DOI:
10.1039/C5RA23783A
(Paper)
RSC Adv., 2016,
6, 281-289
Single frequency analysis for clinical immunosensor design†
Received
10th November 2015
, Accepted 5th December 2015
First published on 7th December 2015
Abstract
In the current paper, electrochemical impedance spectroscopy has been utilized for the development of an immunosensor with a different approach. The time-dependent phase angle shift technique Single Frequency Analysis, (SFA) depends on monitoring the phase angle change resulting from antibody–antigen interactions. For the purpose of designing an immunosensor an appropriate sensing matrix, namely a graphene oxide (GO)–alginate nanosphere nanocomposite structure (AlgNSp), was prepared mechanically in the presence of epichlorohydrin as the linking agent. This hydrogel based composite structure was synthesized sonochemically, a considerably new method for nanoparticle synthesis, and used as a support for GO to create a more durable film. The morphological structure and particle size were investigated using Transmission Electron Microscopy (TEM) revealing spherical structures with a diameter of 200 nm. Fourier-Transform Infrared Spectroscopy (FTIR) and Energy-Dispersive X-ray Analysis (EDAX) also showed the synthesized nanospheres to have an alginate origin. The optimum frequency value at which the most marked phase angle shift occurs was determined as 100 Hz from the Bode plots. Determination of Tau proteins between a concentration range of 250–0.01 ng mL−1 was executed selectively using the proposed system. A set of studies were carried out using biological samples obtained from patients with various neurodiseases to verify the applicability of the proposed immunosensor in clinical diagnosis. Consistency between the results obtained from the proposed system and commercial ELISA applications revealed the SFA technique to be suitable for the design of sensing systems to be used for clinical diagnosis.
1. Introduction
The sensitive and selective determination of proteins and small molecules is important in the diagnosis of most diseases, especially infectious and autoimmune diseases. Particularly, autoimmune diseases have a high prevalence in society as without the possibility of early diagnosis this results in delayed diagnosis at advanced ages with progressed symptoms.1 In this sense, the discovery of early diagnosis systems based on biomarkers that can give us information on whether a biochemical process is normal or pathologic are vital.2–4 Nowadays, in the literature, there are lots of immunosensor studies which have the potential for clinical use in the determination of biomarkers and drugs from easily obtainable samples such as blood, saliva and urine.5,6
Electrochemical Impedance Spectroscopy (EIS) is a method that enables measurement of the current by applying a sinusoidal voltage around a specific voltage value resulting in capacitance and impedance data. Surface modified electrodes tend to form a “double layer” when dipped in an electrolyte solution resulting in a specific impedance value. Any structure binding to the surface will alter the surface structure leading to a change in the double layer which in turn will change the impedance/capacitance values. As a result, this method enables the development of diagnostic systems that are able to determine picomolar levels of biological structures such as DNA,7,8 proteins,9,10 pathogens,11,12 and cells.13,14 Since this technique can also impair the biological layer and cause background signals, the method utilizing only non-faradic impedance to obtain the electrical data is thought to be more useful and accurate in relation to its faradaic counterpart. There are studies dealing with the utilization of electrical impedance (with parameters such as capacitance and phase angle) in the literature.15,16
In this study, Single Frequency Analysis (SFA) was applied to gain insight into the interactions of antigen–antibody systems. A few studies can be found in the literature which use single frequency analysis for general characterization17,18 or for chemosensing to monitor capacity change,19,20 but there isn’t a study where the complete biosensor design including the optimization, characterization, calibration and validation studies is carried out with SFA monitoring of the phase angle change. This work is the first in this regard introducing a new analysis method for biosensor technology. Initially, the frequency value which gives the best response to the graphene oxide (GO)/alginate nanosphere (AlgNSp) modified electrode is determined from the Bode plot. Evaluation of the time dependent phase angle change at this specific frequency value is the essential criterion throughout the experimental phase. Another novelty achieved in this study is the synthesis of alginate nanospheres using the sonochemical method. Although graphene oxide is one of the highly regarded conductive nanomaterials, owing to some of its physical properties individual usage is not very suitable. In this regard, alginate hydrogel was incorporated into the system acting as a binding agent for the graphene oxide. As the alginate hydrogel is not a conducting polymer its highly resistive nature was modified using sonochemical synthesis of nano-sized alginate particles followed by the generation of a suitable nanocomposite sensor matrix with graphene oxide. Anti-Tau protein was used as the model biological molecule in this study and preclinical trials were performed using samples collected from various patients with neurological disease.
