DOI:
10.1039/C5RA23795E
(Paper)
RSC Adv., 2016,
6, 7717-7722
Mechanism of the antioxidation effect of α-Fe2O3 on silicone rubbers at high temperature†
Received
11th November 2015
, Accepted 23rd December 2015
First published on 5th January 2016
Abstract
The high temperature resistance of silicone rubber (SR) could be significantly improved by adding hematite (α-Fe2O3). In this study, the variation of α-Fe2O3 and polysiloxanes was investigated by ultraviolet-visible absorption spectroscopy, X-ray diffraction, Mössbauer spectroscopy, and 29Si NMR spectroscopy after SR aging for 12 h in air at 350 °C. The results indicate that Fe3+ is reduced to Fe2+ by organic free radicals (R˙) in the process of aging. Fe3+ captures R˙ to protect the polysiloxanes from being destroyed and is transformed into Fe3O4. Moreover, magnetic Fe3O4 is only found in the inner layer of SR; however, it is not detected in the outer layer. Therefore, a new mechanism of antioxidation is proposed: on the outer part of SR, the reduction–oxidation cycle of Fe3+ is formed because of sufficient oxygen; however, inside the SR, α-Fe2O3 is gradually reduced to Fe3O4.
Introduction
Silicone rubbers (SRs) with their unique physical and chemical properties, which include exceptionally low glass transition temperatures (Tg) and superior high thermal stability, are extensively investigated and used for various commercial applications.1–6 When SR is used as a sealant, it is always exposed to high temperature air, which results in the oxidation and degradation of the side chains of polysiloxane, which lead to a decrease in the mechanical properties and flexibility of SR. Therefore, improvement in the antioxidation of SR at high temperatures is highly desirable in order to prolong its service life.7 In general, two methods are always applied to improve the antioxidation of SR. First, the modification of molecular chains of the polymer, for instance, poly(diphenyl)siloxane is reported to have better resistance to oxidative degradation than poly(dimethyl)siloxane.6–8 However, this modification method is very inconvenient and expensive because it involves the synthesis of monomers containing phenylsiloxanes.9 Another simple and efficient method involves the use of antioxidation additives such as ferric oxide (Fe2O3) and cerium oxide.10–13 Fe2O3 is a common and most effective heat-resistant additive, which significantly improves the antioxidation of SR. Many studies have reported that Fe2O3 could enhance the thermal stability of SR,13–16 nevertheless, there is still no consensus about the mechanism of the antioxidation effect of Fe2O3 on SR. Nielsen13 suggested that free radicals are destroyed as a result of the reduction of the metal ion as follows:
Mx+1 + R˙ → Mx + R+ (where R˙ is ≡ Si˙ or ≡ SiCH2˙) |
Further studies indicate that at a fairly high oxygen concentration, there is the possibility of regeneration of the metal ion, Mx+1, via the following reaction:
However, direct evidence supporting this mechanism has rarely been reported.13 Kuzmunskii et al.16 claimed that the mechanism of antioxidation did not involve the reduction of Fe3+ by free radicals, but rather involved the absorption of Fe2O3, which causes the oxidation of active molecules or adsorption and inactivation of free radicals, thus decelerating the oxidation of SR.
In this study, a more detailed process of the antioxidation effect of Fe2O3 on SR is investigated. A variety of direct evidence obtained by ultraviolet-visible (UV-Vis) absorption spectroscopy, X-ray diffraction (XRD), Mössbauer spectroscopy and 29Si NMR spectroscopy were used to confirm the mechanism of the reduction–oxidation cycle for the metal ion. This study could possibly help to dissolve the controversy, which exists for decades, about the mechanisms of antioxidation of SR by Fe2O3 at high temperatures.
Experimental
Materials and sample preparation
α,ω-Hydroxylpolydimethylsiloxane was purchased from Dow Corning Co., Ltd. Polysilazane crosslinking agent (KH-CL) and α-Fe2O3 (purity: 99.5%) powder were synthesized in our laboratory.17,18 Dibutyltin dilaurate (purity: 97%) was purchased from Beijing InnoChem Science & Technology Co., Ltd. SR was vulcanized by 5% KH-CL with 20% SiO2 and 5% α-Fe2O3 as an additive using 0.1% dibutyltin dilaurate as the catalyst. The samples were vulcanized for 14 days at room temperature and cut down into dumbbell shapes prior to the mechanical properties test.
