DOI:
10.1039/C5RA23373A
(Paper)
RSC Adv., 2016,
6, 2046-2054
Photoluminescence tuning via energy transfer in Eu-doped Ba2(Gd,Tb)2Si4O13 solid-solution phosphors
Received
6th November 2015
, Accepted 16th December 2015
First published on 18th December 2015
Abstract
We report on the phase formation of a Ba2(Gd,Tb)2Si4O13 solid-solution, and the coexistence of Eu2+/Eu3+ was identified after Eu ion doping although the samples were prepared in a reducing atmosphere. Under 377 nm near-ultraviolet (UV) light excitation, Ba2Tb2Si4O13 exhibits the characteristic emission originating from Tb3+ corresponding to 5D4–7F6,5,4,3 transitions; whereas Ba2Tb2Si4O13:Eu emits bright red emission from Eu3+ with peaks around 594, 613 and 623 nm. Accordingly, photoluminescence tuning of Eu-doped Ba2(Gd,Tb)2Si4O13 phosphors has been realized from green, yellow, orange, to red emission light. Decay time and time-resolved luminescence results revealed that the tunable luminescence behavior should be ascribed to the existence of energy migration in the terbium subset, and successive energy transfer processes Eu2+–Eu3+(Tb3+) and Tb3+–Eu3+ appear to occur in the Ba2Tb2−ySi4O13:yEu (y = 0–0.12) solid-solution phosphors under investigation.
1. Introduction
Lanthanide-doped inorganic phosphor materials have attracted much attention in recent years owing to their potential applications in display technology, solid state lighting, and other fields.1,2 Amongst them, Eu ions have been widely used as activators because both Eu3+ and Eu2+ can function as emission centers in the host lattices.3 Compared with the 4f–5d transition of Eu2+, the emission of the 4f–4f transition of Eu3+ is relatively insensitive to the host and temperature because the 4f shell is shielded by the outer filled 5s and 5p shells. However, the 4f–5d transition of Eu2+ is parity allowed and therefore intense emission bands can be achieved. In addition, for Eu2+ the absorption and emission wavelengths of can be tuned by the host lattice because of the strong interaction between the 5d electron and the ligand anions through covalency and crystal field. Eu3+ ions are employed in luminescent devices such as fluorescent lamps and cathode ray tubes, and phosphors containing Eu3+ ions as activators can emit red light; their excitation bands usually consist of host lattice excitation bands, charge-transfer bands, and direct excitation bands of Eu3+ ions.4 However, the low oscillator strength and narrow line width of Eu3+ 4f–4f absorption transitions leads to a weak absorption in the near-UV and blue region.5 As a comparison, Eu2+ emits in the UV- to visible region and the emission wavelength strongly depends on the nature of the host lattice due to the participation of d orbitals. Therefore, as the characteristic broad-band transition excitation and emission of Eu2+ have been well recognized, and it might be very exciting and interesting if an efficient Eu2+–Eu3+ energy transfer can occur in a single-phase valence-varied Eu-doped phosphor, which can provide an opportunity to tailor the absorption of red-emitting phosphors containing Eu3+ ions. However, the existence of metal–metal charge transfer (MMCT) effect will quench the luminescence of the sensitizer for the designed Eu2+–Eu3+ energy transfer. Therefore, Setlur firstly proposed that energy migration in the terbium subset can be used as an intermediate to alleviate the MMCT effect.6 After that, such a strategy has been realized in Ba3Ln(PO4)3:Ce3+,Tb3+,Eu3+, LnPO4:Ce3+,Tb3+,Eu3+, and Ba2(Ln1−zTbz)(BO3)2Cl:Eu phosphors systems, and some interesting luminescence properties have been observed.7,8 In the present work, Eu-doped Ba2(Gd,Tb)2Si4O13 solid-solution phosphors have been designed, the coexistence of Eu2+/Eu3+ has been evidenced and energy transfer of the type Eu2+–Eu3+(Tb3+) and Tb3+–Eu3+ has been observed.
The crystal structures of the Ba2Gd2−xTbxSi4O13 phase under investigation were derived from the Ba2Gd2Si4O13 phase via Gd/Tb substitution. Ba2Gd2Si4O13 possesses a monoclinic structure and a space group C2/c with lattice parameters a = 12.896(3) Å, b = 5.212(1) Å, c = 17.549(4) Å, β = 104.08(3) Å.9 Up to now, several studies regarding the luminescence properties of rare earth doped Ba2Gd2Si4O13 have been reported. Guo et al. investigated the tunable photoluminescence properties of Ba2Gd2Si4O13:Ce3+,Tb3+,10 and the spectroscopic properties of Ba2Gd2Si4O13:Eu3+.11 Zhang et al. studied the vacuum UV spectroscopic properties of Ba2Gd2Si4O13:Ln3+ (Ln3+ = Ce3+, Tb3+, Dy3+, Eu3+, Sm3+).12 In 2015, Zhou et al. studied the green emitting phosphor BaGd2Si4O13:Eu2+.13 Herein, we have investigated the phase formation of Ba2(Gd,Tb)2Si4O13, and the coexistence of Eu2+/Eu3+; the energy transfer processes Eu2+–Eu3+(Tb3+) and Tb3+–Eu3+ in Ba2Tb2Si4O13 were investigated in detail.
