The morphology of synthesized MoS2 was observed via FESEM and TEM. The SEM images (Fig. 1b and c) clearly show that the obtained MoS2 is of ultrathin nanosheet morphology with uniform lateral size in the range of 100–200 nm. The typical EDS pattern of MoS2 nanosheets (Fig. 1f) reveals the presence of Mo and S with the atomic ratio of Mo/S is about 1
:
1.92, which is very close to that of the stoichiometric MoS2. The elemental of C may come from the contaminants adsorbed on the sample, while the small portion of O may be caused by the partial oxidation of the MoS2. TEM image in Fig. 1d illustrates the MoS2 nanosheets are made of crumpled nanosheets with plenty of folded edges. A typical high resolution TEM (HRTEM) image of MoS2 nanosheets is shown in Fig. 1e. The periodic fringe spacing of ∼0.67 nm corresponds to interplanar spacing between the (002) planes of MoS2, which agrees well with the XRD result.
Fig. 2 shows the nitrogen adsorption–desorption isotherms and the pore size distribution curve of MoS2. The isotherm is of type IV (BDDT classification) with a hysteresis loop in the range of (0.4 to 1.0)P/P0, which indicates pore size distributions in the mesoporous region. The specific surface area of the MoS2 nanosheets, calculated by the multipoint BET method, was 15.09 m2 g−1. The corresponding pore size distribution, calculated from the desorption branch of nitrogen isotherm (Fig. 2, inset), shows a relatively wide range of mesopores. These mesoporous are likely caused by the connection of MoS2 ultrathin nanosheets, which might be beneficial to improve the adsorption capacity and efficiency for dye molecules.12,22
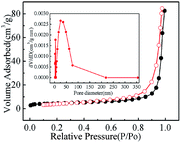 |
| Fig. 2 Nitrogen adsorption–desorption isotherms and pore size distribution (inset) of MoS2. | |
X-ray photoelectron spectroscopy (XPS) was carried out to investigate the surface chemical state and composition of MoS2 nanosheets The binding energies obtained from the XPS analysis were corrected for specimen charging by referencing the C 1s line to 284.5 eV. The survey XPS spectrum (Fig. 3a) for product reveals that the nanosheets are composed of Mo, S, and O. The high-resolution XPS spectrum of Mo 3d was shown in Fig. 3b. Two peaks at 231.6 eV and 228.6 eV can be assigned to Mo 3d3/2, Mo 3d5/2, respectively, and ascribed to Mo (+4) in 1T-MoS2.21 The doublet peaks centred at about 229.2 and 232.6 eV may derive form the 3d5/2 and 3d3/2 of Mo4+ in 2H–MoS2.23 The relatively weak peaks located at 232.2 and 235.9 eV are assigned to Mo 3d peaks with a higher oxidation state (Mo6+), which may originate form the partial oxidation of Mo atoms at the edges or defects on the crystal plane of the MoS2 nanosheets.24,25 The peak centred at 226.7 eV is arising from S2s in MoS2. The S 2p3/2 and S 2p1/2 peaks with a typical spin–orbit doublet of 1.3 eV were at 161.5 and 162.8 eV, respectively, which are characteristic of S2p in MoS2 (Fig. 3c). The high-resolution XPS spectrum of O 1s (Fig. 3d) is wide and asymmetric, implying that more than one type of O chemical state exists in the product. The deconvolution of the O 1s spectrum indicates two contributions of lattice oxygen O2− (in the stronger Mo–O bond) at the lower binding energy of 531.6 and surface adsorbed oxygen (–OH group and chemisorbed oxygen-containing species) at a higher bonding energy of 533.5 eV.
