DOI:
10.1039/C5RA23874A
(Paper)
RSC Adv., 2016,
6, 19700-19706
Exploration of interactions between decyl-β-D-glucopyranoside and bovine serum albumin in aqueous solution
Received
12th November 2015
, Accepted 4th February 2016
First published on 4th February 2016
Abstract
The interactions between decyl-β-D-glucopyranoside (DG) and bovine serum albumin (BSA), in aqueous media, were investigated through the use of surface tension, steady-state fluorescence, and UV-vis absorption spectroscopy measurements. With regard to surface tension, experimental results revealed that the critical micelle concentrations (CMC) within the DG solution, in the absence and presence of BSA, were evaluated as 2.0 mM and 2.34 mM, respectively. Furthermore, the average number of bound DG monomers per BSA molecule was 67 at the critical micelle concentration. Fluorescence and UV-vis absorption spectroscopy indicated that DG had the capacity to quench the intrinsic fluorescence via the formation of DG/BSA complexes. Iodine ion quenching studies have suggested that DG molecules may act to displace tryptophan residues (Trp 214) from their hydrophobic cavities to the surfaces of the protein molecules.
1. Introduction
Globular proteins are typically employed as functional ingredients for health care and pharmaceutical products.1 Bovine serum albumin (BSA) is an important globular protein that has frequently been used as a model for the study of the interactions between proteins and various substrates.2 Surfactants may alter the surface properties of solutions by adsorbing on their surfaces, with the subsequent formation of micelles through aggregation. The interactions between proteins and surfactants have been exploited in many applications, such as reverse micelle extraction, foam separation, protein refolding, and enzyme catalysis.3–7 Therefore, investigations into the interactions between proteins and surfactants are critical and have undergone close scrutiny.8–11
With regards to their ability for interacting with proteins, ionic surfactants are more effective than nonionic ones. It is well known that the charged group of anionic surfactants interacts electrostatically with the oppositely charged group of the amino acids causing denaturation of the protein. Therefore, much experimental work has been made for studying the interaction between proteins and ionic surfactants, being that the studies involving nonionic surfactants are rather scarce.7,12 However, it should be noted that the ionic surfactants can produce significant conformational alterations in the protein structure with subsequent changes in its functionality. Because these modifications are undesirable in many applications, the use of nonionic surfactants is preferred. In addition, due to the favorable physicochemical properties of nonionic surfactants, such as good solubility in both water and organic solvents, excellent stability in solutions, and insensitivity to strong electrolytes, the applications of nonionic surfactants have become extremely wide, particularly in the areas of cosmetics and pharmaceutical preparations.12 Hence, there has been a growing interest toward understanding how these materials interact with biomacromolecules in biological systems.
Presently, a wide range of techniques are utilized for the characterization of binding processes between ethoxylated nonionic surfactants and proteins.13,14 From these investigations, it can be concluded that the hydrophobic moiety of surfactants can bind to the apolar amino acids, whereas the hydrophilic ethyleneoxide chain can interact with the peptide bond and with one or more polar amino acids residues, probably by electrostatic interactions and hydrogen bonding. Recently, a new class of completely biodegradable alkyl polyglycoside nonionic “green” surfactant has been derived from natural products. Decyl-β-D-glucopyranoside (DG) (see Scheme 1) is an important member of this family, which is considered to have a low dermatological impact, biodegradable and manufactured from renewable raw materials.15 Nevertheless, as far as we known, the nature interaction between alkyl polyglycoside nonionic surfactants with proteins appears not to have been studied. On the basis of these facts, we decided to examine this system. In this report, the interaction between nonionic surfactant DG and BSA has been investigated by using surface tension measurements, steady-state fluorescence, and UV-vis absorption spectroscopy. We have paid our more attentions to two aspects: the absorption behavior of protein with surfactant DG and the binding mode of the protein/nonionic surfactant complexes.
