DOI:
10.1039/C5RA21489K
(Paper)
RSC Adv., 2016,
6, 9387-9395
Nanostructured conducting molecularly imprinted polypyrrole based quartz crystal microbalance sensor for naproxen determination and its electrochemical impedance study†
Received
15th October 2015
, Accepted 6th January 2016
First published on 11th January 2016
Abstract
This paper represents a naproxen (NAP) imprinted nanostructured conducting molecularly imprinted polypyrrole (CMIP) film which is electrochemically synthesized on a quartz crystal microbalance (QCM) gold electrode and electrochemically overoxidized for increasing its selectivity as a recognition element of a QCM sensor for the detection of NAP. It was shown that the selectivity of the obtained film toward NAP was enhanced by controlled overoxidation. The characteristics of CMIP and overoxidized (OCMIP) films were investigated by ATR FT-IR, cyclic voltammetry and electrochemical impedance spectroscopy (EIS), and the morphology and coverage of the film were studied by FE-SEM. Parameters of cyclic voltammetric preparation of the CMIP film including the potential range, scan rate, number of cycles (film thickness) and the effect of surfactant on polymer film growth were optimized. The overoxidation condition including overoxidation potential, number of cycles and pH and type of electrolyte solution were studied and optimized. It was found that the excessive overoxidation of CMIP film would cause two unwanted effects on the sensor; first the loss of sensor regeneration in repeated uses, and second, the decrease of the cohesion of film on the gold electrode. Thus the amount of overoxidation of the film was controlled very carefully to obtain the best selectivity with minor loss of film cohesion and sensor reproducibility. This aim was satisfied by the aid of impedimetric study of the film before and during the overoxidation. Results obtained from the QCM and impedance measurements show that overoxidized NAP imprinted CMIP could be used for determination of NAP in aqueous media with a limit of detection of 0.04 μmol L−1 and RSD = 4.9% (n = 5). The proposed sensor offers a good selectivity and cost effective properties for fast determination of NAP with ease of preparation and simple instrumentation.
Introduction
Application of conducting molecularly imprinted polymers (CMIP), particularly polypyrrole, is of great interest in chemical sensor design.1 Many reports of the successful application of imprinted polypyrroles for various analytes are reported in the literature.2–6 A key advantage of CMIPs is the possibility of the application of electrical potential in these polymers for the enhancement of sensing processes, especially in template removal.7 The electrostatic removal of the anionic templates distinctively features the overoxidized CMIP recognition element, in that the quantitative removal of the template takes place nearly straightaway upon application of a positive potential or addition of a suitable oxidizing agent. This is in contrast to the typical non-conducting molecular imprinting procedures in which the removal of the template is often time consuming, going from many hours to days.8
Overoxidation has two effects on selectivity of CMIP; first it can eliminate the template molecule to create the corresponding cavity, and second, it can cure the polymer texture to make the cavity stable.8 On the other hand, the backbone of the polymer loses its positive sites due to the overoxidation, and the potential induced uptake of negatively charged anions becomes limited to more bulky anions with delocalized negative charge. This could create a selectivity toward NAP as a bulky anion in comparison to small charged anionic interfering molecules like ibuprofen. Such capability is explained by the size-discriminating properties of OCMIP films.8 Overoxidation of CMIP proceeds upon application of more positive potentials.9 The overoxidation onset potential is highly dependent on pH of the supporting electrolyte so that in the higher pH values, the overoxidation occurs in the less positive potentials which is coincident with the electrolysis reaction of water to give out oxygen. It was supposed that the oxygen produced in the electrolysis of water results in the overoxidation of the CMIP films.10 Moreover the nucleophilic attack of hydroxide ion to polypyrrole upon overoxidation, destroys its conjugated structure by the insertion of carbonyl groups on the pyrrole rings.11 Detailed discussions of the overoxidation mechanisms can be found elsewhere.10 At the same time, overoxidation is prone to cause exfoliation of the film off the electrode surface especially for polished metallic electrodes.8,9 However this difficulty can be circumvented by roughening the surface of the metallic electrode,8,9 or by controlling the amount of overoxidation process. The former solution could not be applicable to QCM electrode, so the controlled overoxidation is the distinctive way to exploit the advantages of CMIP overoxidation without facing problems evolved from exfoliation of overoxidized CMIP film off the electrode surface. In addition to the afore-mentioned problems of OCMIP film, another limitation could arise from the highly overoxidized film due to the diminished charge transfer capability of film and irreversibility of exchange process and loss of potential induced uptake or release of analyte molecules, which causes the loss of sensor sensitivity, especially for the anionic NAP.3,11,12 This problem crucially urges the overoxidation to be implemented in a controlled manner.