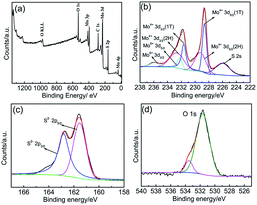 |
| Fig. 3 (a) XPS survey spectra of MoS2 and high-resolution scans of (b) Mo; (c) S and (d) O. | |
Adsorption kinetics and equilibrium studies
To date, most of the reported porous or layered adsorbents exhibit excellent absorbencies for removal of organic dyes, oil, solvents and metal ions from water.12,13,15 However, the selectivity is more attractive and challenging for organic dyes adsorption. Combined with the aforementioned characterization, the MoS2 nanosheets were explored as a potential adsorbent for water treatment. The adsorption behaviors of the MoS2 nanosheets towards organic dyes, including MB, RhB and MO, were performed and the results were shown in Fig. 4a. Obviously, the MoS2 nanosheets exhibited distinct selectivity towards MB with a removal percentage of 98.49% in 300 seconds, while the value for RhB and MO are 76.89% and 34.5%, respectively. It suggests MoS2 nanosheets can be a high-efficiency adsorbent for the adsorption of MB. The preferential adsorption for MB may be related to the electrostatic attraction and π–π stacking interactions between MB and the MoS2 nanosheets.22
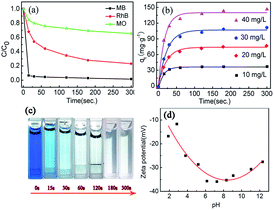 |
| Fig. 4 (a) The selective adsorption for MB, RhB and MO; (b) the effect of contact time on the adsorption capacity of MB onto MoS2 nanosheets at different initial concentrations; (c) photo image of absorption of MB by MoS2 ultrathin nanosheets at different times; (d) zeta potentials of the MoS2 nanosheets at different pH values. | |
So we select MB as the model dye to study the adsorption performance of MoS2 nanosheets. Fig. 4b shows the time-dependent adsorption performance of MoS2 nanosheets towards MB at different initial concentrations. It can be seen that the initial adsorption rates were very fast during the initial stage for all the studied concentrations. For instance, the as-prepared MoS2 could remove more than 85% of the MB within 120 seconds at different concentrations. This high efficiency in adsorption phenomenon can be ascribed to the abundant active sites on the adsorbent's surface and the electrostatic attraction between MoS2 and MB. The adsorption process then proceeds slowly and takes only about 300 seconds to reach an adsorption equilibrium state. As for we all know, the adsorption speed is the highest among the existing adsorbents. The maximum adsorption capacity of the MoS2 nanosheets towards MB is ca. 146.43 mg g−1 (Table S1†), which is much higher than other previously reported materials such as MCGO,26 GO,26 activated charcoal,27 graphene,28 polydopamine microspheres29 and PZS nanotubes.30 It has also shown that the adsorption capacity of MoS2 nanosheets enhanced with the increasing of the initial MB concentrations (Fig. 4b), which can be explained by the fact that the initial dye concentrations provide an important driving force to overcome the mass transfer resistance of the dye between the aqueous phases and the solid phases.13,14 Therefore, increasing initial concentrations is favorable to increase the adsorption capacity.
The degree of adsorption of dyes onto the adsorbent surface is primarily influenced by the surface charge on the adsorbent, which in turn is influenced by the solution pH. As shown in Fig. S2,† the adsorption capacities of MB on the MoS2 nanosheets present a significant increasing trend with the increase of pH values in the range of 1–14, suggesting basic solutions benefit MB adsorption. The zeta potential of MoS2 nanosheets, displayed in Fig. 4d, can demonstrate the changes of surface charge of the adsorbent. The surface charge (zeta potential) of the MoS2 nanosheets indicates that they are highly negatively charged when dispersed in water with various pH values, which can form stable MoS2 dispersions. As surface charge density increases with an increase in the solution pH, the electrostatic attraction between the positively charged dye (MB) and the surface of the MoS2 is increased, which may result in an increase in the rate of adsorption. Similar trends were reported in the literature for the adsorption of basic dyes methylene blue onto polydopamine microspheres,29 PZS nanotubes30 and carbon.31
Adsorption kinetics
Adsorption kinetic studies are thoroughly studied due to their importance on the investigation of the adsorption rate and mechanism. The pseudo-first-order and second-order kinetic model (Fig. 5) were used to research the characteristics of the adsorption process. The differential and integral forms of the pseudo-first order model can be written as:32,33 |
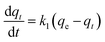 | (3) |
|
ln(qe − qt) = ln qe − k1t
| (4) |
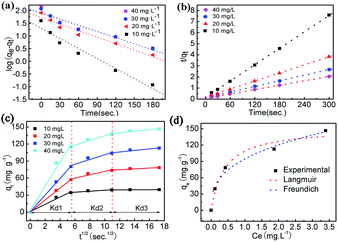 |
| Fig. 5 Pseudo-first-order (a) and pseudo-second-order (b) kinetics plots of MB adsorption onto MoS2 nanosheets. (c) Intraparticle diffusion kinetics plots of MB adsorption onto MoS2 nanosheets; (d) adsorption isotherm curves for the adsorption of MB onto MoS2 nanosheets. | |
The pseudo-second-order model can be expressed as:
|
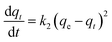 | (5) |
|
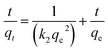 | (6) |
where
k1 and
k2 are the first-order and second-order rate constants for the adsorption process, respectively;
qt is the amount of MB adsorbed (mg g
−1) at various time
t,
qe is the maximum adsorption capacity and
t is the adsorption time (s).