2. Experimental section
2.1 Sample preparation
Ba2Gd2−xSi4O13:xTb and Ba2Tb2−ySi4O13:yEu phosphors were synthesized by high temperature solid–state reaction, starting from a mixture containing commercially-available BaCO3 (99.9%), Gd2O3 (99.995%), SiO2 (99.95%), Tb4O7 (99.995%) and Eu2O3 (99.995%) in the given stoichiometric proportions. After mixing and grinding, the mixtures were placed into an alumina crucible and then fired in air at 1360 °C for 5 h under a 5% H2/N2 gas mixture. After this, the samples were furnace-cooled to room temperature, and ground again into powder for the following measurements.
2.2 Characterization methods
The powder X-ray diffraction (XRD) measurements were conducted on a D8 Advance diffractometer (Bruker Corporation, Germany) operating at 40 kV and 40 mA with Cu Kα radiation (λ = 0.15406 Å), and the scanning rate was fixed at 4° min−1. Diffuse reflectance spectra were measured on a UV-vis-NIR spectrophotometer (SHIMADZU UV-3600) attached to an integrating sphere. BaSO4 was used as a reference standard. Room temperature photoluminescence (PL) excitation and emission spectra were measured on a fluorescence spectrophotometer (F-4600, HITACHI, Japan) with a photomultiplier tube operating at 400 V, and a 150 W Xe lamp used as the excitation lamp. The time-resolved PL spectra were measured by an Edinburgh FLS920 spectrofluorometer with the monitoring wavelength of 377 nm, and the room-temperature decay curves were recorded on the same spectrofluorometer and the corresponding wavelengths (365 nm for Eu2+ emission, 377 for Tb3+ emission and 393 nm for Eu3+ emission) were used to monitor the decay. The temperature-dependent luminescence properties were measured on the same spectrophotometer, which was combined with a self-made heating attachment and a computer-controlled electric furnace. Quantum efficiency was measured using the integrating sphere on the FLS920 fluorescence spectrophotometer (Edinburgh Instruments Ltd., UK).
3. Results and discussion
Fig. 1(a) and (b) show the typical XRD patterns of the as-prepared Ba2Gd2−xSi4O13:xTb (x = 0, 0.5, 1.0, 1.5, 2.0) and Ba2Tb2−ySi4O13:yEu (y = 0, 0.01, 0.03, 0.06, 0.15, 2.0) samples, respectively. It can be found that all the diffraction peaks of these two series of samples can be exactly assigned to the corresponding standard data for the hexagonal phase of Ba2Gd2Si4O13 (ICSD card no. 260737), indicating that this series of Ba2Gd2−xSi4O13:xTb (x = 0, 0.5, 1.0, 1.5, 2.0) solid solution samples with different Tb/Gd ratios can form a single phase, and a small amount of Eu also will not change the crystal structure. We find that the characteristic diffraction peak (1 1 4) shifts to higher diffraction angles from 29.28° to 29.40° for Ba2Gd2−xSi4O13:xTb, while it shifts to lower diffraction angles from 29.40° to 29.22° for Ba2Tb2−ySi4O13:yEu. This variation could be due to the different ionic radii for Gd3+, Tb3+ and Eu3+, further suggesting that a continuous solid solution of Ba2Gd2−xSi4O13:xTb and Ba2Tb2−ySi4O13:yEu has formed in the crystal structure.
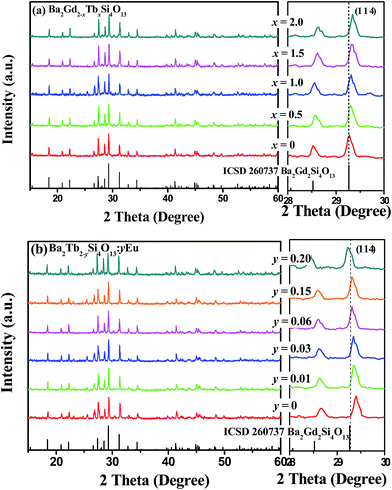 |
| Fig. 1 XRD patterns of as-prepared (a) Ba2Gd2−xSi4O13:xTb (x = 0, 0.5, 1.0, 1.5, 2.0) and (b) Ba2Tb2−ySi4O13:yEu (y = 0, 0.01, 0.03, 0.06, 0.15, 2.0) samples. The standard data for Ba2Gd2Si4O13 (ICSD card no. 260737) is shown as a reference. | |
The evolution of the unit cell parameters a and V of Ba2Gd2−xSi4O13:xTb and Ba2Tb2−ySi4O13:yEu are given in Fig. 2(a) and (b), as a function of x and y, respectively. The linear behavior of the cell parameters illustrates that these two series of compounds both belong to a continuous iso-structural solid solution. Fig. 2(c) presents the crystal structure of Ba2(Gd,Tb)2Si4O13. The structure is based on finite zigzag-shaped C2-symmetric Si4O13 chains and Gd2O12 dimers built of edge-sharing GdO7 polyhedra of C1 symmetry. The [9 + 1]-coordinated Ba atoms are located in voids of the atomic arrangement, as highlighted in Fig. 2(d).