EIS represents a powerful method for probing the interfacial reaction mechanisms of modified electrodes, providing a rapid approach for monitoring the dynamics of interaction for the evaluation of chemical transformations and processes associated with conductivity changes in an electrochemical circuit including CMIP in sensing applications,1,3,12–16 impedimetric sensing1,5 and biosensing applications.17
NAP [(+)-(S)-2-(6-methoxynaphthalen-2-yl)propionic acid], a widely used nonsteroidal anti-inflammatory drug for mild to moderate pain relief, is a typical bulky anionic drug which could be used as an analyte choice of this study. The aim of this study is the preparation of NAP imprinted nanostructured CMIP film which is electrochemically synthesized on a QCM gold electrode and electrochemically overoxidized for increasing its selectivity as recognition element of QCM sensor for detection of NAP.
Materials and methods
Chemicals and reagents
Pyrrole (Merck, Germany) was purified by distillation and stored in fridge prior to use. NAP, ibuprofen and mefenamic acid were obtained from Sigma-Aldrich and used as received. Sodium perchlorate, sodium chloride, sodium phosphate and sodium dodecylbenzenesulfonate (SDBS) were purchased from Merck and used without further purification. Stock solutions of drugs were prepared in methanol (1000 mg mL−1). Standard solutions containing the required amount of each analyte were freshly prepared daily by diluting stock solutions with doubly distilled water. All solvents and buffer solutions were of HPLC grade.
Apparatus
A 10 MHz quartz crystal microbalance system with homemade oscillator electronic circuitry used for detection and quantification of NAP. The quartz crystals used were commercially available 10 MHz, AT-cut type (diameter, 13.67 mm), with polished gold electrodes (diameter, 5.1 mm) on both sides (ICM Co. USA). The oscillation frequency of QCM was followed by a universal frequency counter model 8030 (Dagatron, South Korea) and the signal was acquired and recorded by computer. Electrochemical polymerization and overoxidation of pyrrole and all electrochemical experiments including the impedance measurements were performed by AUTOLAB model PGSTAT 302 (Metrohm, Netherlands) potentiostat and the QCM sensor as working electrode, a Pt counter electrode and an Ag/AgCl reference electrode in a homemade Teflon electrochemical cell such that just one side of QCM gold electrode was in contact with liquid. The EIS equivalent circuit fittings were performed by Nova 1.8 software (Metrohm Autolab B.V.). A pH/ion-meter Metrohm 713 (Metrohm, Switzerland) with a standard uncertainty of 0.1 mV was used for all pH measurements at 25 ± 0.1 °C. The field emission scanning electron micrographs (FE-SEM) of the modified electrode surface were obtained using model SIGMA VPZEISS instrument. The ATR FT-IR spectra of CMIP and OCMIP films were recorded by Bruker model Vertex 80 ATR FT-IR spectrometer.