2. Materials and methods
2.1. Materials
Myelin basic protein, alginate, poly(L-lysine), N-hydroxysuccinimide (NHS), 1-ethyl-3-(3-dimethylaminopropyl)carbodiimide (EDC), graphene oxide, potassium chloride (KCl), potassium ferrocyanide (K4[Fe(CN)6]), potassium ferricyanide (K3[Fe(CN)6]), acetone, sodium dihydrogen orthophosphate (NaH2PO4), and disodium hydrogen orthophosphate (Na2HPO4) were purchased from Sigma (St Louis, MO, USA). De-ionized water was purified using a Millipore Simplicity unit to a resistivity ≥ 18.2 MΩ cm. Electrochemical measurements were carried out with a Gamry PCI 750 electrochemical working station using Framework Version 5.50 software. Enzyme-Linked Immunosorbant Assay (ELISA) measurements were obtained on a Lambda Scan 200 model microplate reader and the microplates were incubated in a Memmert model incubator. FTIR measurements were carried out with a Shimadzu IRAffinity-1 model Infrared Spectrometer. A Sonics VCX 750 instrument was used for the sonochemical synthesis of the alginate nanospheres.
Serum and cerebrospinal fluid (CSF) samples were obtained from the Neurology Department, Medical School, Ankara University with 04-121-12 numbered ethical committee permission.
2.2. Synthesis of nanospheres using sonochemical gelification method
Alginate nanospheres were synthesized sonochemically for the first time and used as the matrix for biosensor applications. The method, reported by Rajaonarivony et al. (1993), was modified by our group to this end.21 Gelification was induced by the cross linking effect of Ca2+ ions with the addition of 2 mL calcium chloride (18 mM) into 38 mL of sodium alginate solution (0.1% w/v) using ultrasonification. This was followed by the addition of 16 mL poly-L-lysine (0.1% w/v) during the procedure to yield the formation of the polyelectrolyte complex. All stages of the synthesis procedure were carried out under ultrasonification conditions of 750 W and 20 kHz. The resulting nanospheres were separated using centrifugation and resuspended in water at a desired concentration. Chemical and physical characterizations of the synthesized alginate nanospheres were achieved with FT-IR and TEM.
2.3. Preparation of GO–AlgNSp–anti-Tau immunosensors and description of electrochemical study
In order to prepare the GO–AlgNSp–anti-Tau electrodes, the carboxyl active groups of GO were activated using 5% ECH (prepared in 0.8 M NaOH, the stock concentration was 15%) in an Eppendorf tube containing 250 μg mL−1 GO. This was followed by the addition of alginate nanospheres from a 2% stock solution to obtain a final concentration of 0.5%. The vortexing procedure in every step ensured homogenization. Scheme 1 is a schematic representation of the nanocomposite structure formed by the linkage of the carboxyl/hydroxyl groups of GO and the carboxyl groups of alginate due to the formation of an oxirane ring. Surface modification was achieved by drop casting 10 μL of the prepared nanocomposite solution onto the screen printed carbon electrode surface (0.2 cm2) and drying for 2 hours. A color change due to structural nanocomposite formation can be seen macroscopically in Scheme 1. Addition of anti-Tau proteins (50 μg mL−1), led to the formation of amide links between the amino groups of the protein and the active carboxyl groups of the alginate nanospheres resulting in immobilization. Finally, the protein immobilized electrode was incubated in bovine serum albumin (BSA) to prevent any non-specific bonding and washed with deionized water to remove non-immobilized proteins.
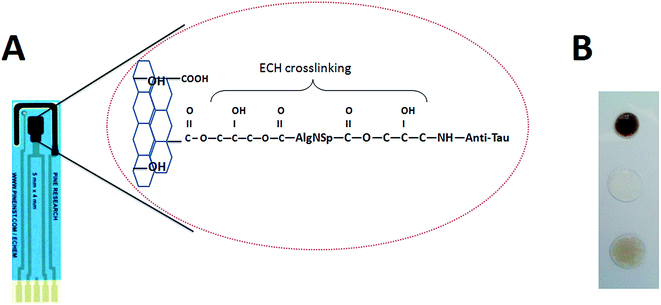 |
| Scheme 1 (A) Schematic representation of the chemical process for surface modification, (B) macroscopic images of GO (top), AlgNSp (middle), and the GO–AlgNSp nanocomposite (bottom). | |
The experiments were executed in a phosphate buffer solution (0.05 M, pH 7.4) containing 0.1 M KCl and 0.5 mM Fe(CN)63−/4−. EIS experiments were carried out in the frequency range between 100 kHz to 0.1 Hz at open circuit potential leading to the detection and evaluation of the frequency value exhibiting the highest phase angle change. This value was set as a specific frequency and single frequency experiments were carried out at this value. Parameters such as GO, AlgNSp, ECH, anti-Tau, incubation period and frequency were optimized using SFA in order to obtain the best performance. Each parameter in the development stage was repeated at least three times to ensure reproducibility.