Measurements
Mechanical properties were measured at room temperature using an Instron 5565. Solid state 29Si NMR experiments were performed at room temperature using a Bruker AVANCE III 400 spectrometer operating at a static magnetic field of 7.05 T using a 4 mm magic angle spinning (MAS) probe. 29Si NMR spectra were obtained under MAS conditions with cross polarization (CP) excitation, using π/2 pulse widths of 4.0 μs. 29Si NMR chemical shifts were determined relative to the external standards of tetramethylsilane (TMS, δ = 0 ppm). The Mössbauer spectrum (MS) was obtained using a MS-500 spectrometer with a 57Co source in a palladium matrix on a constant acceleration drive at room temperature and zero fields. The calibrations of the velocity scale in the magnetic and paramagnetic measurement ranges were performed using α-Fe and sodium nitroprusside, respectively. The isomer shifts for the spectra of each of the ranges were provided with respect to the calibrations used. The spectra were processed using a program that realizes the least-squares method. UV absorption spectroscopy was performed using a UV-2600 spectrometer. XRD was performed on a Rigaku (Japan) D/max 2500 X-ray diffractometer equipped with graphite-monochromatized CuKα radiation (λ = 1.5418 Å).
Results and discussion
The properties of SR before and after aging for 12 h in air at 350 °C are listed in Table 1. SR-1 is SR with α-Fe2O3 as an antioxidation additive and SR-2 is without α-Fe2O3. The mass loss of SR-1 after aging is 11.4%; however, the mass loss of SR-2 can reach up to 24.4%, which is more than twice that of SR-1. Moreover, significant differences in the properties are observed after aging. The tensile strength of SR-1 decreases from 3.33 to 2.27 MPa, thus 68% strength still remains; however, the tensile strength of SR-2 decreases from 3.04 to 0.75 MPa, and only 25% strength remains. The release of free radicals leads to a decrease in the elongation at break of both SR-1 and SR-2 after aging, which results in an increase in the density of cross linking of SR even to the extent of over cross linking. The elongation at break of SR-1 is still 114% after aging; nevertheless, for SR-2 it is only 42%, which indicates the loss of almost complete flexibility. This result is in good agreement with the result corresponding to an increase in hardness. The hardness of SR-2 increases from 40 to 52 because of over cross linking, which is much higher than the increase in hardness of SR-1 (from 40 to 42). Comparisons of the properties variation after aging indicate that α-Fe2O3 could significantly improve the antioxidation of SR. Consequently, more research effort is devoted to investigate the role of α-Fe2O3 to improve the antioxidation of SR.
Table 1 Properties of SR before and after aging for 12 h in air at 350 °C
Sample |
Properties before aging |
Properties after aging |
Tensile strength (MPa) |
Elongation at break (%) |
Hardness (A) |
Tensile strength (MPa) |
Elongation at break (%) |
Hardness (A) |
Mass loss (%) |
SR-1 |
3.33 |
391 |
40 |
2.27 |
114 |
42 |
11.4 |
SR-2 |
3.04 |
388 |
40 |
0.75 |
Fragmentary |
52 |
24.4 |
All the samples were cut longitudinally, as shown in Fig. 1. Fig. 1Ab shows that the longitudinal section of SR-1 is a sandwich structure, revealing a bright red outer part and black inner part. Nevertheless, this sandwich structure did not appear in the SR after aging for 12 h in nitrogen at 350 °C (Fig. 1Ac). This indicates that the generation of the atrament of the sandwich structure was probably related to the oxygen present in air. More interestingly, the atrament with magnetic character can be attracted easily by a ferromagnet (Fig. 1B). Thus, investigation is required to explore the atrament on earth. Moreover, extensive research effort needs to be devoted to investigate the relationship between the atrament and the antioxidation effect of α-Fe2O3.