Results and discussion
Preparation of CMIP film
NAP imprinted CMIP film was synthesized by cyclic voltammetry method on QCM gold electrode in a three electrode arrangement. Prior to the film synthesis, the working gold electrode was treated with fresh piranha solution (98% H2SO4
:
30% H2O2 = 3
:
1, v/v) for 5 minutes, rinsed copiously with doubly distilled water and dried under nitrogen flow. Coating solution including 2 mL of the 0.1 mol L−1 pyrrole aqueous solution and 0.005 mol L−1 of NAP (as template anion) with pH 6 (adjusted with 0.01 mol L−1 NaOH), was deoxygenated with nitrogen flow and placed in the cell. The CMIP film was obtained in the potential range between −0.1 and +0.8 V versus Ag/AgCl reference electrode with scan rate of 50 mV s−1. Fig. 1 shows a typical cyclic voltammogram of pyrrole (0.1 mol L−1) in the above mentioned condition. The cyclic voltammogram of 0.005 mol L−1 NAP aqueous solution (pH = 6) without pyrrole is also shown in the Fig. 1 to ascertain the electro-inactivity of NAP in applied potential range. The optimum film thickness is studied by measuring the frequency shift of sensors with different film thicknesses, to a known concentration of NAP. As could be seen from the results presented in Fig. S1,† the sensor with 1 to 1.2 μg film mass, which corresponds to 200–250 synthesis cycles respectively, shows the maximum sensing response. The rate of film formation per each cycle of synthesis was found to be low in comparison with our previous work which the dopant anion was ibuprofen.7 This could be due to the lower dissociation of NAP molecules in pH of synthesis solution, and also the bulky nature of NAP molecule which hinders the insertion of its anionic form into the CMIP structure for neutralization of the polymer positive charges which is crucial for progress of film synthesis. As a result, to achieve the optimum CMIP thickness, 200 coating cycles should be implemented which takes more than 120 minutes. The best solution for performing the film synthesis at an equitable time is to increase the rate of polymerization by performing the synthesis in the presence of another anion as auxiliary dopant.
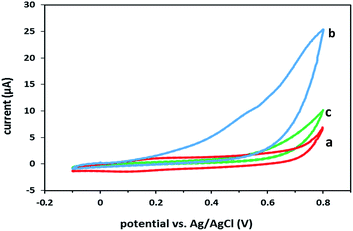 |
| Fig. 1 Typical cyclic voltammograms of 0.005 mol L−1 NAP solution (a), the solution containing 0.005 mol L−1 NAP and 0.1 mol L−1 pyrrole with in cycle 1 (b) and cycle 50 (c) at pH = 6 and scan rate of 50 mV s−1 with potential sweep range of −0.1 to +0.8 V vs. Ag/AgCl. | |
This aim was satisfied by performing the synthesis in the presence of lower amounts of SDBS which dissociate very easily in the synthesis condition, and acts as a good auxiliary anion dopant.18 Fig. 2 shows the frequency shift of the QCM vs. number of synthesis cycles for NAP containing synthesis solutions with and without added SDBS. As could be seen from the Fig. 2, the amount of frequency shift vs. number of synthesis cycles, extremely increases in solutions containing SDBS and hence the optimum film thickness could be achieved only in 20 synthesis cycles in presence of 0.0001 M SDBS and 5 cycles for 0.001 M which takes only 12 and 3 min respectively . The frequency shift of CMIP modified QCM electrode was measured and considered as criteria for reproducibility of CMIP film electrodeposition. For 5 replicate electrosynthesis of CMIP with 200 cycles, a good reproducibility of results has been obtained with RSD ≤ 2%.
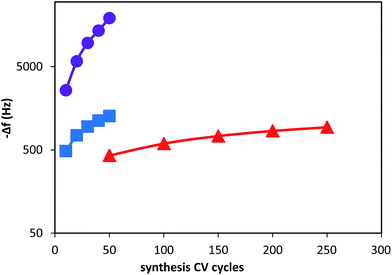 |
| Fig. 2 The frequency shift of the QCM for synthesis of CMIP film in different synthesis solutions containing 0.005 mol L−1 NAP and 0.1 mol L−1 pyrrole at pH = 6 (triangles) and the same solution with added SDBS of 0.0001 mol L−1 (squares) and 0.001 mol L−1 (circles). | |
Overoxidation of CMIP film
The overoxidation of the prepared CMIP film was performed by application of more positive potentials to the polymer film on work electrode in the various electrolyte solutions. This was for the removal of embedded NAP molecules from the CMIP and generation of cavities for NAP11 and increase of sensor selectivity. For this purpose, the CMIP film on gold QCM electrode was electrochemically treated in 0.01 mol L−1 phosphate buffer solution (pH = 7.4) with sweeping the potential from −0.1 to 1.2 V vs. Ag/AgCl by cyclic voltammetry method with scan rate of 50 mV s−1. A typical cyclic voltammogram of CMIP overoxidation is presented in the Fig. 3, shows a decrease in anodic peak current in successive scans due to the decrease of film conductivity.