2.4. Theory
According to the impedance theory, a Nyquist or Bode diagram is mainly composed of three regions; the high frequency part corresponding to the solution properties, the low frequency part corresponding to the electrode, in this case the transducer, and of course the middle region which actually corresponds to the metal/solution interface namely the double layer region. This is the region where almost all faradaic and non-faradaic processes occur. For the present system, the middle frequency region corresponding to the double layer capacity was chosen as the study domain.
According to complex plain impedance, both magnitude and phase are defined by eqn (2.1),
where the magnitude
Z represents the ratio of the voltage difference amplitude to the current amplitude, while
θ gives the phase difference between the voltage and current and
j is the imaginary unit.
This equation can be adapted to Ohm’s law:
The magnitude of the impedance Z acts like resistance, giving the drop in voltage amplitude across an impedance Z′ for a given current I′.
When the antigen interacts with its target antibody, an extra resistance occurs on the transducer surface, and this leads to a decrease in the phase angle of the circuit in the negative direction. By using those phase angle shifts that occur when different concentrations of antigens are applied to the immunosensor, a calibration plot of target molecule vs. phase angle shift can be derived to obtain a calibration equation for the quantitative analysis of the related target.
2.5. Biological applications
Biological applications were carried out with blood samples from multiple sclerosis, myasthenia gravis, epilepsy, Parkinson’s, and polyneuropathy patients and healthy individuals in order to evaluate the clinical potential of the immunosensor. All of the samples acquired were received in accordance with the ethical committee permission from Ankara University Medical School, Neurology Department. Blood samples were centrifuged for 5 minutes at 3000 rpm, the serum was separated and preserved at −20 °C. After dilution to 1
:
100, 10 μL of the sample was added dropwise onto the electrode surface to perform biological trials. The Tau protein levels of each sample were also tested with a commercial Tau protein ELISA kit and the developed system was validated.
3. Results
3.1. Characterization of alginate nanospheres
The morphology and size of the sonochemically synthesized alginate nanospheres were examined with Transmission Electron Spectroscopy and the mean diameter was determined as 200 nm (Fig. 1A). Energy Dispersive X-ray Analysis (EDAX) supported that the synthesized materials were alginate nanospheres (Fig. 1B). Carbon, sodium and oxygen from the carbohydrate structure of sodium alginate and calcium from the calcium chloride crosslinker can be seen easily in the EDAX spectrum.
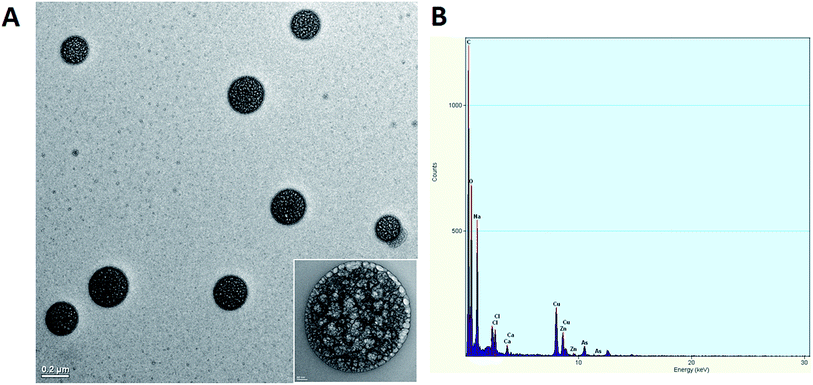 |
| Fig. 1 (A) TEM image, and (B) EDAX spectrum of AlgNSp. | |
Another method used for the characterization of the alginate nanospheres was Fourier Transform Infrared Spectroscopy. As seen in the spectra data (Fig. 2), the O–C–O carboxylate asymmetric stretching vibration at 1590–1600 cm−1, the C–OH deformation vibration with the contribution of the O–C–O symmetric stretching vibration at 1405–1410 cm−1, and the C–O stretching vibration of pyranose at 1020–1030 cm−1 are identical in the alginate (Fig. 2A), alginate nanosphere (Fig. 2B), and anti-Tau immobilized alginate nanosphere (Fig. 2C) spectra. This concluded that the alginate nanosphere synthesis was successful and the result was consistent with the previous literature studies.22,23 There is a strong amide bond peak at 1694 cm−1 in Fig. 2C instead of the carboxyl vibration peak of the alginate nanospheres at 1736 cm−1. This band, resulting from the direct reaction of the active carboxyl groups of the AlgNSp and the amine groups of the antibodies, shows the success of the immobilization step. In addition the C–N vibrations observed at 1218 cm−1 originate from the ECH based mechanism as can be seen in Scheme 1.