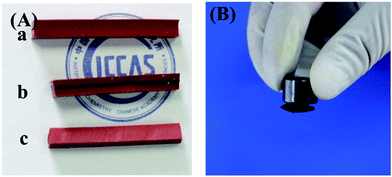 |
| Fig. 1 (A) Images of the SR samples: (a) before aging, (b) after aging in air, and (c) after aging in nitrogen. (B) Magnetic effect of SR after aging in air. | |
To confirm whether the valence of iron changed in the process of aging, UV-Vis absorption spectroscopy was applied to analyze the valence of iron. Fe2+ can be easily coordinated with 1,10-phenanthroline, which results in the formation of a stable product, Fe(phen)32+. Therefore, the coordination compound exhibits a characteristic absorption at 510 nm in the UV-Vis absorption spectrum. Thus, UV-Vis absorption spectroscopy is a simple and convenient tool to verify the existence of Fe2+ in the samples after aging. The atrament of SR-1 was swollen in concentrated hydrochloric acid, and the metallic oxide is dissolved. A small amount of 1,10-phenanthroline was used as a chromogenic agent in the solution. The spectrum shown in Fig. 2 exhibits a strong absorption at 510 nm, which confirms the reduction of Fe3+ to Fe2+ in SR-1 after aging at a high temperature in air.
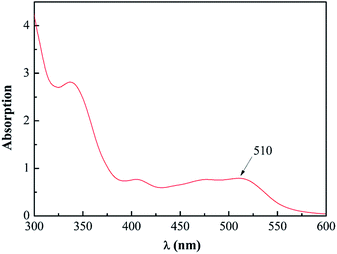 |
| Fig. 2 UV-Vis absorption spectrum of the atrament of SR-1. | |
XRD was also applied to monitor the procedure of aging. Fig. 3 shows that with the extension of aging time, the peaks of α-Fe2O3 disappear gradually, and after aging for 12 h, the peaks correspond to Fe3O4 (JCPDS 85-1436).19,20 All the lattice planes of Fe3O4 are labeled, as shown in Fig. 3d, which indicate that the atrament of the sandwich structure is probably Fe3O4. Thus, this result is also in good agreement with the result that atrament is magnetic, as mentioned before.
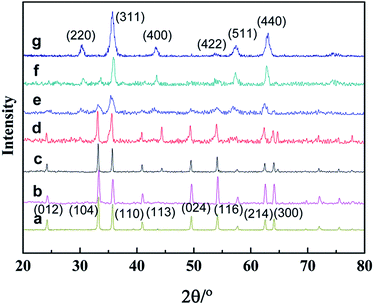 |
| Fig. 3 Monitoring of the procedure of aging by XRD: (a) α-Fe2O3 powder; (b) aging for 12 h, the outer layer; (c) before aging; (d) aging for 4 h; (e) aging for 8 h; (f) aging for 12 h, the atrament; and (g) Fe3O4 powder. | |
Moreover, the lattice plane (012) of α-Fe2O3 first disappears after aging for 8 h (Fig. 3e). This illustrates that the activation energy of Fe3+ on the lattice plane (012), which was reduced by organic free radicals, was the lowest, thus indicating its prior participation in the antioxidation reaction at the initial stage of aging. Subsequently, the Fe3+ on the lattice planes (104), (330), (024), and (113) all disappear in succession. This is attributed to different activities of Fe3+ for antioxidation on different lattice planes. Moreover, the XRD of the outer layer of the sandwich structure exhibits the existence of only α-Fe2O3 (Fig. 3b), which indicates the generation of only Fe3O4 in the inner layer of SR.
Analysis of 57Fe Mössbauer spectra
The Mössbauer spectra (MSs) obtained in the magnetic range are shown in Fig. 4, and their parameters are listed in Table 2. The spectrum of sample I is represented by a superposition of two Zeeman splitting sextets. The sextets with Heff = 518 and 492 kOe are associated with the resonance absorption of Fe3+ ions in α-Fe2O3 and orthoferrite (Fe3+(complex)) structures, respectively, since these parameters (Fig. 4 and Table 2) are close to the published data for iron oxides.19,21–23 The MS lines are broad without visible separate components (Fig. 4); therefore, we tried a mathematical approach to separate the lines of the slightly resolved spectra. This approach allows one to find unresolved lines in experimental spectra using their narrowing and to fit experimental data using a hypothesis model. By applying this approach to the experimental spectra of samples I and II, the different isomer shift (IS) and quadrupole splitting (QS) values were discerned. Fig. 4B demonstrates that using data for the IS and QS of ions with different valences and coordinations, the discerned sextets are attributed to the resonance absorption of remaining α-Fe2O3, Fe13+, and Fe2+ and Fe23+. Based on the published parameters,19–22,24,25 Fe13+ and Fe2+ and Fe23+ were assigned to magnetite (Fe3O4) with an inverse spinel structure, and Fe13+ and Fe2+ and Fe23+ occupied the tetrahedron and octahedron positions, respectively. Furthermore, the ratio of the relative area of the component was 27/67, which indicates that magnetite was not generated in stoichiometry, and the component of magnetite was close to (Fe2O3)1.00(FeO)1.11. These results are in agreement with the XRD analysis, and further confirm that Fe3O4 is generated in the process of antioxidation. Moreover, the Fe3+(complex) is regarded as the composite of Fe3+ on the surface of the α-Fe2O3 complex with oxygen on the backbone of SR13 (Fig. 5).