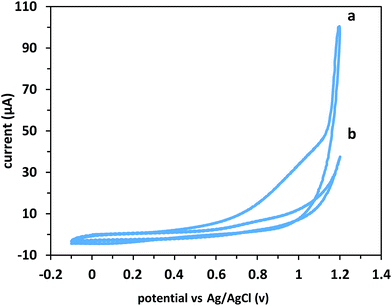 |
| Fig. 3 Typical cyclic voltammogram of CMIP film overoxidation on QCM gold electrode in 0.01 mol L−1 phosphate buffer solution (pH = 7.4) with sweeping the potential from −0.1 to 1.2 V vs. Ag/AgCl with scan rate of 50 mV s−1 for scan 1 (a) and scan 10 (b). | |
Characterization of CMIP and OCMIP films
FE-SEM study
FE-SEM micrographs of prepared CMIP layer before and after overoxidation, with 50k magnification, are shown in the Fig. 4. The very thin layer of CMIP film with a typical spherical morphology of polypyrrole with average size distribution around 30 nm, could be seen in the Fig. 4A. The uniformity and coverage of film are satisfactory according to the lower magnification FE-SEM micrographs obtained from various points of electrode area which a sample micrograph is presented in the Fig. S2.† In the Fig. 4B, the FE-SEM micrograph of the same CMIP film after 10 cycles of overoxidation is shown. After 20 overoxidation cycles, the uniformity of the overoxidized film was decreased and a series of pinholes were appeared in the polymer film due to the loss of cohesion of film on gold electrode and exfoliation of the film which is shown in Fig. S3A† in high magnification. The appearance of larger pinholes after 30 cycles of overoxidation also is shown in Fig. S3B† with lower magnification.
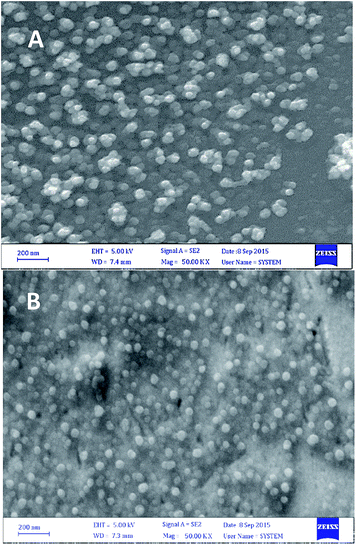 |
| Fig. 4 FE-SEM images of prepared CMIP film deposited on QCM electrode as prepared (A) and after 10 cycle of overoxidation (B). | |
ATR FT-IR spectroscopy
The ATR FT-IR spectra of CMIP film before and after 10 cycles of overoxidation is depicted in Fig. 5. The spectra of as prepared CMIP film shows the band at 1458 cm−1 corresponds to the C–N stretching vibration, the band at 3346 cm−1 corresponds to N–H stretching and a weakly resolved peak around 1560 cm−1 assigns to stretching vibration of the C
C bond of pyrrole.6,15 The peaks at 1712 and 2950 cm−1 are related to the C
O stretching and methylene C–H stretching bond of NAP molecule respectively which proves the imprinting of NAP molecules to CMIP structure.19 The FT-IR spectra of the OCMIP film, clearly confirms the removal of NAP molecule from film (C–H stretching bond of NAP in 2950 cm−1 disappeared after overoxidation) and indicates the incidence of overoxidation by appearing stronger C
O stretching bond in 1710 cm−1 and O–H stretching bond in 3350 cm−1 due to partial breakdown of polypyrrole resonance structure through the nucleophilic attack of hydroxyl groups and formation of carbonyl and C–O bonds.16,19,20
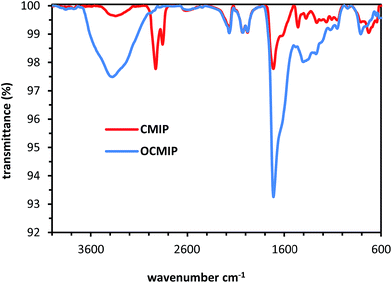 |
| Fig. 5 The ATR FT-IR spectra of CMIP film as prepared (CMIP) and after 10 cycles of overoxidation (OCMIP). | |
EIS study of CMIP film
AC impedance spectra of the CMIP film in different steps including freshly prepared CMIP film, overoxidized film and overoxidized film after NAP uptake and release were collected in the same frequency regions of 100 Hz to 100 kHz with a single sinusoidal wave type of 10 mV amplitude containing the same number of frequency data points. The electrode potential of 0.0 V vs. Ag/AgCl reference electrode corresponding to the reversible charge transfer across the polymer film was employed during the EIS measurements.21 The Nyquist plots for the CMIP film as prepared (CMIP), after 