All kinetic parameters including correlation coefficients (R2), k1, k2 and calculated qe,cal values are determined by linear regression and the results are given in Table S2.† The linearity of the plots (R2 > 0.99), as well as the good agreement between calculated qe and the experimental values, suggest the adsorption of MB onto MoS2 nanosheets can be well-fitted by the pseudo-second-order kinetic model. In addition, the pseudo-second-order rate constants (k2) values decreased from 0.0043 to 0.0006 g mg−1 s−1 with increasing initial MB concentrations, which can be ascribed to the lower competition for the adsorption surface sites at lower MB concentration.22,34 Moreover, the equilibrium adsorption capacity increased with the increase of initial MB concentration and that is caused by the relatively strong driving force at high initial concentrations.
In order to further study the diffusion mechanism of MB onto MoS2 nanosheets, Weber's intra-particle diffusion model was used to analyze the kinetic data, which was presented as follows:35
where
Ci is a constant (mg g
−1),
ki is the diffusion rate of stage i (mg g
−1 s
−1/2). The influence of the boundary layer to the adsorption process can be described by constant
C. If the adsorption process included intraparticle diffusion, the plots of
qt versus the
t1/2 should be linear and if the line passes through the origin, then the rate-limiting process is the intra-particle diffusion.
36
As shown in Fig. 5c, the qt versus t1/2 plot at different intial MB concentrations indicates that there are three reaction steps in the process of MB onto MoS2 nanosheets. Firstly, the sharper linear region was a diffusion adsorption process, corresponding to the diffusion of MB through the solution to the external surface of MoS2 nanosheets. Secondly, the adjacent region was a gradual or slow adsorption stage where intra-particle diffusion was the rate limiting step. Finally, the third part was the final equilibrium stage in which the low concentrations of MB left in solutions lead to slower intra-particle diffusion. The ki,1 value shown in Table S3† is much larger than ki,2 and ki,3 values, suggesting the first diffusion adsorption step was the most important one for the adsorption of MB onto MoS2 nanosheets.14,37 During the adsorption process, MB molecules were firstly adsorbed by the exterior surface of MoS2 nanosheets, and then the MB molecules entered the pores between the nanosheets and subsequently adsorbed when adsorption at the exterior surface reached the equilibration. The increasing of diffusion resistance in the interior adsorption process could be reasonably expected to explain the decrease in diffusion rate.38
Adsorption isotherm
The adsorption isotherm indicates how the adsorption molecules distribute between the liquid phase and the solid phase when the adsorption process reaches an equilibrium state. Adsorption equilibrium is a dynamic concept achieved as the rate of dye adsorption is equal to the desorption rate. The experimental equilibrium data of MB adsorption onto MoS2 nanosheets are fitted to Langmuir and Freundlich models. The Langmuir model is based on the assumption that adsorption is localized on a monolayer and all adsorption sites at the adsorbent are homogeneous. Whereas the Freundlich isotherm presumes that the multilayer of the adsorption process occurs on a heterogeneous surface. The linear form of Langmuir and Freundlich isotherms are expressed as follows:39,40 |
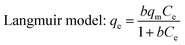 | (8) |
|
Freundlich model: qe = KfCe1/n
| (9) |
where constant b is Langmuir affinity constant (L mg−1), qm is the maximum adsorption capacity (mg g−1), Kf is roughly an indicator of the adsorption capacity, and 1/n is the Freundlich exponent (dimensionless).
Fig. 5d shows the isotherms of MB onto MoS2 nanosheets and the equilibrium adsorption characteristics were analyzed using the Langmuir and Freundlich isotherm models. The corresponding parameters and correlation coefficients are summarized in Table S4.† The R2 (0.9798) of Freundlich model is larger than the Langmuir model (0.9780), indicating the multilayer adsorption of MB onto MoS2 nanosheets.41 In addition, the value of the Freundlich exponent n was larger than 1, representing favorable adsorption condition.
As is well known, a promising adsorbent should not only possess higher adsorption capacity but also good stability and reusability, which will greatly reduce the cost, and also is crucial for water treatment application. Thus, the recycling of MoS2 nanosheets for MB removal was studied. As can be seen in Fig. 6, the MoS2 nanosheets exhibit good stability and retain more than 80% removal efficiency in four successive cycles. More importantly, the MB-adsorbed MoS2 nanosheets could be regenerated simply by washing with deionized water. Therefore, MoS2 nanosheets with excellent adsorption performance, stability and regeneration ability can be extended to environmental application for wastewater treatment.
