DOI:
10.1039/C5RA23986A
(Paper)
RSC Adv., 2016,
6, 3748-3755
Photothermal and photodynamic therapy reagents based on rGO–C6H4–COOH†
Received
14th November 2015
, Accepted 11th December 2015
First published on 14th December 2015
Abstract
A photothermal therapy (PTT) and photodynamic therapy (PDT) reagent was synthesized based on rGO–C6H4–COOH (graphene diazotized). rGO–C6H4–COOH was first conjugated with polyethyleneimine (PEI) to enhance water solubility and then tetrakis(4-carboxyphenyl) porphyrin (TCPP) was further attached to obtain PDT ability for the system. The rGO–PEI–TCPP system exhibited excellent stability in various biological solutions and the cytotoxicity of this rGO–PEI–TCPP system was acceptable. The CBRH7919 cancer cells could be induced to effectively undergo apoptosis by rGO–PEI–TCPP under laser irradiation. The reason is mainly attributed to the excellent photothermal and photodynamic effects, i.e., the production of heat and singlet oxygen. At the same time, the CBRH7919 cancer cells could be pushed to the vulnerable G2 phase of the cell cycle, which is the most sensitive and susceptible stage to damage by radiation.
1. Introduction
Graphene has been applied widely to the field of nano-electronic devices,1,2 transparent conductors,3,4 and nanocomposites5 in the past few years. A new direction for graphene is in the field of photothermal therapy (PTT) and photodynamic therapy (PDT). A PTT based on graphene has been completely designed from graphene oxide (GO) and reduced graphene oxide (rGO), as the degree of conjugation of planar GO is negligible.6–22 Therefore, the effect of PTT would not be ideal. A rGO colloidal solution has the tendency to agglomerate irreversibly in the absence of a stabilizer, thus making further processing difficult.23 Photodynamic therapy (PDT) is a minimally invasive therapeutic modality approved for the treatment of cancers and non-oncological disorders. Extensive studies on the synthesis of various porphyrin derivatives have been reported.15,16,23 Photoirradiation with a specific wavelength to the porphyrin derivatives induces reactions generating radicals such as the hydroxyl (OH) and the peroxy (HO2) radicals, as well as non-radical, and highly reactive singlet oxygen (1O2). The main cytotoxicity agent in PDT is widely believed to be 1O2 and both direct and indirect evidence supports a prevalent role for 1O2 in the molecular processes initiated by PDT.24,25
Herein, the rGO–PEI–TCPP (rGO–C6H4–CO–NH–PEI–NH–OC–TCPP) system was synthesized. According to our previous study,26 the conjugation degree of rGO–C6H4–COOH is higher than GO. Therefore, the effect of PTT should be better than of GO, and at the same time, the TCPP molecule could be used in PDT. A schematic is shown in Scheme 1.
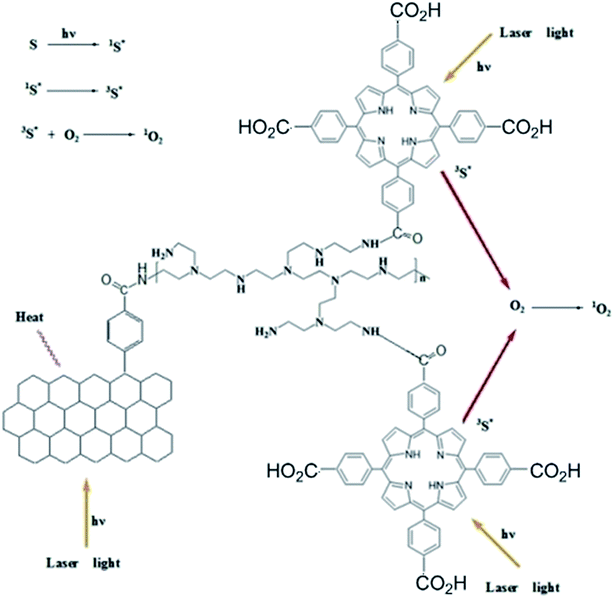 |
| Scheme 1 PTT and PDT illustration of the rGO–PEI–TCPP system. | |
2. Experimental
2.1 Synthesis of graphene oxide (GO), reduced graphene oxide (rGO) and rGO–C6H4–COOH
GO, rGO and rGO–C6H4–COOH were synthesized according to our previous method, and the detailed synthesis process can be observed in the ESI.†26 For FT-IR characterization, solid samples of rGO and rGO–C6H4–COOH were obtained through freeze-drying. The solutions of GO, rGO and rGO–C6H4–COOH were placed on a thin film of amorphous carbon deposited on a copper grid for TEM (JEM-1400) observation. One drop of rGO–C6H4–COOH solution was dropped onto the silicon wafer for AFM (Nanoscope IIIA, Veeco) imaging. The colloidal nature of the rGO and rGO–C6H4–COOH dispersions demonstrated the Tyndall effect, which suggests excellent water dispersability.