10 (OCMIP1), 20 (OCMIP2) and 30 (OCMIP3) cycles of overoxidation and after potential induced uptake and release of NAP for overoxidized film at +0.3 and −0.3 V vs. Ag/AgCl respectively, are compared in Fig. 6. All of the Nyquist plots are composed of the weakly-resolved compressed semi arc at high frequency regions.1,14 The left-side intercept of this semicircle will represent the numerical value for solution resistance (Rs) which was measured around 70 Ω, and the right-side intercept of the corresponding charge transfer resistance (Rct) which can be seen more clearly in the Fig. 6B.5 The diameter and the symmetry of this arc are controlled by the double-layer capacitance (Cdl). The semicircle (defined by the parallel connected Rct and Cdl) is connected with a straight line of ∼45° slope at intermediate frequencies.22 This represents the diffusion process (Warburg type resistor) which followed by a capacitive region of vertical line at the end of EIS spectra (the lowest frequencies) which is related to the ion accumulation (building the capacitor) at the electrolyte/electrode interface and inside the polymer.13 Remarkably, no parts of the spectra in this range resembles the pure Warburg impedance behaviour, which means fast ionic diffusion and/or special diffusion paths similar to that observed for a crystalline oxide material. Changes in the impedance magnitude follow that supported by the ATR/FT-IR spectra thus resulting mainly from differences in the conductivity of CMIP film before and after overoxidation and doped form. Generally, the real component of the impedance increases with amount of overoxidation as a consequence of a decreased conductivity and charge transfer of polymer film.14 On the other hand the imaginary component of the impedance decreases with occurrence of overoxidation as a consequence of an increased capacitance of the overoxidized film.21 It should be noted that the overall pattern of the Nyquist plots remained constant regardless of the polymer structure change in overoxidation steps. It is evident from these data that the resistance of the CMIP film is low at the studied potential, but increases as the number of overoxidation increases from 0 to 20 cycles before increasing sharply at 30 cycles. The Bode plot (|Z| versus log
f) of impedance data for CMIP which is presented in Fig. S4,† shows the same trend and increase in the resistance of CMIP film due to the overoxidation.
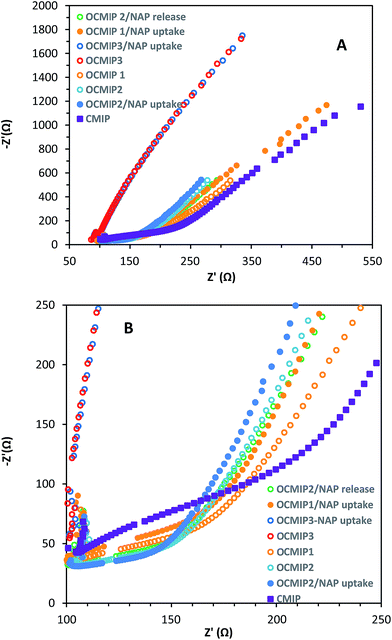 |
| Fig. 6 The Nyquist plots for the CMIP film as prepared (CMIP), after 10 (OCMIP1), 20 (OCMIP2) and 30 (OCMIP3) cycles of overoxidation and after potential induced uptake and release of NAP for overoxidized film at +0.3 and −0.3 V vs. Ag/AgCl respectively measured at 0.0 V vs. Ag/AgCl in 50 mM phosphate buffer (pH = 7.4) (A) and the amplified high frequency region (B). | |
These data were fit to the equivalent circuit presented in Fig. S5,† that represents the Au electrode/CMIP/electrolyte interfaces and is used for the fitting of impedance spectra which consists of an uncompensated solution resistance (RS = 70 Ω cm2) at the high frequency intercept. The circuit element RHF represents resistance at high frequency, or charge-transfer resistance (Rct), which contains contributions from the electronic and ionic resistance, as shown in eqn (1).