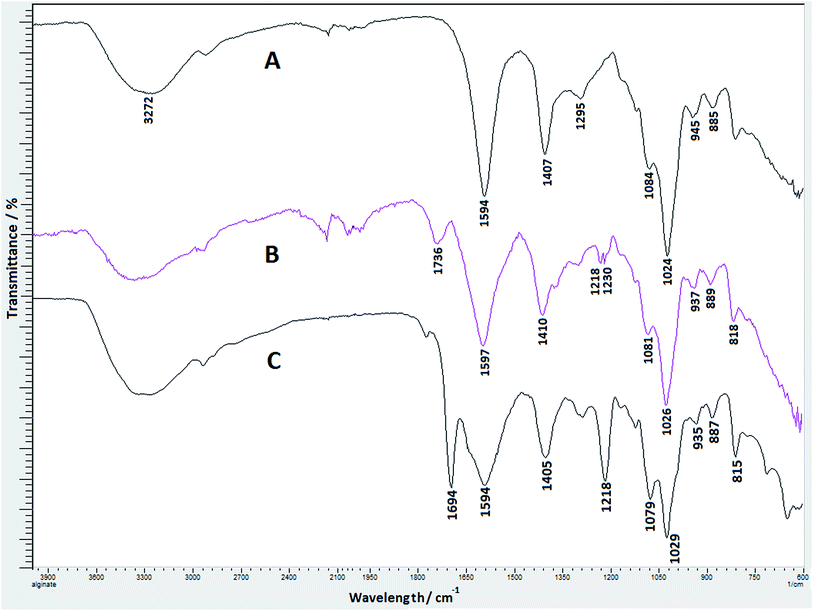 |
| Fig. 2 FTIR spectra of (A) sodium alginate biopolymer, (B) AlgNSp nanoparticles, and (C) anti-Tau immobilized AlgNSp. | |
3.2. Optimization and characterization of the GO–AlgNSp–anti-Tau immunosensor using SFA
First, frequency analysis was performed for the characterization and optimization of the GO–AlgNSp–anti-Tau immunosensor and the optimum frequency value was determined. Bode plots of the GO–AlgNSp–anti-Tau electrode were obtained and the frequency value corresponding to the maximum change in phase angle was determined, the value being 100 Hz (Fig. 3A). Maximum capacitance was observed at around 1–10 Hz and this value is consistent with the Nyquist plot (Fig. 3B). Phase angle scanning was performed at various frequency values following the Tau protein binding of the proposed system, using 100 ng mL−1 Tau protein. As a result of this effort, maximum phase angle change occurred also at 80–100 Hz (Fig. 3C) and the optimum frequency of this system was specified as 100 Hz (Fig. 3D).
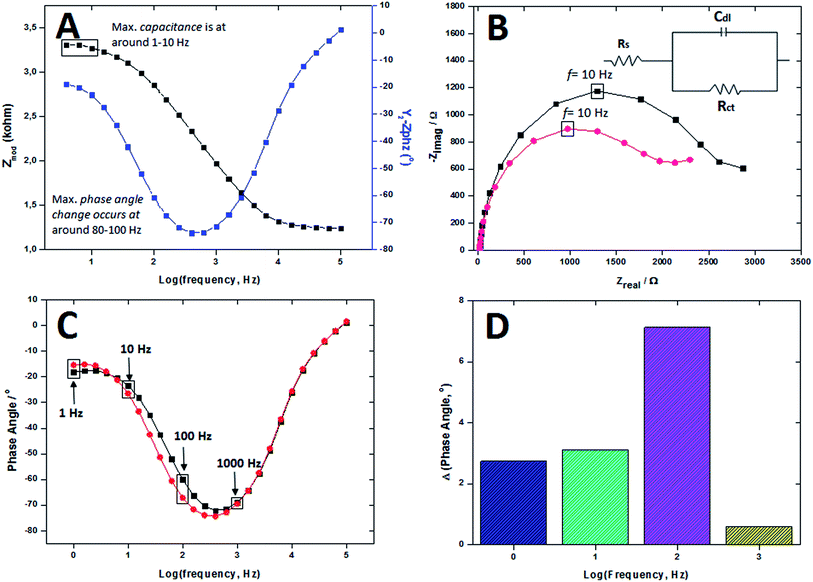 |
| Fig. 3 (A) Bode plot related to the GO–AlgNSp–anti-Tau system for recognizing the frequency range at which maximum phase angle shift occurs, (B) Nyquist plot of the GO–AlgNSp–anti-Tau system supporting the Bode plot results, (C) phase shift–log(frequency) plot for the determination of the optimum frequency at which the maximum shift occurs after the antigen–antibody interaction. The curve with spheres shows the phase shift after the Tau protein binding, (D) frequency optimization graph. | |
In order to achieve maximum performance, optimization studies for the immunosensor were carried out following the frequency optimization. For this purpose, GO concentration (0.1–0.75 mg mL−1), AlgNSp ratio (0.25–1.5%), ECH amount (0.5–5%), anti-Tau concentration (10–250 μg mL−1) and the incubation period (5–60 min) were optimized. Optimum values for GO, AlgNSp, ECH, anti-Tau and the incubation period were determined as 0.5 mg mL−1, 1%, 4%, 100 μg mL−1 and 45 minutes, respectively (Fig. S1–5†). Low amounts of GO did not provide the necessary conductivity and surface coverage. High amounts of GO cause aggregation as a result of van der Waals interactions.24 High concentrations of the alginate polymer seemed to effect the electrical features in a negative way due