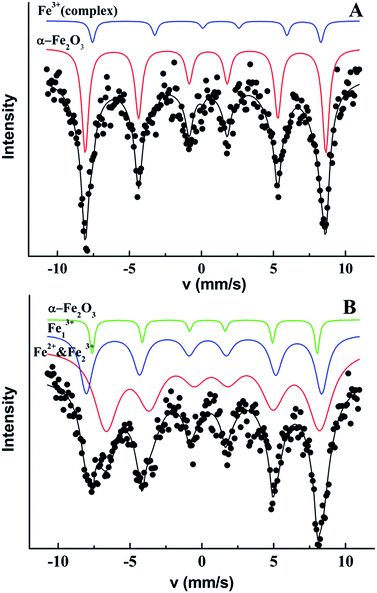 |
| Fig. 4 Mössbauer spectra of sample I (A) before aging and II (B) after aging for 12 h. | |
Table 2 Parametersa of the Mössbauer spectra of samples I and II
Sample |
Ion, phase |
IS, mm s−1 |
QS, mm s−1 |
Heff, kOe |
fwhm, mm s−1 |
S, % |
Heff = effective magnetic field; IS = isomer shift relative to α-Fe, QS = quadrupole splitting; fwhm = absorption line full width at half-maxima; and S = relative area of component. Measurement error of IS, QS, and fwhm: ±0.04 mm s−1, Heff: ±5 kOe, and S: <10%. |
I |
α-Fe2O3 |
0.37 |
0.10 |
518 |
0.29 |
94 |
Fe3+(complex) |
0.86 |
0.49 |
492 |
0.22 |
6 |
II |
α-Fe2O3 |
0.30 |
0.10 |
486 |
0.18 |
6 |
Fe13+ |
0.28 |
0.13 |
508 |
0.52 |
27 |
Fe2+ & Fe23+ |
0.71 |
0.06 |
461 |
1.00 |
67 |
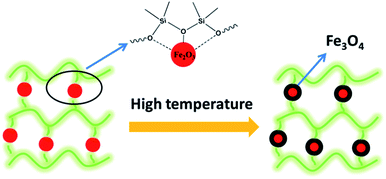 |
| Fig. 5 α-Fe2O3 complex with oxygen on the backbone of SR and antioxidation proceeds from the surface to the inner layer of the α-Fe2O3 particles. | |
This complex structure enhanced the interaction force between the α-Fe2O3 particles and SR, which significantly inhibited the degradation of SR in an inert atmosphere at a high temperature. Thus, α-Fe2O3 could significantly improve the thermal stability of SR. Fig. 6 describes that the abovementioned inference is confirmed by the fact that the smaller size α-Fe2O3 with a higher specific surface area could tremendously enhance the temperature of 5% weight loss from 380 to 550 °C compared to common α-Fe2O3. Furthermore, the value of the IS was significantly affected by the size of α-Fe2O3 according to the literature.25,26 The values listed in Table 2 show that the IS of α-Fe2O3 decreases from 0.37 to 0.30 mm s−1 after aging, which indicates that the size of the α-Fe2O3 particle decreased by less than 10 nm. This could probably be attributed to the fact that antioxidation proceeded from the surface to the inner layer of the α-Fe2O3 particle, which resulted in a decrease in the size of α-Fe2O3 and Fe3O4 coated on the surface of the α-Fe2O3 particles (Fig. 5).