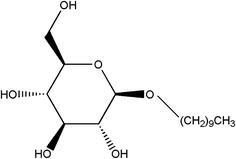 |
| Scheme 1 Molecular structure of decyl-β-D-glucopyranoside. | |
2. Experimental section
2.1. Materials
Bovine serum albumin (BSA) and pyrene were obtained from Sigma and employed as received. Decyl-β-D-glucopyranoside was purchased from Amresco and used without further purification. All DG and DG/BSA solutions were prepared in a Tris–HCl (0.5 M) buffer of constant ionic strength (0.1 M NaCl) at pH 7.4. The pyrene stock solution was prepared in absolute ethanol and stored at 4 °C, whereas the work solutions with lower concentrations were prepared by diluting with the buffer. The water used in these studies was deionized and redistilled, and the other chemicals employed were of analytical grade quality.
2.2. Surface tension measurements
Surface tension measurements were made using the Wilhelmy method with a DCA300 tensiometer (Thermo Cahn), and a 24 mm × 24 mm glass plate, and the solutions were thermostated at 25 °C for 30 min. The glass plate was cleaned with distilled water and acetone, and then flamed. The average measured values of the equilibrium surface tension were obtained in triplicate, and were accurate within ±0.1 mN m−1.
2.3. Fluorescence spectra measurements
Fluorescence measurements were made using a Cary Eclipse fluorescence spectrophotometer (Varian, America), which was equipped with a 150 W Xenon flash lamp. The fluorescence emission spectra were recorded over the range of 300–500 nm following excitation at 280 nm, and both the excitation and emission bandwidths were adjusted to 5 nm.
The synchronous fluorescence spectra were acquired by simultaneously scanning the excitation and emission via a monochromator, where the wavelength intervals (Δλ) were individually fixed at 15 or 60 nm. The excitation and emission bandwidths were set at 5 nm.
The pyrene monomer fluorescence spectrum of each sample was recorded as the average of two emission scans. BSA (2 μM) was equilibrated with DG for one hour prior to the addition of pyrene (1 μM). The solutions were then excited at 335 nm16,17 and the emission spectra between 350 nm and 500 nm were recorded. The excitation and emission slits were set at 5 nm and 2.5 nm, respectively.
2.4. UV-vis absorption spectra measurements
The UV-vis absorption spectrum was recorded with a TU-1810 spectrophotometer (Puxi Analytic Instrument Ltd., Beijing, China) that was equipped with 1 cm quartz cells. The UV-vis absorption spectra of DG and DG–BSA complex were recorded in the range of 200–250 nm. The experiments were carried out at 25 °C and the instrument was thermally controlled by a SHP DC-0515 thermostatic bath.
3. Results and discussion
3.1. The isotherm adsorption behavior of DG to BSA
Surface tension measurements are a useful method to study the surface properties of surfactant solutions. Since proteins may influence surfactant activities, surface tension isotherms of surfactant solutions in the absence and presence of protein molecules may provide insights into the interactions between proteins and surfactants.
The measured surface tension isotherms of DG solutions, in the absence and presence of BSA, are shown in Fig. 1. Moreover, with the studies on the surface tension of DG/BSA systems, we found the interesting isotherm adsorption behavior of DG to BSA, as illustrated in Fig. 2. We can see that the surface tension of DG solutions without BSA progressively decreases with increasing DG concentrations, until it attains 1.0 mM. When the concentration of DG exceeds 1.0 mM, the isotherm shows a sharp decline, whereafter the surface tension progressively decreases with increasing DG concentrations until it attains 2.0 mM, which is the critical micelle concentration (CMC) of DG in solution without BSA. The surface tension corresponding to the CMC is 28.55 dyn cm−1. The steeper drop in the isotherm might have been initiated by changing DG aggregate formations. Additionally, it is shown that at low surfactant concentrations, the surface tension value is nearly equivalent to the presence of the protein, indicating that this species is little surface active. Moreover, it is observed that each plot exhibits a sharp break at each CMC, the surface tension remaining almost constant above this point.