Here, Re is the electronic resistance and Ri is the ionic resistance. CPEHF and CPELF elements are constant phase elements associated with high frequency impedance and low frequency impedance, respectively. Constant phase elements were used to determine the capacitance and were used rather than capacitors to take into account the inhomogeneity of the surface of the electrode.3,12
The CPEHF represents the high frequency capacitance, or double layer capacitance, in parallel with RHF. At overoxidized states the value for RHF is dominated by Ri. Based on the well-known high electronic conductivity of CMIP when it is even slightly oxidized, it can be assumed that the electronic resistance is negligible, i.e., (Ri ≫ Re) and CPELF represents the low frequency capacitance.17
The charge transfer resistance Rct is the parameter of interest as it provides information about the stability of the overoxidized polymer material and charge transfer phenomenon through the CMIP film which is responsible for QCM sensor signal.12,13 The parameters of the best fits of the experiment – simulation data are collected in Table 1.
Table 1 Impedance values of parameters for the circuit elements evaluated by fitting the impedance data of CMIP film in different conditions to the equivalent circuit shown in Fig. S5
Element/film type |
CMIP |
OCMIP1 |
OCMIP2 |
OCMIP2/Nap |
OCMIP3 |
Rs (Ω) |
70.11 ± 0.25 |
73.83 ± 0.54 |
71.05 ± 0.33 |
72.12 ± 0.36 |
68.23 ± 0.21 |
CPE-THF (mF) |
61.72 ± 0.78 |
69.62 ± 0.85 |
74.30 ± 1.02 |
71.52 ± 0.42 |
94.58 ± 2.14 |
CPE-PHF (mF) |
0.68 ± 0.18 |
0.73 ± 0.07 |
0.76 ± 0.02 |
0.7 ± 0.03 |
0.84 ± 0.06 |
RHF (Ω) |
85.14 ± 2.57 |
120.35 ± 5.08 |
150.41 ± 4.28 |
141.01 ± 3.07 |
254.27 ± 8.51 |
CPE-TLF (mF) |
132.05 ± 3.45 |
108.24 ± 2.31 |
96.08 ± 5.17 |
99.83 ± 2.36 |
47.41 ± 3.12 |
CPE-PLF (mF) |
0.94 ± 0.03 |
0.91 ± 0.02 |
0.83 ± 0.02 |
0.88 ± 0.01 |
0.68 ± 0.05 |
Moreover, to investigate the sensing mechanism of proposed sensor, which is supposed to be based on potential induced uptake of the NAP, cyclic voltammograms of the hexacyanoferrate probe, are recorded on the bare gold electrode, and the electrode modified with as prepared CMIP and OCMIP with optimum overoxidation, and the results are depicted in Fig. S6.† As could be seen from the figure, the anodic and cathodic currents are decreased with overoxidation, but after the 10 cycles of overoxidation, almost 50% of the film conductivity is maintained compared to the CMIP film according to the amount of passed charge. This is in accordance with the EIS data obtained for resistance of film.