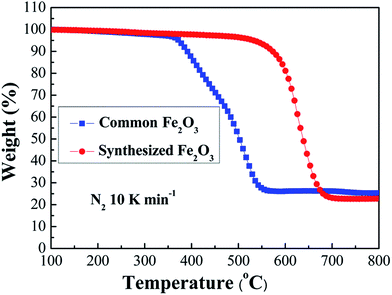 |
| Fig. 6 TGA curves of SR with different sized α-Fe2O3. The diameter of common α-Fe2O3 is 60 μm and the synthesized α-Fe2O3 is 2 μm. | |
Mechanisms of α-Fe2O3 antioxidation. The mechanical property variation of SR after aging actually indicates the significant role of α-Fe2O3 as an antioxidation additive at high temperatures. The antioxidation mechanism is proposed based on the characterization of α-Fe2O3 before and after aging. As described in Scheme 1 and 2, with oxygen at a high temperature, the side methyl groups of polysiloxane are oxidized, which lead to the generation of a series of organic free radicals.13 The Fe3+ of α-Fe2O3 captures organic free radicals, and thus Fe3+ is reduced to Fe2+, and R+ is generated simultaneously. Then, the intermediate R+ no longer destroys the polysiloxanes because it is rapidly quenched by the remaining trace of nucleophile such as amidogen, ammonia, or hydroxyl. Moreover, the outer layer of SR-1 is exposed to sufficient oxygen, thus Fe2+ is oxidized to Fe3+ again and forms a reduction–oxidation cycle. Nevertheless, in the inner layer of SR-1, the permeation rate of oxygen was very low, thus the inner layers were exposed to insufficient oxygen. Therefore, Fe2+ continues to transform to magnetic atrament Fe3O4 at high temperatures. This result well explains that the longitudinal section of SR was a sandwich structure after aging and the outer layer of SR-1 contained only α-Fe2O3. However, Fe3O4 was detected only in the inner layer. Furthermore, Fig. 7 shows that SR without α-Fe2O3 is evidently oxidized, resulting in the formation of the trifunctional (T) structure (
), which further leads to over cross linking of the polymers. Nevertheless, SR-1 exhibits the appearance of a few T structures. This result is consistent with the abovementioned variation in properties. It can be noted that the formation of the T structure is attributed to organic free radicals at high temperatures, and the fewer T structures of SR-1 also confirm that the approach of α-Fe2O3 antioxidation involves quenching or destroying of the organic free radicals.
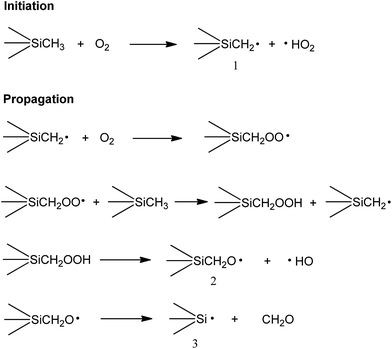 |
| Scheme 1 Free radical mechanism for the oxidation of SR. | |
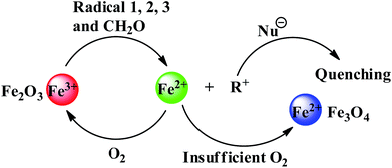 |
| Scheme 2 Mechanisms of α-Fe2O3 antioxidation. | |
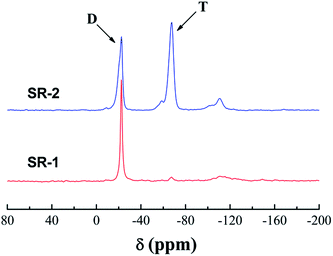 |
| Fig. 7 CPMAS 29Si NMR spectra of SR-1 and SR-2 after aging for 12 h in air. D stands for the silicon on the linear polymer and T stands for the silicon of the trifunctional structure. | |
Conclusion
The antioxidation of SR could be improved significantly by adding α-Fe2O3. Results from UV-Vis absorption spectroscopy, XRD, Mössbauer spectra, and CPMAS 29Si NMR analysis confirm the reduction–oxidation cycle of the α-Fe2O3 antioxidation mechanism. A reduction–oxidation antioxidation cycle was formed on the outer layer of SR due to the presence of sufficient oxygen. However, for the inner layer of SR, the Fe2+ ions were continuously transformed to magnetic atrament Fe3O4 at high temperatures because of insufficient oxygen. This study illustrates the mechanism of antioxidation of α-Fe2O3 and could contribute significantly to the design and synthesis of novel antioxidation additives to further improve the antioxidation of SR or other organic polymers at high temperatures.
Acknowledgements
We are grateful to the National Nature Sciences Foundation of China (No.51503211) for financial support. Special acknowledgements are given to Prof. Xie Zemin for his enduring help during the work and Mrs Guiyun Xu for her assistance on mechanical properties tests.