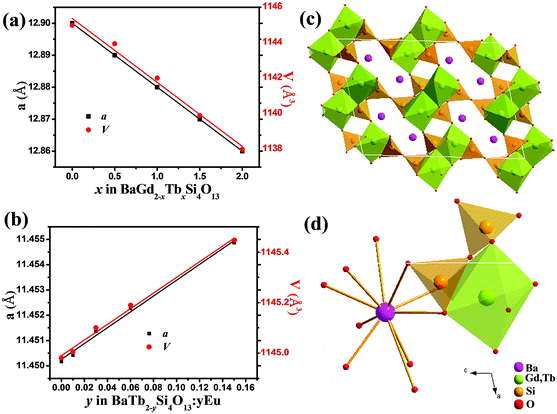 |
| Fig. 2 Unit cell parameters a, V of (a) Ba2Gd2−xSi4O13:xTb (x = 0, 0.5, 1.0, 1.5, 2.0) and (b) Ba2Tb2−ySi4O13:yEu (y = 0, 0.01, 0.03, 0.06, 0.15)are plotted. (c) Crystal structure of Ba2Gd2Si4O13 viewed along the b axis. The SiO4 tetrahedra and GdO7 polyhedra are highlighted in the unit cell, and Ba2Tb2Si4O13 is isostructural with Ba2Gd2Si4O13. (d) The bonding of O2− anions with Ba2+, Si4+ and Gd3+ cations in the independent part of the Ba2(Gd,Tb)2Si4O13 unit cell. | |
Room temperature photoluminescence excitation (PLE) and emission (PL) spectra of the Ba2Gd1.99Si4O13:0.01Eu phosphors are shown in Fig. 3(a). Upon excitation at 365 nm, the PL spectra of Ba2Gd1.99Si4O13:0.01Eu present a broad band in the green region (350–600 nm) and several small peaks on the shoulders near 600 nm. It is well known that Eu2+ ions provide a broad emission band corresponding to the 4f65d–4f7 transition, while Eu3+ ions emit discrete narrow lines belonging to the 5D0–7FJ transitions (J = 0–6). Moreover, the two excitation spectra for Ba2Gd1.99Si4O13:0.01Eu give the corresponding typical spectral profile for the two ions. Although the europium precursor was Eu2O3 containing the trivalent ion and the samples were prepared in a reducing atmosphere, it seems that the trivalent europium coexists with divalent europium in this compound. In order to prove it, a Eu3+ singly doped Ba2Gd2Si4O13 phosphor was prepared in air and its PLE and PL spectra were comparatively investigated. As also shown in Fig. 3(a), there are three narrow peaks centered at 594, 613 and 624 nm in the PL spectrum of Ba2Gd1.99Si4O13:0.01Eu3+ upon 393 nm excitation, which agrees with the position of Eu3+ emission in Ba2Gd1.99Si4O13:0.01Eu2+/Eu3+. Furthermore, the PLE spectral profile of Ba2Gd1.99Si4O13:0.01Eu3+ is similar to that of Ba2Gd1.99Si4O13:0.01Eu2+/Eu3+ monitored at 613 nm; this confirms the consistence of Eu2+ and Eu3+ in the Ba2Gd2Si4O13 host when prepared in reducing atmosphere. Fig. 3(b) shows the PLE (λem = 543 nm) and PL (λem = 377 nm) spectra of Ba2Gd1.99Si4O13:0.01Tb3+. The PLE spectrum observed in the range of 200–300 nm was attributed to the 4f8–4f75d1 (7F6–7D) transition of Tb3+ and the one in the range of 300–400 nm come from the 4f–4f transition of Tb3+. The Tb3+ emission lines are located at 487 nm, 542 nm, 585 nm, and 624 nm, and are assigned to the 5D4–7F6,5,4,3 transitions, respectively.14
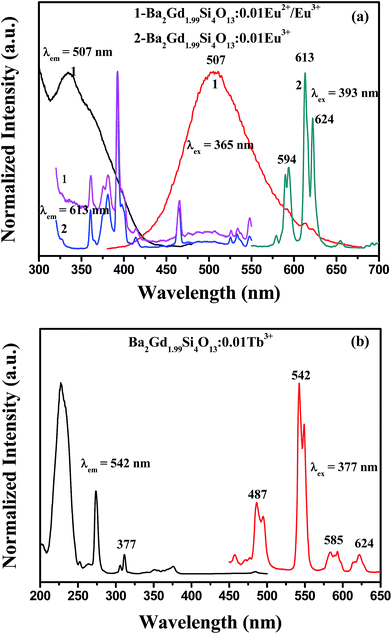 |
| Fig. 3 PLE and PL spectra of (a) Ba2Gd1.99Si4O13:0.01Eu2+/Eu3+ prepared in a reducing atmosphere, Ba2Gd1.99Si4O13:0.01Eu3+ prepared in air, and (b) Ba2Gd1.99Si4O13:0.01Tb3+ prepared in air. | |
The decay curves and lifetime values of Eu2+/Eu3+ (excitation wavelength 365 nm for Eu2+ and 393 nm for Eu3+) and Tb3+ (excitation wavelength 377 nm) in Ba2Gd1.99Si4O13:0.01Eu/Tb phosphor are shown in Fig. 4. Since there are several different cations with variable environments for the doped rare earth ions in this system, we have tried different exponential functions. It is found that, in order to get the best fitting (with the confidence coefficient of 99%) and evaluate the decay time, the experimental curves were fitted by the sum of two exponential decays using the formula:15
|
I(t) = A1 exp(−t/τ1) + A2 exp(−t/τ2)
| (1) |
where
I is the luminescence intensity,
A1 and
A2 are constants,
τ is the time,
τ1 and
τ2 are the rapid and slow decay exponential components, respectively. On this basis, the effective decay constant (
τ*) can be calculated as:
16 |
τ* = (A1τ12 + A2τ22)/(A1τ1 + A2τ2)
| (2) |
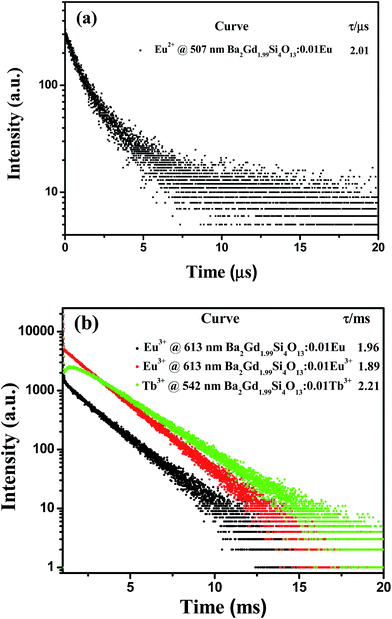 |
| Fig. 4 (a) Decay time curves and the corresponding lifetime values of Eu2+ in Ba2Gd1.99Si4O13:0.01Eu phosphor. (b) Eu3+ and Tb3+ decay curves of Ba2Gd1.99Si4O13:0.01Eu/Tb phosphor. | |