2.2 Synthesis of rGO–C6H4–CO–NH–PEI and rGO–C6H4–TCPP
rGO–C6H4–CO–NH–PEI was synthesized by means of 1-ethyl-3-(3-dimethylaminopropyl) carbodiimide (EDC) chemistry. In detail, branched polyethyleneimine (PEI; 1.2 g, Mr = 1.8 kDa, Alfa Aesar) was dissolved in water (10 mL) with stirring. Then, EDC·HCl (0.2 g) and N-hydroxysuccinimide (NHS; 0.15 g) were added to 60 mL aqueous solution of rGO–C6H4–COOH (the abovementioned rGO–C6H4–COOH solution without further treatment) with stirring for 3 h (pH 5.5–6). Finally, the abovementioned two solutions were mixed and stirred for 48 h, and then sonicated for 48 h with a 500 W sonicator. The mixture was dialyzed (molecular weight cutoff (MWCO): 7000 Da) against water for another 48 h, then centrifuged at 10
000 rpm for 10 min to remove any precipitates. A solid sample was obtained for FT-IR spectroscopy by lyophilization.
To conjugate TCPP to rGO–C6H4–CO–NH–PEI, TCPP was first activated by NHS. In detail, TCPP (2 mmol) was dissolved in anhydrous ethanol (20 mL). NHS (4 mmol) and dicyclohexylcarbodiimide (DCC, 4 mmol) were added. The mixture was stirred overnight at room temperature. Filtration was carried out to remove all precipitates. The mixture was mixed with the abovementioned aqueous solution of rGO–C6H4–CO–NH–PEI with stirring for 48 h to produce a rGO–PEI–TCPP (Scheme 1) solution. The mixture was then dialyzed against ethanol (MWCO: 3500 Da) for 48 h to remove the TCPP, and finally dialyzed against water for another 48 h. The rGO–PEI–TCPP solution was sonicated for 72 h using a 500 W sonicator to obtain a small-sized product. The solution was used directly for the determination of UV-Vis and fluorescent spectroscopy measurements. One drop of this rGO–PEI–TCPP solution was mounted on a thin film of amorphous carbon deposited on a copper grid for TEM imaging.
2.3 Cell lines and cell culture
Mouse liver cancer CBRH7919 cells (cancer cells) were maintained in Dulbecco's Modified Eagle's Medium (DMEM, Fanbo) supplemented with 10% (v/v) fetal bovine serum (FBS, Fanbo), penicillin–streptomycin (1.0% penicillin–streptomycin, 1.0% Glutamax, respectively, Fanbo) in an incubator supplied with 85% humidity and in a 5% CO2 atmosphere at 37 °C. The cell culture medium was changed every two days and the cells were split upon 80% confluence.
2.4 Cancer cell uptake
For cellular uptake, the mouse liver cancer CBRH7919 cells (200 μL, ≈1 million mL−1) were incubated with rGO–PEI–TCPP (50 μL) in PBS for 1 h at 37 °C. The rGO–PEI–TCPP concentration in the solution during incubation was approximately 1 mg mL−1. The cells were washed three times with PBS to remove unbound rGO–PEI–TCPP before confocal fluorescence imaging. The internalized rGO–PEI–TCPP was visually observed by confocal laser scanning microscopy (Leica TCS SPE) on a cover glass.
2.5 Cytotoxicity
Cck8 assay. For cck8 assays, cancer cells were seeded into 96-well plates at a density of 1 × 104 per well in a medium (200 μL). The cells were then incubated with various concentrations of rGO–PEI–TCPP for 48 h. Furthermore, 20 μL cck8 was added to each well, and then were incubated for 1 h. The optical densities were measured at 450 nm using a multi-detection microplate reader (SynergyTM HT, BioTek Instruments Inc, USA). Data from cck8 assays were analyzed using Origin Pro8.SR3 software and SPSS Statistics V17 software; the significance level was set at P < 0.05.