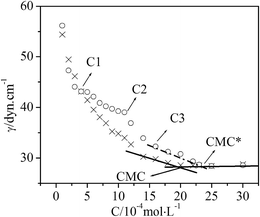 |
| Fig. 1 Gibbs adsorption isotherm of DG/BSA systems in the absence and presence of BSA. C1, C2 and C3 represent the different concentrations regions of DG, pH = 7.40, T = 298 K (×, the solutions without BSA; ○, the solutions with BSA, [BSA] = 5.0 × 10−6 mol L−1). | |
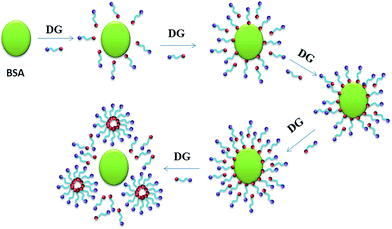 |
| Fig. 2 Illustration of the binding behavior of DG to BSA with the increase concentration of DG in aqueous solution. | |
From Fig. 1 we can also see that the surface tension isotherm of the DG solution with 5.0 × 10−6 mM BSA may be divided into five stages. The first stage possesses a low DG concentration, where the surface tension isotherm is coincident with that of solution without BSA. This indicates that there are no obvious surfactant molecules binding to BSA, and that BSA molecules exhibit negligible surface activity as compared to DG at the concentrations under the experimental conditions. The second stage involves DG concentrations from C1 to C2. For this stage, the surface tension values of the solution with BSA are greater than that of the solution without BSA, and the departure of the two isotherms progressively increases with the increase in DG concentration. Increasing DG concentrations serves to initiate binding between DG and BSA molecules, which acts to lower the concentration of free DG molecules and decrease the DG molecules that are adsorbed on the surface of the solution. Hence, the surface tension values are larger than that of the solution without BSA. So, it can be concluded that a number of DG molecules absorbs on the surface of BSA (see Fig. 2). The third stage involves DG concentrations from C2 to C3, which reveals a steeper drop. This stage corresponds to a steeper drop in the isotherm of the solution without BSA, which might have originated via altered DG aggregate forms as well. At the same time, the above observations indicate that as the surfactant concentration increases, DG/BSA complexes are formed, and they counteract the surface tension lowering to some extent. The fourth stage encompasses DG concentrations from C3 to CMC* (2.34 mM) which is the critical micelle concentration of the DG solution with 5.0 × 10−6 mM BSA. For this stage, the isotherms of DG solutions with BSA are parallel to the isotherms of DG solutions without BSA, which indicates that the difference in the stoichiometrical concentrations of DG for the two isotherms at the same surface tension value is constant. Since this difference is caused by the binding of DG to BSA, it means that the number of DG molecules bound to BSA has no change for this stage. The fifth stage deals with DG concentrations from CMC* to even higher, where the surface tension isotherm is again coincident with that of the solution without BSA. The surface tension corresponding to the CMC* is identical to the surface tension associated with the CMC of DG in the solution without BSA. The equivalent surface tension means that the surface composition is same. The interesting adsorption behavior may originate from the formation of DG micelle (see Fig. 2). Moreover, it is the formation of micelle that drives DG molecules departing from the surface of BSA. Therefore, when the concentration of DG is higher, it can be concluded that BSA and DG compounds don't adsorb on the surface of the solution, or at least, that their adsorption can be neglected.
A method was developed by Nishikido et al.,18 which involved surface tension measurements to quantify the number of protein-bound nonionic surfactants. This method was successfully applied in C12E6/BSA, C12E6/lysozyme,18 MEGA-10/BSA,12 and N-octyl-β-D-glucopyranoside/BSA systems.19 An assumption in this method was that protein/surfactant complexes were not active at the surface. As mentioned above, the DG and BSA system accords with this assumption such that this method can be utilized to calculate the number of DG binding events on BSA. The expression for the surface tension of a solution that contains surfactants and proteins, as proposed by Nishikido,18 is given as follows:
|
γ = −aRTln[a + Ks(a − Γ0pf)Csf] + γp + aRTln a
| (1) |
where
γ is the surface tension,
a is the maximum adsorption of surfactant,
Ks is Langmuir constant for the adsorption of the surfactant at the air–liquid interface,
Γ0p and
γp refer to the amount of adsorbed protein at the air–liquid interface and the surface tension of solutions that contain the same concentration of proteins without surfactant,
f is the ratio between maximum surfactant adsorption and maximum protein adsorption, and
Csf is the concentration of surfactant monomer or free surfactant concentration.