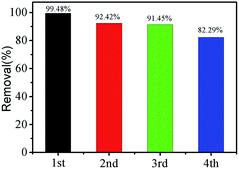 |
| Fig. 6 Removal efficiency of MB onto MoS2 nanosheets in four successive cycles. | |
Conclusion
In summary, MoS2 ultrathin nanosheets have been successfully synthesized through a simple hydrothermal method, which could be used as an efficient, robust and recyclable adsorbent for water treatment application. The obtained MoS2 exhibits super performance for the rapid and efficient removal of MB from aqueous solution, with a maximum adsorption capacity of 146.43 mg g−1 in 300 seconds. The kinetics and isotherm of adsorption process were found to obey the pseudo-second-order kinetics and Freundlich isotherm model, respectively. Furthermore, MoS2 adsorbent can retain the high removal efficiency in four successive cycles by simply washing. Thus, the present MoS2 nanosheets could be explored as an exceptionally promising candidate for environmental remediation.
Acknowledgements
This work was financially supported by the NSF of China (No. 21373122, 51572152 and 51502155), the Research Project of Hubei Provincial Department of Education (No. D20151203), the NSF of Hubei Province of China (No. 2011CDA118 and D20151203), the Scientific Research Foundation for the Introduction of Talent of China Three Gorges University (No. KJ2014B008).
References
- B. Royer, N. Cardoso, E. Lima, V. Ruiz, T. Macedo and C. Airoldi, J. Colloid Interface Sci., 2009, 336, 398–405 CrossRef CAS PubMed.
- R. Q. Long and R. T. Yang, J. Am. Chem. Soc., 2001, 123, 2058–2059 CrossRef CAS PubMed.
- A. Bayat, S. F. Aghamiri, A. Moheb and G. R. Vakili-Nezhaad, Chem. Eng. Technol., 2005, 28, 1525–1528 CrossRef CAS.
- C. Han, Y. D. Wang, Y. P. Lei, B. Wang, N. Wu, Q. Shi and Q. Li, Nano Res., 2015, 8, 1199–1209 CrossRef CAS.
- K. Dutta, S. Mukhopadhyay, S. Bhattacharjee and B. Chaudhuri, J. Hazard. Mater., 2001, 84, 57–71 CrossRef CAS PubMed.
- G. S. Wu, J. P. Wang, D. F. Thomas and A. C. Chen, Langmuir, 2008, 24, 3503–3509 CrossRef CAS PubMed.
- C. A. Martínez-Huitle and E. Brillas, Appl. Catal., B, 2009, 87, 105–145 CrossRef.
- T. Depci, A. R. Kul and Y. Önal, Chem. Eng. J., 2012, 200–202, 224–236 CrossRef CAS.
- E. Alver and A. Ü. Metin, Chem. Eng. J., 2012, 200–202, 59–67 CrossRef CAS.
- W. S. Tan and A. S. Y. Ting, Bioresour. Technol., 2014, 160, 115–118 CrossRef CAS PubMed.
- T. S. Anirudhan and A. R. Tharun, Chem. Eng. J., 2012, 181–182, 761–769 CrossRef CAS.
- W. W. Lei, D. Portehault, S. Qin and Y. Chen, Nat. Commun., 2013, 4, 1777–1784 CrossRef PubMed.
- L. H. Ai, Y. Zeng and J. Jiang, Chem. Eng. J., 2014, 235, 331–339 CrossRef CAS.
- L. H. Ai and Y. Zeng, Chem. Eng. J., 2013, 215–216, 269–278 CrossRef CAS.
- C. H. Lin, D. S. Wong and S. Y. Lu, ACS Appl. Mater. Interfaces, 2014, 6, 16669–16678 CAS.
- H. Y. Wang, B. Y. Wang, D. Wang, L. Lu, J. G. Wang and Q. C. Jiang, RSC Adv., 2015, 5, 58084–58090 RSC.
- X. Li, J. G. Yu, J. X. Low, Y. P. Fang, J. Xiao and X. B. Chen, J. Mater. Chem. A, 2015, 3, 2485–2534 CAS.
- J. Deng, W. T. Yuan, P. J. Ren, Y. Wang, D. H. Deng, Z. Zhang and X. H. Bao, RSC Adv., 2014, 4, 34733–34738 RSC.
- Z. Y. Yin, H. Li, H. Li, L. Jiang, Y. M. Shi, Y. H. Sun, G. Lu, Q. Zhang, X. D. Chen and H. Zhang, ACS Nano, 2012, 6, 74–80 CrossRef CAS PubMed.
- C. F. Zhu, Z. Y. Zeng, H. Li, F. Li, C. H. Fan and H. Zhang, J. Am. Chem. Soc., 2013, 135, 5998–6001 CrossRef CAS PubMed.