FCM assays. For FCM assays using 12-well culture plates, exponentially growing cancer cells were seeded (1 × 105 cell per well) and pre-incubated for 24 h, followed by co-incubation with different concentrations of rGO–PEI–TCPP for 48 h. Then, the medium was discarded and the cells were washed three times with PBS. Cells were then detached by trypsinization, centrifuged, and dispersed again in PBS for measurements. Liver cells treated with rGO–PEI–TCPP were stained with propidium iodide (PI) and Annexin V-EGFP before flow cytometry (Coulter Epics XL, America) analysis.
2.6 PTT and PDT
After 1 h of incubation at 37 °C with rGO–PEI–TCPP, the cell culture bottle containing the cancer cells was stored in a carbon dioxide cell incubator until irradiation. The source of irradiation was a 20 W 410 nm diode laser (RPMC lasers). Each cancer cell sample was irradiated with the 410 nm laser at a power of 20 W after incubation, including control cells without any exposure to rGO–PEI–TCPP. Immediately after heating, the cells were seeded in a 96-well plate with 100 μL of media. After 24 h of incubation at 37 °C, cell viability and cell-cycle assays were determined by FCM assays.
Statistical analysis. Statistical analysis of the data was carried out using a student's t-test, and the analysis was performed using SPSS Statistics V17. Differences were considered statistically significant when the P value was less than 0.05. The data are presented as the mean-standard deviation.
3. Result and discussion
3.1 Synthesis of rGO–C6H4–COOH
The Tyndall effect proves the colloidal nature of the suspension, and the very obvious Tyndall effects for the rGO and rGO–C6H4–COOH solutions was demonstrated, as shown in Fig. 1a and b, suggesting that these solutions have excellent dispersability in aqueous solutions. TEM observations were performed to demonstrate the morphologies of GO, rGO and rGO–C6H4–COOH. One can observe that the GO (Fig. 1c) has a big continuous sheet, and rGO sheets have ripple lines between the layers, which were demonstrated in Fig. 1d, suggesting that high quality rGO sheets were prepared. As shown in Fig. 1e, the rGO–C6H4–COOH sheets are very seriously accumulated between the graphene layers, and when rGO–C6H4–COOH is in a solid state, a fold on the edge can also be found. The conjugation of the p-disubstituted phenyl group (–C6H4–COOH) with planar rGO was confirmed by AFM observations and FT-IR measurements. The height of the rGO–C6H4–COOH sheets was confirmed by the AFM observations, as