In the case of a pure surfactant solution, the surface tension is given by
|
γs = −aRTln[1 + KsC0s] + γwater
| (2) |
where
C0s is the concentration of the pure surfactant, and
γwater is the surface tension of pure water. According to the assumption that the concentration of surfactants monomers at the CMC of DG solutions without BSA, and at CMC* with BSA, is identical, and taking into account that
γCMC =
γ*
CMC which can be seen in
Fig. 1, we can obtain:
|
γCMC = −aRTln[1 + KsCMC] + γwater
| (3) |
|
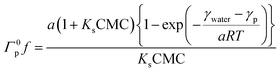 | (4) |
According to the Gibbs adsorption equation, we can acquire a from the slope of the isotherm adsorption in the pre-micellar region. Subsequently, Ks may be determined by introducing a, CMC and γwater into eqn (3). Once Γ0pf from eqn (4) is obtained, Csf may finally be calculated by applying eqn (1). The average number of bound surfactant monomers per protein molecule (υ) may be determined by using the relationship:
|
 | (5) |
where
Cs is the total surfactant concentration. We calculated
υ according to method given in
Table 1, with all of the parameters obtained. From
Table 1, we can see that the average number of bound DG monomers per BSA molecule (
υ) is 67 at CMC*. Since the concentration of BSA is 5.0 × 10
−6 M, the amount of DG bound to the BSA molecules is 0.335 mM, which is practically equivalent to the difference of CMC* (2.34 mM) and CMC (2.00 mM).
Table 1 Parameters obtained from the data treatment based on surface tension measurements for DG and DG/BSA systems at 25 °C
BSA (μM) |
CMC (mM) |
a × 103 (mM m−2) |
Γ0p × f × 103 (mM m−2) |
Ks (L mol−1) |
(Cs)sat (mM) |
(Csf)sat (mM) |
υsat |
0 |
2.00 |
3.56 |
|
|
|
|
|
5 |
2.34 |
5.02 |
3.13 |
7.07 × 104 |
2.34 |
1.99 |
67 |
3.2. The binding sites of DG in BSA
Pyrene monomer fluorescence spectra are highly influenced by the polarity of a solvent.20,21 The ratio (I1/I3) between the intensities of the first (373 nm) and third (384 nm) major vibrational peaks in the fluorescence spectra of pyrene is often used as a probe to measure the polarity of the pyrene environment.12,22,23 Since pyrene has a much lower solubility in water (∼10−7 M) than in hydrocarbon (0.075 M), it is very strongly distributed into micelles as soon as they form. Moreover, the transfer of pyrene from the body of solution to the micelles is accompanied by a decrease in the I1/I3 ratio. Hence, it is expected that the formation of micelles would be revealed by a sudden decrease in the I1/I3 ratio, when a surfactant is added to an aqueous solution that contains pyrene.
Fig. 3 shows the I1/I3 ratio of pyrene fluorescence as a function of DG concentration, in the absence and presence of BSA. In the solution without BSA, the values of I1/I3 are larger at lower DG concentrations, and smaller at higher DG concentrations. The steep change of I1/I3 is in the vicinity of 2.0 mM of DG. This is consistent with the CMC (2.0 mM) obtained in surface tension measurements. In the solution with BSA, the values of I1/I3 are much smaller than that in the solution without BSA at lower DG concentrations. This illustrates that pyrene is bound to the hydrophobic sites of BSA. As DG concentrations are increased, the I1/I3 value is also gradually decreased. This indicates that the binding of DG molecules to BSA displaces the pyrene from its BSA resident hydrophobic sites. The steep decrease of I1/I3 is in the region of between 2.0 mM and 2.5 mM DG. This is a reasonable result because the probe-based procedure requires the formation of aggregates where the probe can be incorporated. Simultaneously, this result is also in accordance with the CMC* (2.34 mM) that obtained in the surface tension measurements.