to poor conductivity while low concentrations were insufficient for the effective binding of GO. Low concentrations of ECH did not ensure efficient protein immobilization, whereas high concentrations caused undesired interactions between the proteins. Low amounts of the model biological agent, anti-Tau protein, were not able to provide effective protein interactions whereas high amounts tended to generate extra resistance on the electrode surface preventing electrical transmission. Short incubation periods were insufficient for the chemical binding of the protein to the surface while prolonged periods did not seem to have any effect on binding.
The experimental process of the fabricated immunosensor was characterized using the SFA method, following the frequency and optimization studies. Fig. 4 reveals a phase angle shift of 20.5° for the bare screen-printed carbon electrode. The phase angle shift increased to 29.2° for the AlgNSp modified surface. Addition of the conductive GO nanosheets decreased this value to 27.93° and further the immobilization of Tau antibodies and Tau protein incubation increased this value again (27.98° and 33.57°). These results show the success of the surface modification, immobilization and antibody–antigen interactions.
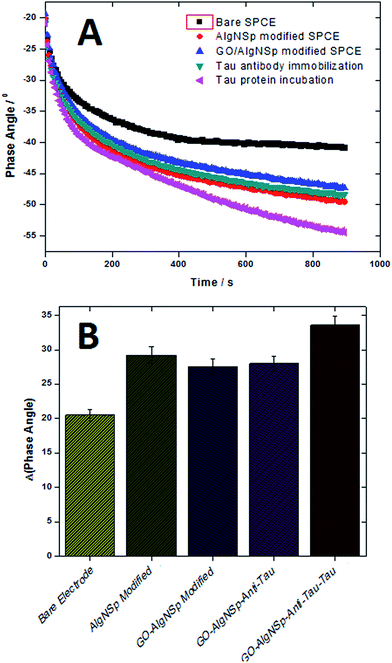 |
| Fig. 4 (A) SFA graph showing different modification steps, (B) column graph showing phase angle shifts after different modification steps. | |
3.3. Determination of Tau protein and validation of immunosensor
The interactions between the Tau antibodies and proteins were examined in a phosphate buffer solution (0.05 mM, pH 7) containing 0.1 M KCl (pH 7.4) and 5 mM [Fe(CN)6]4−/3−. The sensor signal was evaluated from Δ (phase angle) = phase angle (1) − phase angle (2) where phase angle (1) and phase angle (2) represent the GO–AlgNSp–anti-Tau immunosensor and Tau protein incubated electrode, respectively. Calibration graphs for 1–0.01 ng mL−1 (low) and 5–250 ng mL−1 (high) Tau protein concentration were acquired using phase angle changes and are shown in Fig. 5. Linear regression equations were obtained as Δ (phase angle) = (1.703 ± 0.009) + (5.91 ± 0.08) Tau protein per ng per mL for the low concentration range, and Δ (phase angle) = (7.55 ± 0.07) + (0.02 ± 7.5 × 10−4) Tau protein per ng per mL for the high calibration range.