shown in Fig. 1f. The height lies mostly in the range from 1.6 to 3.2 nm. The theoretical height of the single-layer rGO–C6H4–R (R = COOH, NO2, OCH3, or Br) sheet on both sides is about 2.2 nm, and the height of the bare rGO sheets is about 1.0 nm with substituted aromatic groups contributing about 0.6 nm of the height.27 Heights ranging from 1.6 nm to 3.2 nm suggest that single-layers and double-layers of rGO–C6H4–COOH co-exist, and the –C6H4–COOH groups were attached and stand perpendicularly to one side, as well as on both sides of the rGO sheets. The p-disubstituted phenyl groups (–C6H4–COOH) of rGO–C6H4–COOH were confirmed by FT-IR measurements, as shown in Fig. 1g. The peak at 1014.57 cm−1 is the characteristic vibration of the p-disubstituted phenyl group, and the peak at 824.25 cm−1 is the C–H bending vibration of the p-disubstituted phenyl group.28,29
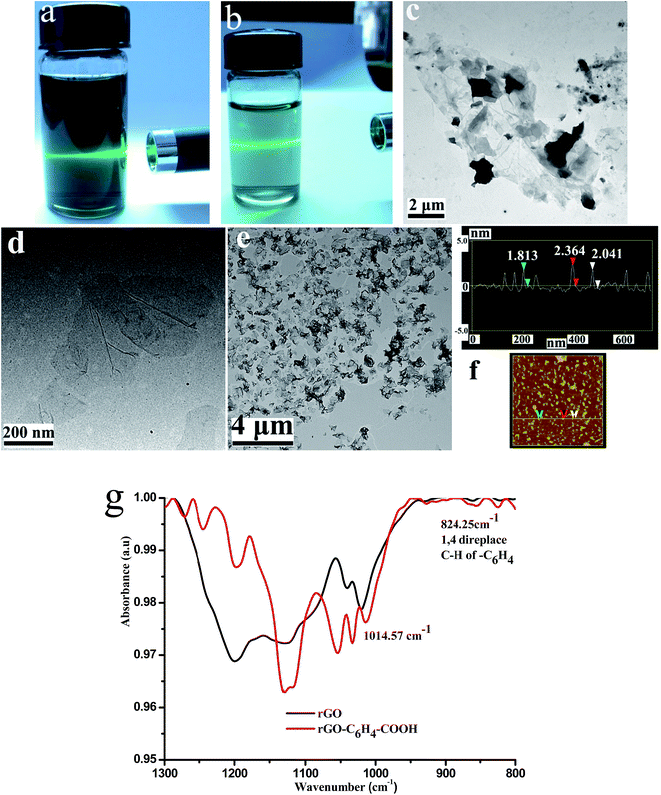 |
| Fig. 1 Characterization of GO, rGO and rGO–C6H4–COOH. (a) Tyndall effect of the rGO colloidal solution; (b) Tyndall effect of the rGO–C6H4–COOH colloidal solution; (c) TEM image of GO; (d) TEM image of rGO; (e) TEM image of rGO–C6H4–COOH; (f) AFM image of rGO–C6H4–COOH; and (g) FT-IR spectra of rGO and rGO–C6H4–COOH. | |
3.2 Synthesis of rGO–PEI–TCPP
According to the comparison of the FT-IR spectra of rGO–C6H4–CO–NH–PEI with rGO–C6H4–COOH (Fig. 2a), a characteristic amide–carbonyl (C
O vibration of NH–CO group) stretching vibration at approximately 1649.93 cm−1 (ref. 30) is present and is consistent with the presence of branched PEI molecules on rGO–C6H4–COOH sheets. The appearance of these characteristic peaks reveals the successful conjugation of the –COOH (rGO–C6H4–COOH) groups with the –NH2 (PEI) groups. Fig. 2b is the colloidal solution of rGO–PEI–TCPP, and the TEM image in Fig. 2c (sheets of rGO–PEI–TCPP were marked by white arrows) shows that the size of rGO–PEI–TCPP was about 20–50 nm. Because of the difficulty in confirming the conjugation of rGO–C6H4–CO–NH–PEI with TCPP by means of FT-IR spectra, UV-Vis spectroscopy was used to analyze the conjugation. By comparing the UV-Vis spectra (Fig. 2d) of TCPP in ethanol with rGO–PEI–TCPP in water, one can observe that the peak overlap of the two compounds, which indicates that TCPP was conjugated to rGO–C6H4–CO–NH–PEI,31 and the fluorescence spectra also supports this point (Fig. 2e). The rGO–PEI–TCPP colloidal solution was very stable and could exist in various types of biological solutions without aggregation (Fig. S1†).