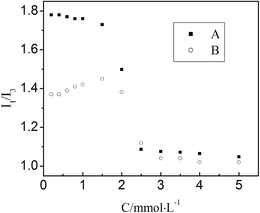 |
| Fig. 3 Dependencies of I1/I3 ratios on DG concentrations in solutions with and without BSA. (pH = 7.40, T = 298 K) , solutions without BSA; ○, solutions with BSA, [BSA] = 2.0 × 10−6 mol L−1. | |
3.3. The fluorescence quenching mechanism of BSA
The fluorescence of proteins originates primarily from Trp residues and tyrosine (Tyr) residues. Synchronous fluorescence may be utilized to analyze changes of the conformation of proteins.24 If Δλ = 15 nm, the synchronous fluorescence spectra exhibit the spectral character of Tyr residues only. However, if Δλ = 60 nm, the synchronous fluorescence spectra exhibit the spectral character of Trp residues only. As shown in Fig. 4, the fluorescence intensity of BSA was increased from 140 to 230 for Δλ = 15 nm (Fig. 4a) and decreased from 880 to 550 for Δλ = 60 nm (Fig. 4b) as the DG concentrations were increased. This indicates that DG interacts, for the most part, with Trp residues rather than Tyr residues.
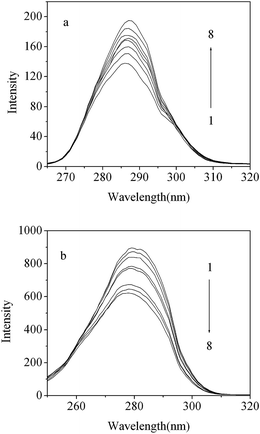 |
| Fig. 4 Synchronous fluorescence spectrum of BSA in the absence and presence of DG (pH = 7.40, T = 298 K): (a) Δλ = 15 nm; (b) Δλ = 60 nm. [BSA] = 2.0 × 10−6 mol L−1; from 1 to 8: [DG] = 0, 1.0, 2.0, 3.0, 4.0, 5.0, 6.0, 8.0 ( ×10−4 mol L−1). | |
Fig. 5 shows the effects of different amounts of DG on the fluorescence emission spectra of BSA. It was observed that the fluorescence intensity of BSA was decreased as the DG concentration was increased. This indicates that DG can interact with the Trp residues of the BSA, while quenching their intrinsic fluorescence. Additionally, an apparent blue shift was observed for the emission wavelengths with increasing DG concentrations. This reveals that the microenvironment in close proximity to the BSA Trp residues is converted to a more hydrophobic environment. This may be initiated by the approach of neutral DG molecules to the Trp residues.
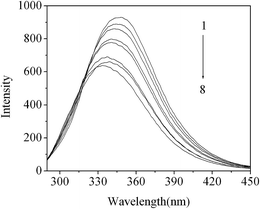 |
| Fig. 5 The fluorescence spectra of the DG/BSA system. From 1 to 8: [DG] = 0, 1.0, 2.0, 3.0, 4.0, 5.0, 6.0, 8.0 ( ×10−4 mol L−1); [BSA] = 2 × 10−6 mol L−1, pH = 7.4; λex = 280 nm. | |
3.4. Conformational changes of BSA induced by DG
The binding of surfactants to proteins leads to changes in the conformation of the proteins. Fig. 6 depicts the UV-vis absorption spectra of BSA in solution, with and without DG. From Fig. 6 we can see that there is a decrease in the UV-vis absorption spectrum of BSA at 213 nm with the addition of DG, which corresponds to the adsorption of protein backbones. It is seen that the absorption of BSA gradually decreases with the addition of DG. Moreover, a slight blue shift is also observed in the binding process. These observations are considered to be the result of a conformational change that is due to the α-helix content.25 This indicates that BSA molecules associate with DG, forming a DG/BSA complex.