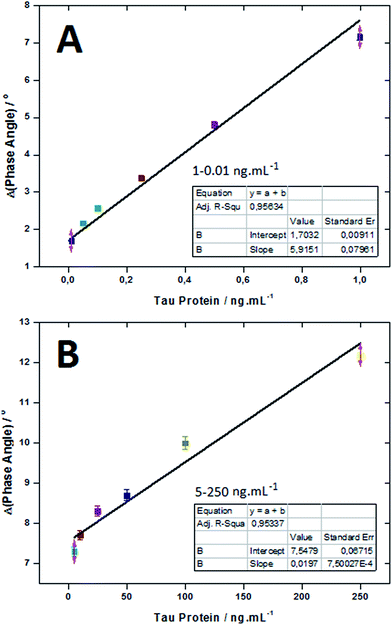 |
| Fig. 5 Calibration graphs of the GO–AlgNSp–anti-Tau immunosensor for (A) low, and (B) high concentration levels. | |
The optimized and calibrated GO–AlgNSp–anti-Tau immunosensor was further investigated for its specificity using non relevant proteins such as MBP (Myelin Basic Protein), neurofilament protein and IgG (Immunoglobulin G). To this end, 100 ng mL−1 of each of these proteins was used in the SFA experiments and the results are presented in Fig. 6A. In comparison to the 11° phase angle shift obtained for the Tau protein, MBP, neurofilament and IgG showed phase angle shifts of 1.25, 0.67 and 1.19° respectively. These values are relatively low compared to the Tau protein signal values. In addition due to the fact that the calibration curve graphs end at 1.5°, these values become irrelevant.
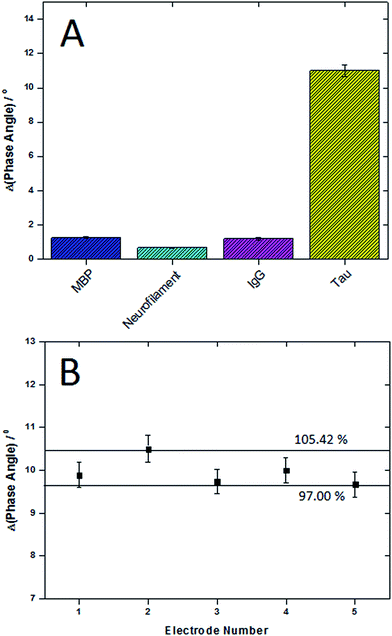 |
| Fig. 6 (A) Specificity of the immunosensor toward MBP, neurofilament, IgG, and Tau proteins. (B) Reproducibility graph showing the results obtained from 5 electrodes prepared simultaneously. | |
Another study aimed at validation was the reproducibility. For this purpose, five GO–AlgNSp–anti-Tau immunosensors were fabricated and 100 ng mL−1 Tau protein was incubated on each of these electrodes followed by the application of the SFA protocol. The electrodes revealed similar responses ranging between 97.00–105.42% (Fig. 6B). Based on these results, the reproducibility of the immunosensor seemed to be fairly good.
3.4. Pre-clinical study
The clinical potential of the developed GO–AlgNSp–anti-Tau immunosensor was evaluated using pre-clinical studies with biological samples from various neurological disease patients. Application of the biological samples onto the immunosensor was identical to the sensor development stage; drop casting of 10 μL of the sample followed by 45 minutes incubation, washing and application of the SFA protocol. The results are shown in Fig. 7. Statistical assessment of the results was performed using Analysis of Variance (ANOVA) followed by Tukey comparison in multiple biological samples. The ANOVA statistical analysis results for the proposed immunosensor and the ELISA results suggested that there were no significant differences between the results given by the two methods (P > 0.05). In experiments with healthy serum and cerebrospinal fluid and serum samples from patients with MS, myasthenia gravis, epilepsy, Parkinson’s and polyneuropathy, deviations of 8.40, 8.40, 5.27, 7.70, 5.36, 0, NA and 9.1% were observed, respectively. As the Parkinson’s sample contained Tau protein levels of 0.002 ng L−1 and because the limit of detection for the developed system was 0.01 ng L−1, this sample could not be analyzed with the proposed system. The proposed GO–AlgNSp–anti-Tau electrical impedance immunosensor looked to be suitable for clinical applications.