The effective decay time (τ*) were calculated to be 2.01 μs, 1.96 ms, 1.89 ms and 2.21 ms for the 4f65d–4f7 (Eu2+ emission at 507 nm) in Ba2Gd1.99Si4O13:0.01Eu, 5D0–7F2 (Eu3+ emission at 613 nm) in Ba2Gd1.99Si4O13:0.01Eu, 5D0–7F2 (Eu3+ emission at 613 nm) in Ba2Gd1.99Si4O13:0.01Eu3+, and 5D4–7F5 (Tb3+ emission at 543 nm) in Ba2Gd1.99Si4O13:0.01Tb3+, respectively. The decay profile of the 5D4 emission for the latter sample shows a clear rise at short times, due to the slow relaxation from 5D3 to 5D4, attributed to multiphonon relaxation in the silicate host containing high frequency vibrations. The non-exponential character of the decay profiles is presumably due to the presence of several slightly different sites for the dopants and, in the case of Ba2Gd1.99Si4O13:0.01Eu, to possible Eu2+–Eu3+ energy transfer processes.
The possibility of the Eu2+/Eu3+ coexistence can be verified by the luminescence behavior. Fig. 5(a) shows the PLE and PL spectra of Ba2Tb2Si4O13. It can be seen that the PLE spectrum of Tb3+ exhibits two obvious broad bands from 200 to 300 nm with two peaks at 252 and 279 nm and some weak transitions from 300 to 400 nm. Fig. 5(b) represents the PLE and PL spectra of Ba2Tb1.97Si4O13:0.03Eu obtained at different experimental conditions. When Eu ions with a low concentration (y = 0.03) were introduced into this matrix, the PLE spectrum of Ba2Tb1.97Si4O13:0.03Eu phosphor prepared in reducing atmosphere has been obviously broadened and the intensity of the sharp lines corresponding to the emission of Tb3+ has obviously increased. Moreover, the excitation band edge has shifted to 450 nm, showing a broad-band character. By comparing the two excitation bands of Ba2Tb1.97Si4O13:0.03Eu phosphor obtained at different experimental conditions, there is an obvious difference in the excitation band owing to the appearance of a broad excitation feature, which supports the existence of Eu2+. Upon excitation at 377 nm, the Tb3+ emission intensity decreases accompanied by a remarkable increase of the emission intensities at 594, 613, and 624 nm for Ba2Tb1.97Si4O13:0.03Eu phosphor, which are the characteristic red emission peaks of Eu3+ ions corresponding to the transitions from 5D0 to 7FJ (J = 1 and 2).17 Generally, we can propose that the enhancement of the red-emitting luminescence can be ascribed to the energy transfer from Tb3+ to Eu3+.18,19 Furthermore, it is notable that the emission intensity of the Ba2Tb1.97Si4O13:0.03Eu phosphor prepared in reducing atmosphere is relatively higher than the one of the Ba2Tb1.97Si4O13:0.03Eu fired in air, indicating an efficient energy transfer between Eu2+–Eu3+ (Tb3+) and the Eu2+ absorption.
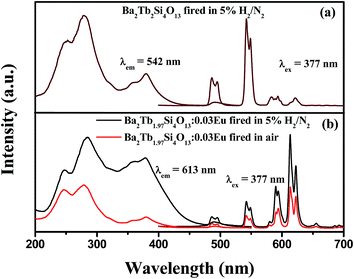 |
| Fig. 5 PLE and PL spectra of (a) Ba2Tb2Si4O13 and (b) Ba2Tb1.97Si4O13:0.03Eu phosphor. | |
The diffuse reflectance spectra of Ba2Gd2−xSi4O13:xTb (x = 0, 0.5, 1.0, 1.5, 2.0) phosphors are shown in Fig. 6(a). The spectrum of the Ba2Gd2Si4O13 host shows a high reflectance in the visible range, and the band gap estimation in the Ba2Gd2Si4O13 host is shown by the inset in Fig. 6(a). The band gap of the Ba2Gd2Si4O13 host can be estimated according to eqn (3)20
|
[F(R∞)hν]n = A(hν − Eg)
| (3) |
where
hν is the photon energy;
A is a proportional constant;
Eg is the value of the band gap;
n = 2 for a direct transition or 1/2 for an indirect transition; and
F(
R∞) is the Kubelka–Munk function defined as
21 |
F(R∞) = (1 − R)2/2R = K/S
| (4) |
where
R,
K, and
S are the reflection, absorption, and scattering coefficient, respectively. From the linear extrapolation of [
F(
R∞)
hν]
2 = 0 in the inset of
Fig. 6(a), the
Eg value was estimated to be about 4.20 eV. As Tb
3+ ions were doped into the Ba
2Gd
2Si
4O
13 host, a strong absorption in the range of 200–350 nm assigned to the f–d transition of Tb
3+ was observed, as found in the excitation spectrum. The
Eg value gradually increases, and the absorption intensity is enhanced at higher Tb
3+ ion concentrations. As a comparison, Ba
2Tb
2−ySi
4O
13:
yEu phosphors show an obvious broad absorption band near 400 nm (
Fig. 6(b)), which should be caused by the 4f
7–4f
65d
1 transition of Eu
2+.