Notes and references
- C. J. Teng and W. P. Weber, Macromolecules, 2003, 36, 5126 CrossRef CAS.
- T. M. GÄdda, A. K. Nelson and W. P. Weber, J. Polym. Sci., Part A: Polym. Chem., 2004, 42, 5235 CrossRef.
- T. Hu, L. Dai, X. Gao, J. Xie, W. Xie, Z. Xie and Z. Zhang, J. Polym. Sci., Part A: Polym. Chem., 2014, 52, 1408 CrossRef CAS.
- J. Chojnowski, M. Cypryk, W. Fortuniak, M. Scibiorek and K. Rozga-Wijas, Macromolecules, 2003, 36, 3890 CrossRef CAS.
- M. Cypryk, K. Kaźmierski, W. Fortuniak and J. Chojnowski, Macromolecules, 2000, 33, 1536 CrossRef CAS.
- H.-F. Fei, X. Gao, X. Han, Q. Wang, T. Hu, Z. Zhang and Z. Xie, J. Polym. Sci., Part A: Polym. Chem., 2015, 53, 1023 CrossRef CAS.
- Y. P. Liu and Z. Zhang, Asian J. Chem., 2012, 24, 1141 CAS.
- X. Gao, Q. Wang, H. Sun, Y. Tan, Z. Zhang and Z. Xie, Phosphorus, Sulfur Silicon Relat. Elem., 2013, 189, 1514 CrossRef.
- H. W. Ahn and S. J. Clarson, Silicon, 2011, 3, 157 CrossRef CAS.
- T. H. Thomas and T. C. Kendrick, J. Polym. Sci., Part A-2, 1969, 7, 537 CrossRef CAS.
- T. H. Thomas and T. C. Kendrick, J. Polym. Sci., Part A-2, 1970, 8, 1823 CrossRef CAS.
- S. Luciano and H. William, Ind. Eng. Chem., 1958, 50, 1583 CrossRef.
- J. M. Nielsen, J. Polym. Sci. Chem., 1973, 40, 189 Search PubMed.
- G. Budden, J. Coated Fabr., 1998, 27, 294 CAS.
- D. K. Thomas, Polymer, 1972, 13, 479 CrossRef CAS.
- E. A. Goldovskii, R. K. Fatkulina, A. S. Kuzmunskii and A. A. Dontsov, Kauch. Rezina, 1978, 8, 17 Search PubMed.
- L.-S. Zhong, J.-S. Hu, H.-P. Liang, A.-M. Cao, W.-G. Song and L.-J. Wan, Adv. Mater., 2006, 18, 2426–2431 CrossRef CAS.
- Z. Li, H.-F. Fei, Y. Tan, X. Zhang, Z. Xie and Z. Zhang, RSC Adv., 2015, 5, 38093–38099 RSC.
- G. Zou, K. Xiong, C. Jiang, H. Li, T. Li, J. Du and Y. Qian, J. Phys. Chem. B, 2005, 109, 18356 CrossRef CAS.
- T. J. Daou, J.-M. Greneche, S.-J. Lee, S. Lee, C. Lefevre, S. Bégin-Colin and G. Pourroy, J. Phys. Chem. C, 2010, 114, 8794 CAS.
- G. F. Goya, T. S. Berquo and F. C. Fonseca, J. Appl. Phys., 2003, 94, 3520 CrossRef CAS.
- L. Q. Xu, W. Q. Zhang, Y. W. Ding, Y. Y. Peng, S. Y. Zhang, W. C. Yu and Y. T. Qian, J. Phys. Chem. B, 2004, 108, 10859 CrossRef CAS.
- S. S. Shinde, S. S. Meena, S. M. Yusuf and K. Y. Rajpure, J. Phys. Chem. C, 2011, 115, 3731 CAS.
- N. Randrianantoandro, A. M. Mercier, M. Hervieu and J. M. Greneche, Mater. Lett., 2001, 47, 150 CrossRef CAS.
- L. H. Bowen, E. De Grave and A. M. Bryan, Hyperfine Interact., 1994, 94, 1977 CrossRef CAS.
- Z. Radek, Chem. Mater., 2002, 14, 969 CrossRef.
Footnote |
† Electronic supplementary information (ESI) available: SEM images of synthesized α-Fe2O3. See DOI: 10.1039/c5ra23795e |
|
This journal is © The Royal Society of Chemistry 2016 |
Click here to see how this site uses Cookies. View our privacy policy here.