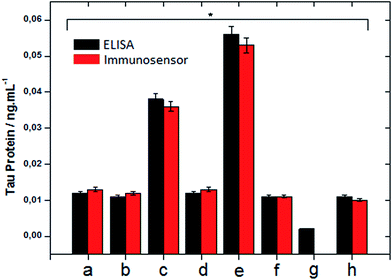 |
| Fig. 7 Pre-clinical study for Tau protein detection in biological samples obtained from patients with various neurodiseases: (a) blood, and (b) CSF samples obtained from a healthy donor; (c) serum, and (d) CSF samples obtained from a MS patient; serum samples obtained from (e) myasthenia gravis, (f) epilepsy, (g) Parkinson’s, and (h) polyneuropathy patients. The left bars show the results obtained with an ELISA study, whereas the right bars show the immunosensor results (* indicates that there was no difference between the two methods according to the Tukey test, P > 0.05). | |
Since the determination of Tau protein levels is considerably new and important in neuroclinical research, it is hard to reach a definite opinion about the relation between Tau protein levels and neurodiseases. When the clinical levels of Tau proteins in neurodiseases are investigated, one can meet with different results because of several variations such as the type of Tau protein, different types of a disease such as Relapsing Remitting Multiple Sclerosis (RRMS) and Seconder Progressive MS (SPMS), and personal differences like age or sex. For instance, in a study carried out by Zetterberg et al. (2013), it was found that the plasma Tau level of Alzheimer’s Demands (AD) patients with mild cognitive impairment (MCI) varied between 0.3 and 10 pg mL−1, whereas in the control groups it varied between 0.3–50 pg mL−1.25 In another study carried out by Bartosik-Psujek et al. (2010), Tau levels in RRMS patients (N = 30, male-11, female-19, age = 34.4) were 82.2 pg mL−1.26 In SPMS (N = 24, male-9, female-15, age = 42.8) this level is 74.2 pg mL−1. Moreover, a study introduced to the literature by Tumani et al. (2015) claimed that Tau levels show differences among different seizure types in epilepsy.27 While the highest level (319 pg mL−1) was observed in patients with status epilepticus (SE), the minimum level (139 pg mL−1) was observed in psychogenic seizures. Considering these findings, it can be said that, in neuroclinical research, Tau protein concentration can vary within pg mL−1 levels. These findings are also consistent with the results obtained in our studies where the Tau levels varied between 10–60 pg mL−1.
Considering both the sensor’s performance and the clinical results, it can be said that the proposed GO–AlgNSp–anti-Tau electrical SFA immunosensor seems suitable for clinical application.
4. Conclusion
Graphene oxide is one of the most conductive and mechanically durable materials known today and is frequently used in electrochemical sensor studies. In this work, graphene oxide, as a component of a biosensor platform, compensates for the insulation disadvantage of AlgNSp. Although graphene oxide is an exceptionally durable material, it is not able to chemically bond to inert electrode surfaces, thus it is not suitable for unaccompanied use. AlgNSp in a hydrogel form was used in order to eliminate the mechanical disadvantage. AlgNSp was synthesized with a sonochemical method that allowed the nanoparticles to be synthesized in mild conditions, over a brief time period and to a desired size. EDAX and FTIR results show that the synthesized particles have an alginate origin and the TEM results confirm that 200 nm is the average particle size.
Although SFA was used in the demonstration of antibody–antigen interactions in previous work, the entire design of a biosensor has never been carried out with a SFA method. In this work, development steps such as optimization, calibration, validation and biological trials were achieved using the SFA method and as a result, a SFA-based immunosensor was developed. The immunosensor is able to determine Tau protein at 0.01 ng mL−1 levels and this value is lower than many immunosensors that were designed with the EIS method.28–30 The proposed technique is fairly sensitive due to it being responsive to electrical change occurring at the transducer surface and enables sensitive determination of phase angle change against time. Moreover, working with a frequency specific for the proposed system enables an additional advantage to better see the alteration on the electrical layer. Thanks to the method being time-dependent, we can also see the other processes on the electrode surface, such as the detachment of biomolecules. The only disadvantage of this method is that the measurement duration is about 10–15 minutes.
Acknowledgements
This work financially supported by the Scientific and Technological Research Council of Turkey (no. 113S255).
References
- G. Cooper and B. Stroehla, Autoimmun. Rev., 2003, 2, 119–125 CrossRef PubMed.
- B. Derkus, E. Emregul, C. Yucesan and K. C. Emregul, Biosens. Bioelectron., 2013, 46, 53–60 CrossRef CAS PubMed.
- Y. Zhang, J. Bai and J. Y. Ying, Lab Chip, 2015, 15(6), 1465–1471 RSC.
- N. Cohen, P. Sabhachandani, A. Golberg and T. Konry, Biosens. Bioelectron., 2015, 66, 454–460 CrossRef CAS PubMed.
- S. R. Balakrishnan, U. Hashim, S. C. B. Gopinath, P. Poopalan, H. R. Ramayya, M. I. Omar, R. Haarindraprasad and P. Veeradasan, PLoS One, 2015, 10(9), e0137891 CAS.
- M. Giannetto, M. Mattarozzi, E. Umiltra, A. Manfredi, S. Quaglia and M. Careri, Biosens. Bioelectron., 2014, 62, 325–330 CrossRef CAS PubMed.
- T. Rolim, J. Cancino and V. Zucolotto, Biomed. Microdevices, 2015, 17(1), 3 CrossRef PubMed.
- M. Riedel, J. Kartchemnik, M. J. Schöning and F. Lisdat, Anal. Chem., 2014, 86, 7867–7874 CrossRef CAS PubMed.
- M. Johari-Ahar, M. R. Rashidi, J. Barar, M. Aghaie, D. Mohammednejad, A. Ramazani, P. Karami, G. Cougos and Y. Omidi, Nanoscale, 2015, 7, 3768–3779 RSC.