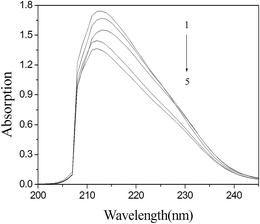 |
| Fig. 6 UV absorption spectra of the interaction between DG and BSA. [BSA] = 2 × 10−6 mol L−1, from 1 to 5: [DG] = 0, 1, 3, 6, 9 ( ×10−4 mol L−1). (T = 298 K, pH = 7.4). | |
Iodine ions are potent fluorescence quenchers of exposed Trp residues; however, their charge and large size restrict them from accessing the Trp that are embedded within the hydrophobic cores of proteins.26 Therefore, iodine ion quenching experiments may be employed to quantify the ratio of Trp on the surface of a protein to its overall Trp, and then to study the binding parameters of the proteins with ligands.27
Since the quenching reaction of I− on Trp is dynamic,21 a modified form of the Stern–Volmer equation may be used as follows:28
|
F0/ΔF = 1/(f0K[Q]) + 1/f0
| (6) |
where
K is the Stern–Volmer quenching constant, Δ
F =
F0 −
F is the difference of the fluorescence intensity of BSA, with and without I
−,
f0 is the fraction of residues that may be quenched by I
−, and [Q] is the concentration of I
−.
Eqn (6) reveals that there is a linear correlation between
F0/Δ
F and 1/[Q]. The data shown in
Fig. 7 have been fitted to
eqn (6), and the values of
f0 obtained were 54.41% for the BSA system, and 59.69% for the DG and BSA system. This shows that the Trp residue fraction on the surface of the BSA is higher in the presence of DG, than in its absence.
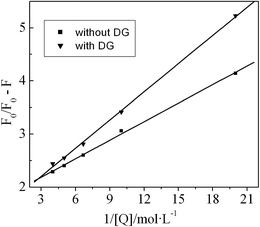 |
| Fig. 7 Effects of I− on the fluorescence spectrum of BSA solution, with and without DG. [BSA] = 2.0 × 10−6 mol L−1, pH = 7.40, T = 298 K ( , the solution without DG. ○, the solution with 1.0 × 10−4 mol L−1 DG). | |
In fact, BSA contains two tryptophan residues (Trp 135 and Trp 214; the numbering scheme in accordance with the BSA mature protein in Unit Protcode: ALBU_BOVIN). The majority of the polar amino acid residues are resident on the surface of the BSA, whereas most of the non-polar amino acid residues are contained within a large hydrophobic cavity.29 Trp 135 is more exposed to a hydrophilic environment, whereas Trp 214 is deeply embedded within a hydrophobic cavity.30 A study of the UV-absorption spectra mentioned above indicated that the interactions between DG and BSA act to alter the morphology of the BSA. Since DG is a neutral molecule, it preferentially interacts with the hydrophobic cavity of BSA. Additionally, it is well-known that Trp fluorescence decreases upon exposure to a polar environment; therefore, it can be postulated that the binding of surfactant on protein causes some conformational change in the protein structure, resulting in a greater exposure of Trp residues to the aqueous environment. Consequently, this interaction compresses the contours of the hydrophobic cavity such that it effectively purges the Trp 214. This is likely the reason for the higher fraction of Trp residues on the surface of the BSA in the presence of DG, than are present in its absence.
4. Conclusions
This work investigated the interactions between decyl-β-D-glucopyranoside and BSA. Surface tension and pyrene monomer fluorescence spectra studies revealed the isotherm adsorption behavior of DG to BSA. It was indicated that the average number of bound DG monomers per BSA molecule was 67 at CMC* and that the complex was not active at the surface. In the solution devoid of BSA, the CMC of DG was 2.0 mM, whereas the CMC* of DG was 2.34 mM in the solution with 5.0 × 10−6 mM BSA. The UV-absorption spectrum experiment revealed a conformational change of BSA when it was associated with DG, which indicated again that DG/BSA complexes may be formed in the system studied. BSA fluorescence spectra experiments showed that DG interacted primarily with Trp residues, rather than Tyr residues, and further, that these interactions quenched the intrinsic fluorescence of BSA. Iodine ion quenching studies revealed that DG molecules may effectively displace Trp 214 from the hydrophobic cavities of BSA molecules to their surface. Consequently, these results illustrated the binding mode of BSA with DG. Through the current work, the absorption behavior, the binding mode and fluorescence quenching mechanism of DG/BSA systems have been elucidated, so our main objectives have reached on the whole. The interactive behaviors of nonionic surfactants (DG) with BSA studied here provide an effective approach to further study the interfacial properties of nonionic surfactants with proteins. Now, the studies on the interaction between the other members of alkyl polyglycoside with different proteins are carrying out in our laboratory, which will promote the understanding the nature of the binding properties of nonionic surfactants with proteins in the future.