- J. F. Xie, J. J. Zhang, S. Li, F. B. Grote, X. D. Zhang, H. Zhang, R. X. Wang, Y. Lei, B. C. Pan and Y. Xie, J. Am. Chem. Soc., 2013, 135, 17881–17888 CrossRef CAS PubMed.
- A. X. Yan, S. Yao, Y. G. Li, Z. M. Zhang, Y. Lu, W. L. Chen and E. B. Wang, Chem.–Eur. J., 2014, 20, 6927–6933 CrossRef CAS PubMed.
- L. H. Yuwen, F. Xu, B. Xue, Z. M. Luo, Q. Zhang, B. Q. Bao, S. Su, L. X. Weng, W. Huang and L. H. Wang, Nanoscale, 2014, 6, 5762–5769 RSC.
- W. J. Zhou, D. M. Hou, Y. H. Sang, S. H. Yao, J. Zhou, G. Q. Li, L. G. Li, H. Liu and W. Chen, J. Mater. Chem. A, 2014, 2, 11358–11364 CAS.
- Y. M. Sun, X. L. Hu, W. Luo and Y. H. Huang, ACS Nano, 2011, 5, 7100–7107 CrossRef CAS PubMed.
- L. L. Fan, C. N. Luo, X. J. Li, F. G. Lu, H. M. Qiu and M. Sun, J. Hazard. Mater., 2012, 215–216, 272–279 CrossRef CAS PubMed.
- M. J. Iqbal and M. N. Ashiq, J. Hazard. Mater., 2007, 139, 57–66 CrossRef CAS PubMed.
- T. Wu, X. Cai, S. Tan, H. Li, J. Liu and W. D. Yang, Chem. Eng. J., 2011, 173, 144–149 CrossRef CAS.
- J. W. Fu, Z. H. Chen, M. H. Wang, S. J. Liu, J. H. Zhang, J. N. Zhang, R. P. Han and Q. Xu, Chem. Eng. J., 2015, 259, 53–61 CrossRef CAS.
- Z. H. Chen, J. N. Zhang, J. W. Fua, M. H. Wang, X. Z. Wang, R. P. Han and Q. Xu, J. Hazard. Mater., 2014, 273, 263–271 CrossRef CAS PubMed.
- S. Senthilkumaar, P. R. Varadarajan, K. Porkodi and C. V. Subbhuraam, J. Colloid Interface Sci., 2005, 284, 78–82 CrossRef CAS PubMed.
- F. J. Wu, W. Liu, J. L. Qiu, J. Z. Li, W. Y. Zhou, Y. P. Fang, S. T. Zhang and X. Li, Appl. Surf. Sci., 2015, 358, 425–435 CrossRef CAS.
- Q. S. Liu, T. Zheng, N. Li, P. Wang and G. Abulikemu, Appl. Surf. Sci., 2010, 256, 3309–3315 CrossRef CAS.
- W. J. Zhou, Z. Y. Yin, Y. P. Du, X. Huang, Z. Y. Zeng, Z. X. Fan, H. Liu, J. Y. Wang and H. Zhang, Small, 2013, 9, 140–147 CrossRef CAS PubMed.
- W. J. Weber and J. C. Morris, J. Sanit. Eng. Div., Am. Soc. Civ. Eng., 1963, 89, 31–60 Search PubMed.
- S. Ghorai, A. Sarkar, M. Sarkar, A. B. Panda, H. Schönherr and S. Pal, ACS Appl. Mater. Interfaces, 2014, 6, 4766–4777 CAS.
- B. Cheng, Y. Le, W. Q. Cai and J. G. Yu, J. Hazard. Mater., 2011, 185, 889–897 CrossRef CAS PubMed.
- I. Langmuir, J. Am. Chem. Soc., 1917, 39, 2221–2295 CrossRef.
- H. M. F. Freundlich, Z. Phys. Chem., 1906, 57, 385–471 CAS.
- L. H. Li, J. Xiao, P. Liu and G. W. Yang, Sci. Rep., 2015, 5, 9028–9034 CrossRef CAS PubMed.
- W. J. Liu, T. Yang, J. Xu, Q. Chen, C. Yao, S. X. Zuo, Y. Kong and C. Y. Fu, Environ. Prog. Sustainable Energy, 2015, 34, 437–444 CrossRef CAS.
Footnotes |
† Electronic supplementary information (ESI) available. See DOI: 10.1039/c5ra24328a |
‡ These authors contributed equally to the work. |
|
This journal is © The Royal Society of Chemistry 2016 |