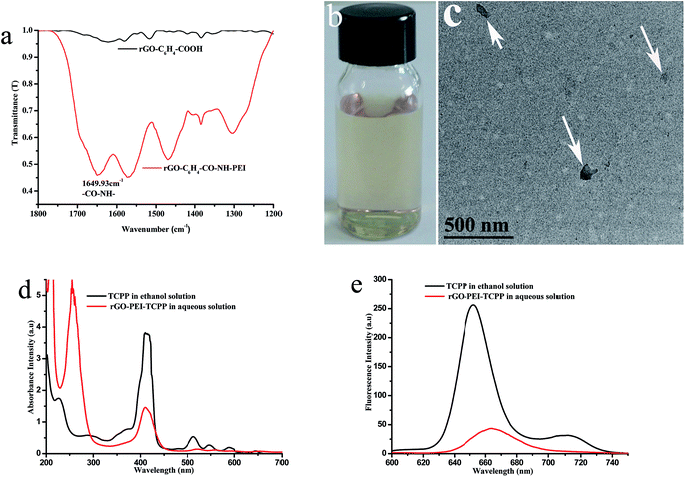 |
| Fig. 2 Characterization of rGO–C6H4–CO–NH–PEI and rGO–PEI–TCPP. (a) FT-IR spectra of rGO–C6H4–COOH and rGO–C6H4–CO–NH–PEI; (b) the rGO–PEI–TCPP colloidal solution; (c) TEM image of rGO–PEI–TCPP; (d) UV-Visible spectra of TCPP and rGO–PEI–TCPP; and (e) fluorescence spectra of TCPP and rGO–PEI–TCPP. | |
3.3 Cytotoxicity of rGO–PEI–TCPP
The uptake behavior of rGO–PEI–TCPP by cancer cells was demonstrated with confocal laser fluorescence microscopy imaging (Fig. 3a). The cytotoxicity of rGO–PEI–TCPP was determined using FCM (flow cytometer) assays (Fig. 3b and S2†) and cck8 assays (Table 1) with CBRH7919 cells. In the cck8 assay (Table 1), the rGO–PEI–TCPP has no clear toxicity to CBRH7919 cells at concentrations up to 15 mg mL−1, meaning that the relative cancer cell viability is not less than 80%. The result of the FCM assay is very similar with that of the cck8 assay, as shown in Fig. 3b. According to the results of the cck8 FCM assays, the rGO–PEI–TCPP concentration of 10 mg mL−1 was used for PTT and PDT in the current experiment. Under irradiation, the temperature of the cell pellets increased in the first minute of irradiation and then leveled off at ∼44 °C for CBRH7919 cancer cells treated with rGO–PEI–TCPP (Fig. 3c). After re-plating the cells in a 96-well plate (n = 6), along with the cells not exposed to 410 nm irradiation, we monitored cell viability after 24 h. CBRH7919 cancer cells were incubated with rGO–PEI–TCPP and irradiated with 410 nm visible light for 5 min before FCM apoptosis assay and cell cycle assay after 24 h.
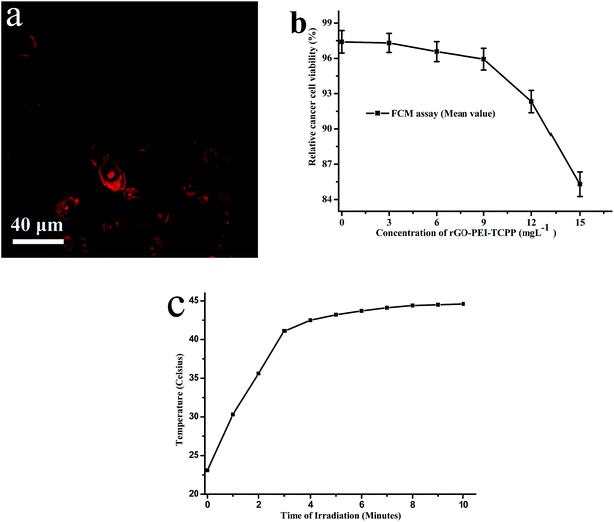 |
| Fig. 3 (a) Confocal laser fluorescence images of rGO–PEI–TCPP uptake by CBRH7919 cancer cells, λex = 532 nm, darkness field; (b) the effect of rGO–PEI–TCPP concentration to relative CBRH7919 cancer cell viability; and (c) temperature change curves of the CBRH7919 cancer cell solution treated with rGO–PEI–TCPP with increasing irradiation times. | |
Table 1 Cck8 assay: effect of different concentrations of rGO–PEI–TCPP for cancer cell viability (% ± s, △ represents that P < 0.05)
Group |
Concentration/mg L−1 |
24 h (%) |
48 h (%) |
72 h (%) |
Control |
0 |
102.9 ± 3.2 |
94.1 ± 5.5 |
93.3 ± 4.3 |
rGO–PEI–TCPP |
3 |
97.5 ± 1.8 |
93.8 ± 3.1△ |
91.6 ± 2.6△ |
rGO–PEI–TCPP |
6 |
97.3 ± 1.8 |
91.9 ± 3.2△ |
91.4 ± 3.1 |
rGO–PEI–TCPP |
9 |
93.1 ± 0.9△ |
91.6 ± 1.5△ |
89.8 ± 1.1△ |
rGO–PEI–TCPP |
12 |
91.6 ± 2.0△ |
88.8 ± 3.5△ |
85.2 ± 2.8△ |
rGO–PEI–TCPP |
15 |
89.8 ± 1.5△ |
88.1 ± 1.9 |
84.9 ± 2.5△ |
3.4 Apoptosis and cell cycle life
The effect of rGO–PEI–TCPP as a PTT and PDT reagent to induce CBRH7919 cancer cell apoptosis can be analyzed with FCM (Fig. 4a–c and S3–S5†). The cancer cell viability of the control group is about 98.06% (Fig. 4a), and the 10 mg L−1 rGO–PEI–TCPP group without irradiation is about 95.46% (Fig. 4b), which indicates the low toxicity of rGO–PEI–TCPP. However, cancer cell viability of untreated cancer cells by rGO–PEI–TCPP (10 mg L−1) with laser irradiation is about 92.59% (Fig. S3b†). Comparing with the control group (Fig. S3a†), this shows that laser irradiation can induce slight cancer cell apoptosis. With the same concentration of rGO–PEI–TCPP (10 mg L−1) with irradiation, the viability of cancer cells decreased sharply. It is about 78.12%, i.e., rGO–PEI–TCPP as a reagent for PTT and PDT could effectively induce cancer cell apoptosis. This could be further proved by confocal laser fluorescence microscopy imaging (Fig. S4†) and comparing with the control group (Fig. S4a and b†). The morphology of cancer cells in the 10 mg L−1 rGO–PEI–TCPP group without irradiation had almost no change (Fig. S4c and d†), which indicates low cytotoxicity. However, the 10 mg L−1 rGO–PEI–TCPP group with irradiation could cause deformation of the cancer cell morphology (Fig. S4e and f†), and the cancer cell shape was apparently contractible, most of which became rounded and condensed. At the same time, spherical protrusions at the cell surface and apoptotic bodies were observed, which is a classic characteristic of apoptosis. Moreover, as another control experiment (Fig. S5†), rGO–C6H4–CO–NH–PEI could also induce cancer cell apoptosis on irradiation, and the cell viability is about 87.75% (Fig. S5c†). Comparing the control group (Fig. S5a†) and the without irradiation group (Fig. S5b†), the apoptosis effect of rGO–C6H4–CO–NH–PEI was less than that of rGO–PEI–TCPP (Fig. 4c). The reason mainly included two aspects, one is the existence of the graphene group. When the laser irradiated the plane of graphene, heat would be produced, and the temperature of the solution would increase, which could induced cancer cell apoptosis.6,8 The other aspect is the existence of the porphyrin group, according to references, singlet oxygen would be produced when the laser irradiates the mixture solution. Singlet oxygen is a very effective germicide, and it could effectively kill the cancer cell.24,25
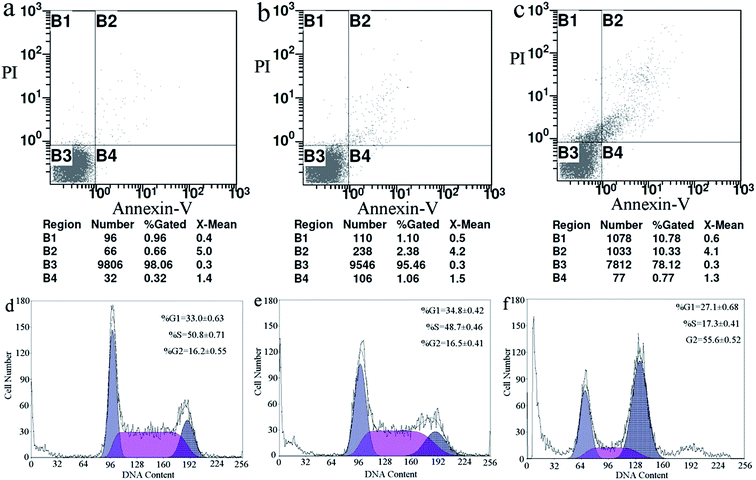 |
| Fig. 4 Effect of rGO–PEI–TCPP on apoptosis and the cell cycle of the CBRH-7919 cell lines. (a) Control apoptosis group; (b) 10 mg L−1 rGO–PEI–TCPP apoptosis group without irradiation; (c) 10 mg L−1 rGO–PEI–TCPP apoptosis group with irradiation; (d) control cell cycle group; (e) 10 mg L−1 rGO–PEI–TCPP cell cycle group without irradiation; (f) 10 mg L−1 rGO–PEI–TCPP cell cycle group with irradiation. In the FCM (a), (b) and (c), the B1, B2, B3 and B4 zones denote the cell necrosis period, the late apoptotic cells period, the normal cell death period and the early apoptotic cells period, respectively. | |
In a cell population, the effect of rGO–PEI–TCPP as a PTT and PDT reagent on each cell varies as the cell advances through its cell cycle (Fig. 4d–f). CBRH7919 cancer cells were employed for the cell cycle assay. As was shown in Fig. 4d, the CBRH7919 cancer cells of the control group in the cell cycle assay tend to block in the S phase, i.e., 50.8% ± 0.71%, 16.2% ± 0.55% in G2 (G2/M) phase, and 33.0% ± 0.63% in G1 phase (G0/G1). Compared with the control group, the 10 mg L−1 rGO–PEI–TCPP group (Fig. 4e), which was not irradiated by laser light, can induce the CBRH7919 cancer