Acknowledgements
This work was supported by the National Natural Science Foundation of China (No. 21303043, 21273061), the Research Fund for the Doctoral Program of Higher Education of China (No. 20114104110002), the Program for Innovative Research Team (in Science and Technology) in University of Henan Province (No. 14IRTSTHN005).
References
- W. Chanasattru, E. A. Decker and D. J. McClements, Impact of cosolvents (polyols) on globular protein functionality: ultrasonic velocity, density, surface tension and solubility study, Food Hydrocolloids, 2008, 22, 1475–1484 CrossRef CAS.
- X. R. Li, G. K. Wang, D. J. Chen and Y. Lu, β-Carotene and astaxanthin with human and bovine serum albumins, Food Chem., 2015, 179, 213–221 CrossRef CAS PubMed.
- M. A. Mir, N. Gull, J. M. Khan, R. H. Khan, A. A. Dar and G. M. Rather, Interaction of bovine serum albumin with cationic single chain + nonionic and cationic gemini + nonionic binary surfactant mixtures, J. Phys. Chem. B, 2010, 114, 3197–3204 CrossRef CAS PubMed.
- B. K. Paul, A. Samanta and N. Guchhait, Exploring hydrophobic subdomain IIA of the protein bovine serum albumin in the native, intermediate, unfolded, and refolded states by a small fluorescence molecular reporter, J. Phys. Chem. B, 2010, 114, 6183–6196 CrossRef CAS PubMed.
- C. M. C. Faustino, A. R. T. Calado and L. Garcia-Rio, Gemini surfactant–protein interactions: effect of pH, temperature, and surfactant stereochemistry, Biomacromolecules, 2009, 10, 2508–2514 CrossRef CAS PubMed.
- N. Gull, S. Chodankar, V. K. Aswal, P. Sen, H. R. Khan and U. D. Kabir, Spectroscopic studies on the interaction of cationic surfactants with bovine serum albumin, Colloids Surf., B, 2009, 69, 122–128 CrossRef CAS PubMed.
- S. Mehan, V. K. Aswal and J. Kohlbrecher, Cationic versus anionic surfactant in tuning the structure and interaction of nanoparticle, protein, and surfactant complexes, Langmuir, 2014, 30, 9941–9950 CrossRef CAS PubMed.
- Z. Liu, Z. H. Liu, D. H. Wang, F. X. Ding and N. Yuan, Smart hydrogels for bioseparation, Bioseparation, 1998, 7, 177–184 CrossRef.
- D. N. Lu, Z. Liu, Z. X. Liu, M. L. Zhang and Y. P. Ou, Molecular simulation of surfactant-assisted protein refolding, J. Chem. Phys., 2005, 122, 134902–134912 CrossRef PubMed.
- J. Wang, D. N. Lu, Y. Lin and Z. Liu, How CTAB assists the refolding of native and recombinant lysozyme, Biochem. Eng. J., 2005, 24, 269–277 CrossRef CAS.
- C. M. L. Carvalho and J. M. S. Cabral, Reverse micelles as reaction media for lipase, Biochimie, 2000, 82, 1063–1085 CrossRef CAS PubMed.
- C. C. Ruiz, J. M. Hierrezuelo, J. Aguiar and J. M. Peula-Garcia, Physicochemical Studies on the Interaction between N-Decanoyl-N-Methylglucamide and Bovine Serum Albumin, Biomacromolecules, 2007, 8, 2497–2503 CrossRef CAS PubMed.
- T. Chakraborty, I. Chakraborty, S. P. Moulik and S. Ghosh, Physicochemical and Conformational Studies on BSA–Surfactant Interaction in Aqueous Medium, Langmuir, 2009, 25, 3062–3074 CrossRef CAS PubMed.