22 The reflectance spectrum and PLE spectrum indicate that the absorption of Ba
2Tb
2Si
4O
13:Eu phosphor matches well with near-UV chips for applications in near-UV LEDs.
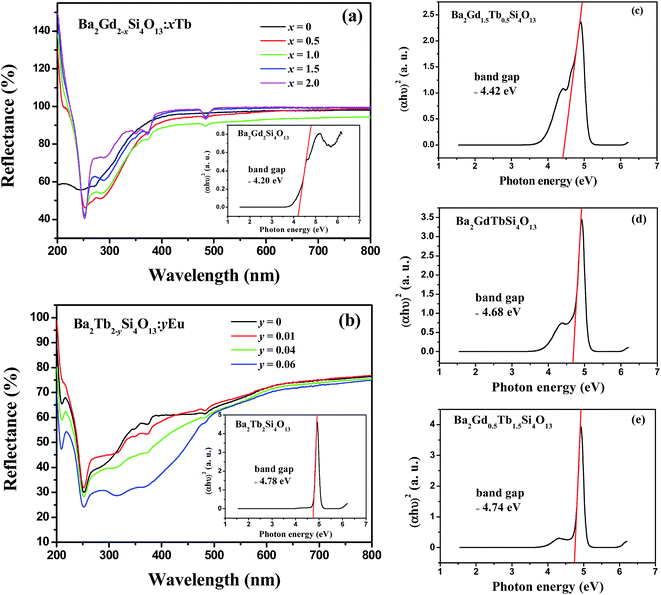 |
| Fig. 6 The diffuse reflectance spectra of (a) Ba2Gd2−xSi4O13:xTb (x = 0, 0.5, 1.0, 1.5, 2.0) and (b) Ba2Tb2−ySi4O13:yEu (y = 0, 0.01, 0.04, 0.06) phosphors. The extrapolation of the band gap energy for (inset of a) Ba2Gd2Si4O13, (inset of b) Ba2Tb2Si4O13, (c) Ba2Gd1.5Tb0.5Si4O13, (d) Ba2GdTbSi4O13, and (e) Ba2Gd0.5Tb1.5Si4O13. | |
Fig. 7(a) illustrates the emission spectra of Ba2Tb2−ySi4O13:yEu (y = 0–0.12) samples upon excitation at 377 nm. With increasing Eu concentration, one can see that the relative emission intensities of Tb3+ becomes weak, whereas the emission intensities of Eu3+ firstly increase until they reach saturation at y = 0.03, reflecting the result of energy transfer from Tb3+ to Eu3+. In addition, it is surprising to find the appearance of a broad band centered around 507 nm with increasing y, which can be assigned to the 4f65d1–4f7 transition of Eu2+, further supporting the coexistence of Eu2+ and Eu3+.23 Because of the Eu2+–(Tb3+)n–Eu3+ energy transfer process, the CIE coordinates upon 377 nm excitation of Ba2Tb2−ySi4O13:yEu (y = 0, 0.01, 0.03, 0.09) progressively shift from green (0.2966, 0.5798) to red (0.5048, 0.3955) with increasing y as displayed in Fig. 7(b). As also given in the photographs of the emitting phosphors, the as-observed emitting color is obvious, which means that tunable luminescence can be realized in the novel Ba2Tb2−ySi4O13:yEu phosphors via Eu2+–(Tb3+)n−Eu3+ energy transfer processes.