cell block mostly in the S phase (48.7% ± 0.46%) and G1 phase (34.8% ± 0.42%), and the arrested rate of the G1 phase is about 16.5% ± 0.41%, which is similar than of the control group. The 10 mg L−1 rGO–PEI–TCPP could not induce the CBRH7919 cancer cells apoptosis. As was shown in Fig. 4f for the rGO–PEI–TCPP group that was irradiated by laser light, the arresting rate of the G1 phase is 27.1% ± 0.68% and the S phase is 17.3% ± 0.41%, suggesting that DNA synthesis in CBRH7919 cells is active after being treated with rGO–PEI–TCPP irradiated by laser light, and the restriction point requirements have been met.32 However, the G2 phase shows a great difference with the control groups and the 10 mg L−1 rGO–PEI–TCPP group that was not irradiated, and the arresting rate is 55.6% ± 0.52%, suggesting that RNA synthesis and the chromatin spiral of CBRH7919 cancer cells treated with rGO–PEI–TCPP that were irradiated are active. After 24 h, the concentration of nanomaterials in the cells could be ranked according to the different phases: G2/M > S > G0/G1, and the cancerous cells can pass through S or G2/M phases more often than healthy cells. These cells are the most sensitive in the late M and G2 phases and most resistant in the late S phase. The pattern of resistance and sensitivity correlates with the level of sulfhydryl compounds in the cells. Sulfhydryls are natural radioprotectors and tend to be at their highest levels in S and at their lowest near mitosis.33 As shown by the rGO–PEI–TCPP group that was irradiated in our experiments, it could effectively hinder the CBRH7919 cancer cells in the G2 phase and prevent the CBRH7919 cancer cells from entering the mitosis period (M phase).
4. Conclusions
Herein, rGO–PEI–TCPP, a PTT and PDT reagent based on rGO–C6H4–COOH (graphene diazotized), was synthesized. rGO–PEI–TCPP had excellent stability in various biological solutions and low cytotoxicity. The rGO–PEI–TCPP could effectively induce CBRH7919 cancer cell apoptosis under laser irradiation through the PTT and PDT function, at the same time, the CBRH7919 cancer cells could be pushed to the vulnerable G2 phase of the cell cycle, which is the most sensitive and susceptible to damage by irradiation.
5. Acknowledgements
This study was financially supported by NSFC (Grant No. 21201020), the Shandong Provincial Independent Innovation and Achievements Transformation Foundation (Grant No. 2014CGZH1316), the Shandong Provincial Natural Science Foundation., China (Grant No. ZR2013BM022) and a Project of Shandong Province Higher Educational Science and Technology Program (Grant No. J15LC01).
References
- L. Jiao, L. Zhang and X. Wang, et al., Nature, 2009, 458, 877–880 CrossRef CAS PubMed.
- X. Li, X. Wang and L. Zhang, et al., Science, 2008, 319, 1229–1232 CrossRef CAS PubMed.
- X. Li, G. Zhang and X. Bai, et al., Nat. Nanotechnol., 2008, 3, 538–542 CrossRef CAS PubMed.
- K. S. Kim, Y. Zhao and H. Jang, et al., Nature, 2009, 457, 706–710 CrossRef CAS PubMed.
- S. D. D. A. Stankovich, G. H. B. Dommett and K. M. Z. E. J. Kohlhaas, et al., Nature, 2006, 282–286 CrossRef CAS PubMed.