- T. Cserhati, Alkyl ethoxylated and alkylphenol ethoxylated nonionic surfactants: interaction with bioactive compounds and biological effects, Environ. Health Perspect., 1995, 103, 358–364 CrossRef CAS PubMed.
- C. M. Persson, A. J. Kumpulainen and J. C. Eriksson, Adsorption of n-Decyl-β-D-Glucopyranoside and n-Decyl-â-D-Maltopyranoside Mixtures at the Liquid–Vapor Interface, Langmuir, 2003, 19, 6110–6114 CrossRef CAS.
- K. K. Andersen, P. Westh and D. Otzen, Global study of myoglobin–surfactant interactions, Langmuir, 2008, 24, 399–407 CrossRef CAS PubMed.
- X. Y. Wang, J. B. Wang, Y. L. Wang, J. P. Ye and H. K. Yan, Micellization of a series of dissymmetric gemini surfactants in aqueous solution, J. Phys. Chem. B, 2003, 107, 11428–11432 CrossRef CAS.
- N. Nishikido, T. Takahara, H. Kobayashi and M. Tanaka, Interaction between hydrophilic proteins and nonionic detergents studied by surface tension measurements, Bull. Chem. Soc. Jpn., 1982, 55, 3085–3088 CrossRef CAS.
- Y. Zheng, D. J. Chen, G. K. Wang, M. H. Xu and Y. Lu, Studies on the interaction between N-octyl-β-D-glucopyranoside and bovine serum albumin, Acta Chim. Sin., 2011, 60, 633–639 Search PubMed.
- H. Chikako, I. Miwa, T. Rie and E. Kazutoyo, Solubilization of pyrene in CnE7 micelles, Langmuir, 2002, 18, 1999–2003 CrossRef.
- H. Chikako, K. Hiroko, M. Kenichiro and E. Kazutoyo, Studies on bovine serum albumin–sodium dodecyl sulfate complexes using pyrene fluorescence probe and 5-doxylstearic acid spin probe, J. Colloid Interface Sci., 2004, 278, 310–317 CrossRef PubMed.
- T. Costa, K. Schillén, M. D. G. Miguel, B. Lindman and J. S. D. Melo, Association of a hydrophobically modified polyelectrolyte and a block copolymer followed by fluorescence techniques, J. Phys. Chem. B, 2009, 113, 6194–6204 CrossRef CAS PubMed.
- D. Wu, G. Y. Xu, Y. H. Sun, H. X. Zhang, H. Z. Mao and Y. J. Feng, Interaction between proteins and cationic gemini surfactant, Biomacromolecules, 2007, 8, 708–712 CrossRef CAS PubMed.
- J. B. F. Lloyd, Synchronized excitation of fluorescence emission spectra, Nature, Phys. Sci., 1971, 231, 64–65 CrossRef CAS.
- C. X. Sun, J. H. Yang, X. Wu, X. R. Huang, F. Wang and S. F. Liu, Unfolding and refolding of bovine serum albumin induced by cetylpyridinium bromide, Biophys. J., 2005, 88, 3518–3524 CrossRef CAS PubMed.
- S. Y. Wang, X. L. Xu and Q. L. Liu, Application of fluorescence spectroscopy in the study of protein conformation, Adv. Chem., 2001, 13, 257–260 Search PubMed.
- M. Kallubai, A. Rachamallu, D. P. Yeggonia and R. Subramanyam, Comparative binding mechanism of lupeol compounds with plasma proteins and its pharmacological importance, Mol. BioSyst., 2015, 11, 1172–1183 RSC.
- Y. J. Hu, Y. Liu and X. H. Xiao, Investigation of the interaction between berberine and human serum albumin, Biomacromolecules, 2009, 10(3), 517–521 CrossRef CAS PubMed.
- X. R. Li, D. J. Chen, G. K. Wang and Y. Lu, Investigation on the interaction between bovine serum albumin and 2,2-diphenyl-1-picrylhydrazyl, J. Lumin., 2014, 156, 255–261 CrossRef CAS.
- A. Sulkowska, Interaction of drugs with bovine and human serum albumin, J. Mol. Struct., 2002, 614, 227–232 CrossRef CAS.
|
This journal is © The Royal Society of Chemistry 2016 |