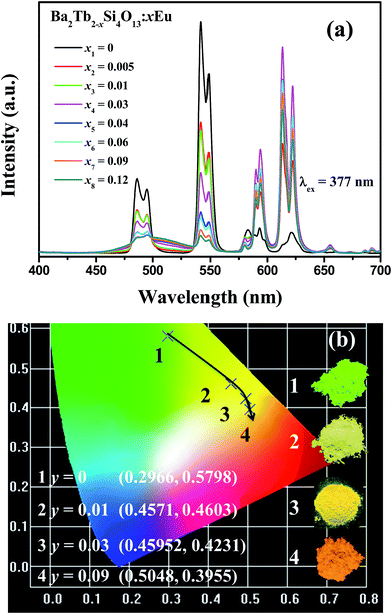 |
| Fig. 7 (a) PL spectra for the phosphors Ba2Tb2−ySi4O13:yEu (y = 0, 0.005, 0.01, 0.03, 0.04, 0.06, 0.09, 0.12) phosphors, and (b) the corresponding CIE coordinates and photographs. | |
In order to demonstrate the energy transfer from Tb3+ to Eu3+, the decay curves of Tb3+ emission at 542 nm and Eu3+ emission at 613 nm of Ba2Tb2Si4O13 and Ba2Tb1.995Si4O13:0.005Eu phosphors are shown in Fig. 8(a) (excitation at 377 nm). Using eqn (1) and (2), the values of the effective decay time were found to be 2.59, 0.96 and 1.83 ms for the 5D4–7F5 (Tb3+ emission at 543 nm) in Ba2Tb2Si4O13, 5D4–7F5 (Tb3+ emission at 543 nm) in Ba2Tb1.995Si4O13:0.005Eu, and 5D0–7F2 (Eu3+ emission at 613 nm) in Ba2Tb1.995Si4O13:0.005Eu, respectively. Accordingly, the energy transfer efficiency (ηT) can be calculated using the following expression:24
|
 | (5) |
here
τ0 and
τx are the corresponding lifetime values of donor Tb
3+ in the absence and the presence of the acceptor Eu
3+, and
ηT is the energy transfer efficiency. As shown in the inset of
Fig. 8(a), the total
ηT is found to be 62.9% from Tb
3+ to Eu
3+ in the Ba
2Tb
1.995Si
4O
13:0.005Eu phosphor. We also measured the decay curves of the Ba
2Tb
2−ySi
4O
13:
yEu phosphors excited at 377 nm, as given in
Fig. 8(b). It was found that the lifetimes for Tb
3+ decreased with increasing Eu concentration, which offers clear evidence for the presence of energy transfer from Tb
3+ to Eu
3+, and the energy transfer efficiencies are 62.9%, 68.7%, 73.7%, 81.5% and 89.2% depending on different Eu concentration, 0.005, 0.01, 0.02, 0.03 and 0.06, respectively.
Fig. 8(c) shows the decay curves of the
5D
4–
7F
5 transition of Tb
3+ upon excitation at 273, 365, and 377 nm. Based on the decay curves in
Fig. 8(c),
eqn (1) and
(2), the value of decay time were found to be practically independent of the excitation wavelength, with values 0.49, 0.45 and 0.48 ms for excitation of 273, 365, and 377 nm, respectively.
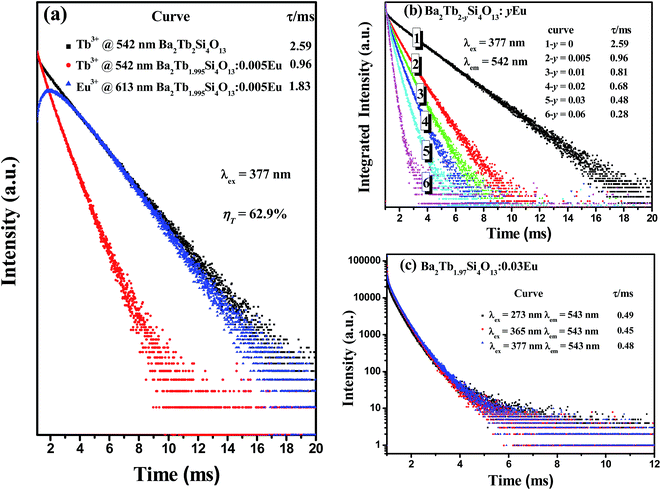 |
| Fig. 8 (a) Decay time curves and lifetimes of Eu3+ in Ba2Tb1.995Si4O13:0.005Eu phosphor and Tb3+ in Ba2Tb2Si4O13 and Ba2Tb1.995Si4O13:0.005Eu phosphor. (b) Tb3+ decay curves of Ba2Tb2−ySi4O13:yEu phosphors monitoring 542 nm emission. (c) Tb3+ decay curves of Ba2Tb1.97Si4O13:0.03Eu phosphors monitoring 542 nm emission under 273, 365 and 377 nm excitation. | |
To study the energy transfer process from Tb3+ to Eu3+ in Ba2TbSi4O13:Eu phosphor in more detail, time-resolved emission spectra of Ba2Tb1.995Si4O13:0.005Eu were measured upon excitation into the Tb3+ band with a delay time ranging from 0 to 3.12 ms (Fig. 9). At t = 0 ms, only the characteristic emission of Tb3+ is observed; no Eu3+ emission is present because the excitation energy Tb3+ has not been transferred yet to Eu3+. As also shown in Fig. 9, when t = 0.78 ms, the emission of Tb3+ starts to decrease accompanied by the presence of Eu3+ emissions due to the efficient energy transfer of Tb3+ to Eu3+. The emission of Tb3+ decreases gradually with further increase of the decay time, whereas that of Eu3+ begins to increase due to transfer of more excitation energy from Tb3+ to Eu3+. Therefore, the Eu3+ ion acts as the terminal of the thermal of the energy transfer processes in the Ba2TbSi4O13:Eu phosphor. Thus, we can obtain bright red luminescence in the Ba2TbSi4O13:Eu phosphor upon near UV excitation.