- K. Yang, S. Zhang and G. X. Zhang, et al., Nano Lett., 2010, 10, 3318–3323 CrossRef CAS PubMed.
- J. F. Lovell, C. S. Jin and E. Huynh, et al., Nat. Mater., 2011, 10, 324–332 CrossRef CAS PubMed.
- J. T. Robinson, S. M. Tabakman and Y. Y. Liang, et al., J. Am. Chem. Soc., 2011, 133, 6825–6831 CrossRef CAS PubMed.
- J. F. Lovell, C. S. Jin and E. Huynh, et al., Angew. Chem., Int. Ed., 2012, 51, 2429–2433 CrossRef CAS PubMed.
- E. Huynh, J. F. Lovell and B. L. Helfield, et al., J. Am. Chem. Soc., 2012, 134, 16464–16467 CrossRef PubMed.
- T. Numata, T. Murakami and F. Kawashima, et al., J. Am. Chem. Soc., 2012, 134, 6092–6095 CrossRef CAS PubMed.
- J. L. Li, H. C. Bao and X. L. Hou, et al., Angew. Chem., Int. Ed., 2012, 51, 1830–1834 CrossRef CAS PubMed.
- A. Harada, M. Ono and E. Yuba, et al., Biomater. Sci., 2013, 1, 65–73 RSC.
- R. Bonnett, et al., Chem. Soc. Rev., 1995, 24, 19–33 RSC.
- E. D. Sternberg, D. Dolphin and C. Brücker, Tetrahedron, 1998, 54, 4154–4202 CrossRef.
- J. Schmitt, V. Heitz and A. Sour, et al., Angew. Chem., Int. Ed., 2015, 54, 169–173 CrossRef CAS PubMed.
- M. Nurunnabi, K. Parvez and M. Nafiujjaman, et al., RSC Adv., 2015, 5, 42141–42161 RSC.
- H. D. Chen, F. Y. Liu and Z. Lei, et al., RSC Adv., 2015, 5, 84980–84987 RSC.
- Z. Hu, J. Li and Y. D. Huang, et al., RSC Adv., 2015, 5, 654–664 RSC.
- M. J. Hajipour, O. Akhavan and A. Meidanchi, et al., RSC Adv., 2014, 4, 62557–62565 RSC.
- J. M. Shen, F. Y. Gao and L. P. Guan, et al., RSC Adv., 2014, 4, 18473–18484 RSC.
- Y. P. Zeng, Z. Y. Yang and S. L. Luo, et al., RSC Adv., 2015, 5, 57725–57734 RSC.
- P. Laaksonen, A. Walther and J. M. Malho, et al., Angew. Chem., Int. Ed., 2011, 123, 8847–8850 CrossRef.
- T. Murakami, H. Nakatsuji and M. Inada, et al., J. Am. Chem. Soc., 2012, 134, 17862–17865 CrossRef CAS PubMed.
- A. Harada, M. Ono and E. Yuba, et al., Biomater. Sci., 2013, 1, 65–73 RSC.
- G. C. Wei, M. M. Yan and R. H. Dong, et al., Chem.–Eur. J., 2012, 18, 14708–14716 CrossRef CAS PubMed.
- J. R. Lomeda, C. D. Doyle and D. V. Kosynkin, et al., J. Am. Chem. Soc., 2008, 130, 16201–16206 CrossRef CAS PubMed.
- L. Zhang, J. Xia and Q. Zhao, et al., Small, 2010, 6, 537–544 CrossRef CAS PubMed.
- Y. Si and E. T. Samulski, Nano Lett., 2008, 8, 1679–1682 CrossRef CAS PubMed.
- X. M. Sun, Z. Liu and K. Welsher, et al., Nano Res., 2008, 1, 203–212 CrossRef CAS PubMed.
- L. M. Zhang, J. G. Xia and Q. H. Zhao, et al., Small, 2010, 6, 537–544 CrossRef CAS PubMed.
- E. Valk, R. Venta and A. Iofik, et al., Nature, 2011, 480, 128–132 CrossRef PubMed.
- M. Mahmoudi, K. Azadmanesh and M. A. Shokrgozar, et al., Chem. Rev., 2011, 111, 3407–3432 CrossRef CAS PubMed.
Footnote |
† Electronic supplementary information (ESI) available. See DOI: 10.1039/c5ra23986a |
|
This journal is © The Royal Society of Chemistry 2016 |