- B. Derkus, E. Emregul, K. C. Emregul and C. Yucesan, Sens. Actuators, B, 2014, 192, 294–302 CrossRef CAS.
- A. Shabani, C. A. Marquette, R. Mandeville and M. F. Lawrence, Talanta, 2013, 116, 1047–1053 CrossRef CAS PubMed.
- N. Couniot, T. Vanzieleghem, J. Rasson, N. Overstraeten-Schlögel, O. Poncelet, J. Mahillon, L. A. Francis and D. Flandre, Biosens. Bioelectron., 2015, 67, 154–161 CrossRef CAS PubMed.
- H. Yu, J. Wang, Q. Liu, W. Zhang, H. Cai and P. Wang, Biosens. Bioelectron., 2011, 26, 2822–2827 CrossRef CAS PubMed.
- M. Abdolahad, M. Janmaleki, M. Taghinejad, H. Taghnejad, F. Salehi and S. Mohajerzadeh, Nanoscale, 2013, 5, 3421 RSC.
- H. Shafiee, M. Jahangir, F. Inci, S. Wang, R. B. M. Willenbrecht, F. F. Giguel, A. M. N. Tsibris, D. R. Kuritzkes and U. Demirci, Small, 2013, 9(15), 2553–2563 CrossRef CAS PubMed.
- H. Abiri, M. Abdolahad, M. Gharooni, S. A. Hosseini, M. Janmaleki, S. Azimi, M. Hosseini and S. Mohajerzadeh, Biosens. Bioelectron., 2015, 68, 577–585 CrossRef CAS PubMed.
- B. Ozcan, B. Demirbakan, G. Yesiller and M. K. Sezginturk, Talanta, 2014, 125, 7–13 CrossRef CAS PubMed.
- M. N. Sonuc and M. K. Sezginturk, Talanta, 2014, 120, 355–361 CrossRef CAS PubMed.
- T. P. Huynh, A. Pietrzyk-Le, K. C. Bikram, K. R. Noworyta, J. W. Sobczak, P. S. Sharma, F. D’Souza and W. Kutner, Biosens. Bioelectron., 2013, 41, 634–641 CrossRef CAS PubMed.
- P. S. Sharma, M. Dabrowski, K. Noworyta, T.-P. Huynh, K. C. Chandra, J. W. Sobczak, P. Pieta, F. D’Souza and W. Kutner, Anal. Chim. Acta, 2014, 844, 61–69 CrossRef CAS PubMed.
- M. Rajaonarivony, C. Vauthier, G. Couvrraze, F. Puisieux and P. Couvreur, J. Pharm. Sci., 1993, 82(9), 912 CrossRef CAS PubMed.
- D. Leal, B. Matsuhiro, M. Rossi and F. Caruso, Carbohydr. Res., 2008, 343, 308–316 CrossRef CAS PubMed.
- B. Sarmento, D. Ferreira, F. Veiga and A. Riberio, Carbohydr. Polym., 2006, 66, 1–7 CrossRef CAS.
- D. Li, M. B. Muller, S. Gilje, R. B. Kaner and G. G. Wallace, Nat. Nanotechnol., 2008, 3, 101–105 CrossRef CAS PubMed.
- H. Zetterberg, D. Wilson, U. Andreasson, L. Minthon, K. Blennow and J. Randall, Alzheimer’s Res. Ther., 2013, 5, 9 CrossRef CAS PubMed.
- H. Bartosik-Psujek, M. Psujek, J. Jaworski and Z. Stelmasiak, Acta Neurol. Scand., 2010, 123(4), 252–256 CrossRef PubMed.
- H. Tumani, C. Jobs, J. Brettschneider, A. C. Hoppner, F. Kerling and S. Fauser, Epilepsy Res., 2015, 114, 23–31 CrossRef CAS PubMed.
- G. C. Jimenez, S. Eissa, A. Ng, H. Alhadrami, M. Zourob and M. Siaj, Anal. Chem., 2015, 87, 1075–1082 CrossRef PubMed.
- S. Li, H. Cui, Q. Yuan, J. Wu, A. Wadhwa, S. Eda and H. Jiang, Biosens. Bioelectron., 2014, 51, 437–443 CrossRef CAS PubMed.
- P. Mashazi, P. Tetyana, S. Vilakazi and T. Nyokong, Biosens. Bioelectron., 2013, 49, 32–38 CrossRef CAS PubMed.
Footnote |
† Electronic supplementary information (ESI) available. See DOI: 10.1039/c5ra23783a |
|
This journal is © The Royal Society of Chemistry 2016 |