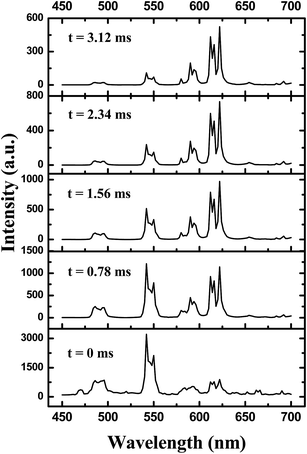 |
| Fig. 9 The time-resolved spectra of Ba2Tb1.995Si4O13:0.005Eu phosphor under 377 nm excitation. | |
Fig. 10 illustrates the energy level model for the energy transfer processes of the type Eu2+–Tb3+–Eu3+ in the Ba2Tb2Si4O13 host. As can be seen from Fig. 10, the excitation features of Ba2Tb2Si4O13:Eu phosphors in the n-UV region come from the combined absorption of Tb3+, Eu3+, and Eu2+, so that a broad and strong absorption band can be observed in the PLE spectrum (Fig. 3(b)). The energy absorbed by Eu2+ can also be efficiently transferred from the 4f65d1 configuration to the excited levels of Eu3+ and Tb3+; accordingly, the energy absorbed by Tb3+ can be transferred from the 5D4 level of Tb3+ to the 5D0 (5D1) level of Eu3+.25,26
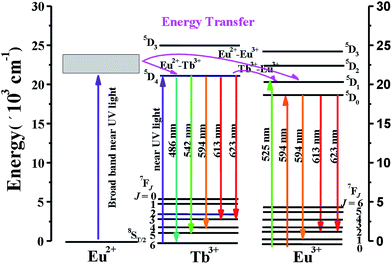 |
| Fig. 10 Energy level diagram for the ET processes among Eu2+, Tb3+, and Eu3+ in Ba2Tb2Si4O13 phosphor. | |
The thermal stability of phosphor is one of the important parameters for possible applications. For this reason, the emission spectra of the selected Ba2Tb1.97Si4O13:0.03Eu phosphor at various temperatures are shown in Fig. 11(a). The variations of the relative emission intensities as a function of temperature are plotted in Fig. 11(b). When the temperature is increased to 150 °C, the PL intensities of Tb3+ and Eu3+ ions decrease to 94.9% and 88.2% of the corresponding initial value (25 °C). In Fig. 11(b), we have also given the temperature dependent PL intensities variation of the commercial BaMgAl10O19:Eu2+ (BAM) blue phosphor, and the comparable thermal stability can be found. The small intensity decrease indicates that Ba2Tb2Si4O13:Eu could be used for high-power LED application. In general, the thermal quenching of emission intensity can be explained by several mechanisms. A widely accepted mechanism is the electronic transition through the intersection between the ground and excited states. In other words this mechanism is described as a large displacement between the potential energy curves of the ground and excited state in the configuration coordinate diagram.27 In the present case, this mechanism would be valid in the case of excitation in the absorption profile of Eu2+. To better understand the thermal quenching phenomena, the activation energy for the thermal quenching was fitted using the equation describing the crossover between Franck Condon displaced potential energy curves:28,29
|
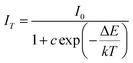 | (6) |
where
I0 is the initial PL intensity of the phosphor at room temperature,
IT is the PL intensity at different temperatures,
c is a constant, Δ
E is the activation energy for thermal quenching, and
k is Boltzmann constant (8.62 × 10
−5 eV). According to the equation, the activation energy Δ
E can be calculated from a plotting of ln[(
I0/
I) − 1] against 1/
kT, where a straight slope equals −Δ
E. As shown in
Fig. 11(b), Δ
E were found to be 0.351 and 0.296 eV for Tb
3+ and Eu
3+, respectively. The relatively high activation energy results in a good thermal stability for this phosphor. The quantum efficiency of the phosphor is another important parameter to be considered for practical application, and we have also measured the internal quantum efficiency (QE) of Ba
2Tb
2−xSi
4O
13:
xEu (
x = 0, 0.005, 0.03 and 0.09) phosphors. The measured internal QE values are 30.06%, 31.66%, 39.35% and 23.39%, respectively, for
x = 0, 0.005, 0.03, 0.09 under 377 nm excitation.
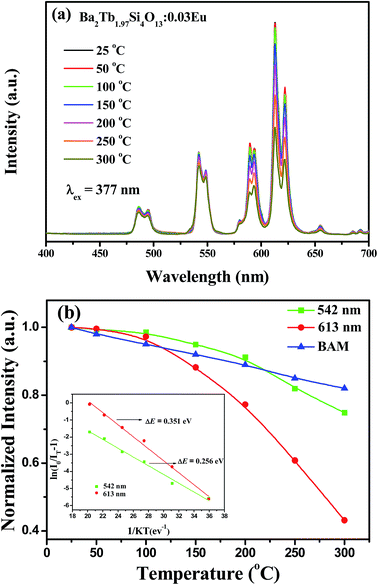 |
| Fig. 11 (a) Temperature-dependent PL spectra of Ba2Tb1.97Si4O13:0.03Eu. (b) The variations of the relative emission intensities as a function of temperature of the Ba2Tb1.97Si4O13:0.03Eu phosphor and commercial BAM phosphor. The inset shows relative PL intensities of Tb3+, and Eu3+ in Ba2Tb2Si4O13 host with raised temperatures. The inset shows the activation energy (ΔE) of Tb3+ and Eu3+ in Ba2Tb1.97Si4O13:0.03Eu phosphor. | |
4. Conclusion
In summary, color-tunable Ba2Gd2−xTbxSi4O13:yEu solid-solution phosphors were prepared by the high-temperature solid–state reaction in reducing atmosphere. The terbium ions in the host can not only form an iso-structural phase originated from the Ba2Gd2Si4O13, but they can be also used to realize the Eu2+–(Tb3+)n–Eu3+ energy transfer channel between broad-band absorption of Eu2+ and the narrow-line emission of Eu3+. On the basis of this process, the emission color of the obtained phosphors can be varied from green to yellow and finally to red by adjusting the concentration of Eu. Moreover, the temperature dependence of the luminescence intensity shows that this phosphor has an excellent thermal stability against temperature quenching.
Acknowledgements
The present work was supported by the National Natural Science Foundations of China (Grant No. No.51572023, No.51272242), Natural Science Foundations of Beijing (2132050), the Program for New Century Excellent Talents in University of Ministry of Education of China (NCET-12-0950), Beijing Nova Program (Z131103000413047), Beijing Youth Excellent Talent Program (YETP0635), the Funds of the State Key Laboratory of New Ceramics and Fine Processing, Tsinghua University (KF201306), and Fundamental Research Funds for the Central Universities (FRF-TP-15-005A2).
References
- J. C. Bünzli, Nat. Chem., 2010, 2, 698 CrossRef PubMed
. - G. G. Zhang, J. Wang, Y. Chen and Q. Su, Opt. Lett., 2010, 35, 2382 CrossRef CAS PubMed
. - G. J. Gao, S. Reibstein, M. Y. Peng and L. Wondraczek, J. Mater. Chem., 2011, 21, 3156 RSC
. - M. Y. Peng, Z. W. Pei, G. Y. Hong and Q. Su, J. Mater. Chem., 2003, 13, 1202 RSC
. - K. H. Kwon, W. B. Im, H. S. Jang, H. S. Yoo and D. Y. Jeon, Inorg. Chem., 2009, 48, 11525 CrossRef CAS PubMed
. - A. A. Setlur, Electrochem. Solid-State Lett., 2012, 15, J25 CrossRef CAS
. - D. W. Wen, J. X. Shi, M. M. Wu and Q. Su, ACS Appl. Mater. Interfaces, 2014, 6, 10792 CAS
. - Z. G. Xia, J. Q. Zhuang, A. Meijerink and X. P. Jing, Dalton Trans., 2013, 42, 6327 RSC
. - M. Wierzbicka-Wieczorek, U. Kolitsch and E. Tillmanns, Acta Crystallogr., Sect. C: Cryst. Struct. Commun., 2010, 66, 129 Search PubMed
. - H. Guo, H. Zhang, J. J. Li and F. Li, Opt. Express, 2010, 18, 27257 CrossRef CAS PubMed
. - H. Guo, H. Zhang, R. F. Wei, M. D. Zheng and L. H. Zhang, Opt. Express, 2011, 19, A201 CrossRef CAS PubMed
. - F. Zhang, Y. Wang and Y. Tao, Mater. Res. Bull., 2013, 48, 1952 CrossRef CAS
. - H. P. Zhou, Q. P. Wang, M. S. Jiang, X. X. Jiang and Y. Jin, Dalton Trans., 2015, 44, 13962 RSC
. - E. Zych, J. Trojan-Piegza, D. Hreniak and W. Strek, J. Appl. Phys., 2003, 94, 1318 CrossRef CAS
. - P. L. Li, Z. J. Wang, Z. P. Yang and Q. L. Guo, Opt. Commun., 2014, 332, 83 CrossRef CAS
. - P. I. Paulose, G. Jose, V. Thomas, N. V. Unnikrishnan and M. K. R. Warrier, J. Phys. Chem. Solids, 2003, 64, 841 CrossRef CAS
. - J. Yang, C. M. Zhang, C. X. Li and J. Lin, Inorg. Chem., 2008, 47, 7262 CrossRef CAS PubMed
. - F. H. Le, L. X. Wang, W. Jia, D. Z. Jia and S. J. Bao, J. Alloys Compd., 2012, 512, 323 CrossRef CAS
. - T. Li, P. L. Li, Z. J. Wang, S. C. Xu, Q. Y. Bai and Z. P. Yang, Dalton Trans., 2015, 44, 16840 RSC
. - J. Wang, S. B. Wang and Q. Su, J. Solid State Chem., 2004, 177, 895 CrossRef CAS
. - Z. G. Xia, Y. Y. Zhang, M. S. Molokeev and V. V. Atuchin, J. Phys. Chem. C, 2013, 117, 20847 CAS
. - W. B. Im, N. N. Fellows, S. P. DenBaars, R. Seshadri and Y. Kim, Chem. Mater., 2009, 21, 2957 CrossRef CAS
. - Y. Zhang, X. J. Li, K. Li, H. Z. Lian, M. M. Shang and J. Lin, ACS Appl. Mater. Interfaces, 2015, 7, 2715 CAS
. - Z. G. Xia and R. S. Liu, J. Phys. Chem. C, 2012, 116, 15604 CAS
. - M. Bettinelli and C. D. Flint, J. Phys.: Condens. Matter, 1990, 2, 8417 CrossRef CAS
. - S. C. Xu, P. L. Li, Z. J. Wang, T. Li, Q. Y. Bai, J. Sun and Z. P. Yang, J. Mater. Chem. C, 2015, 3, 9112 RSC
. - H. D. Luo, J. Liu, X. Zheng, L. X. Han, K. X. Ren and X. B. Yu, J. Mater. Chem., 2012, 22, 15887 RSC
. - J. Zhou and Z. G. Xia, Sci. Rep., 2015, 3, 12149 CrossRef PubMed
. - W. H. Fonger and C. W. Struck, J. Chem. Phys., 1970, 52, 6364 CrossRef CAS
.
|
This journal is © The Royal Society of Chemistry 2016 |