Optimization of CMIP/QCM sensor conditions for NAP
Selectivity enhancement and sensor reusability
As described in the film synthesis section, three different CMIP films were prepared with different amounts of SDBS as auxiliary dopant anion. According to data presented in Table 2, the CMIP film without the SDBS represents better results for selective detection of NAP, however it takes more than 2 h to perform the film synthesis. This could be due to the formation of more NAP template cavities in CMIP prepared without SDBS in comparison with the films which were synthesized in the presence of SDBS as auxiliary dopant.8
Table 2 Effect of SDBS auxiliary dopant on CMIP film selectivity for NAP
[SDBS] (mmol L−1) |
NAP |
Ibuprofen |
Required synthesis time (min) |
−Δf (Hz) |
Selectivitya (%) |
−Δf (Hz) |
Selectivity (%) |
Selectivity (%) = (−Δfspecies/−ΔfNAP) × 100. |
0 |
35 ± 1 |
100 |
9 ± 1 |
25.7 |
120 |
0.1 |
33 ± 2 |
100 |
12 ± 3 |
36.4 |
20 |
1 |
30 ± 4 |
100 |
16 ± 5 |
53.3 |
5 |
The overall process of sensor preparation and sensing is depicted in Scheme 1. The amount of overoxidation is controlled to obtain the maximum sensing capability (especially the selectivity) with minimum loss of film cohesion and sensor reusability. For this purpose the response of sensors with different degree of overoxidation by different overoxidation CV cycles for same concentrations of aqueous solution of analyte (NAP) and interfering molecules with similar structure (ibuprofen and mefenamic acid) were studied. The results which are shown in Fig. 7A indicate that the overoxidation of film increases the selectivity of sensor toward NAP up to 10 overoxidation cycles. On the other hand the reusability of the sensor in successive determinations decreases by increment of overoxidation degree (Fig. 7B). Considering this dilemma, the optimum amount of overoxidation achieved by 10 CV cycles.
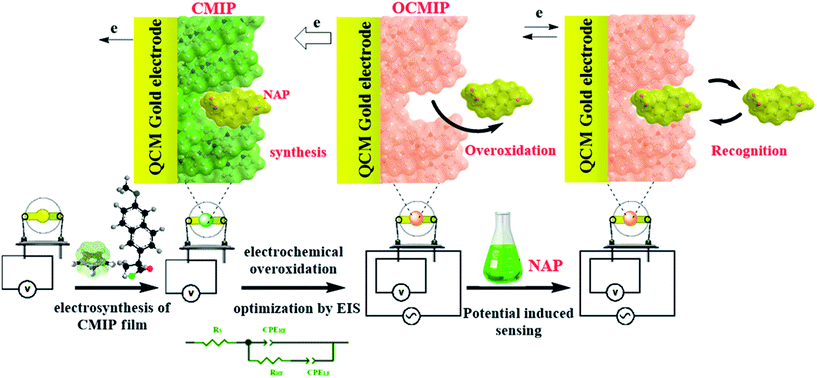 |
| Scheme 1 The overall process of preparation and sensing mechanism of proposed sensor. | |
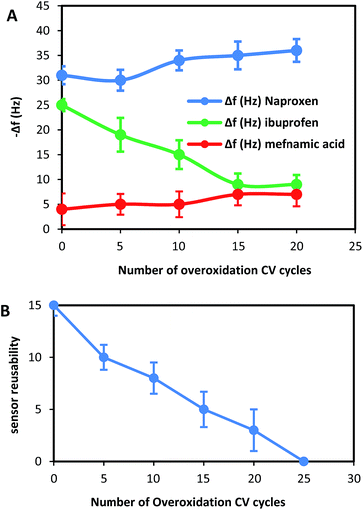 |
| Fig. 7 The response of sensor with different degree of overoxidation by different overoxidation CV cycles for same concentrations of aqueous solution of analyte (NAP) 5 μM and interfering molecules with similar structure (ibuprofen 10 μM and mefenamic acid 10 μM) (A). The reusability of the sensor in successive determinations for different degrees of overoxidation (B). | |
pH effect
According to the overoxidation mechanism of CMIP film from literature, the overoxidation of CMIP depends on the pH of electrolyte solution. In acidic pH the overoxidation process starts in more positive potential with a controlled rate while in alkaline solutions the overoxidation commences in less positive potentials with fast rates. The latter one is not favoured due to damage to the QCM electrode material in more positive potentials. On the other hand the control of overoxidation rate is crucial for optimum overoxidation degree for better selectivity and reusability of presented sensor. So the overoxidation in a mild basic pH and potential could be a better choice. Fig. S7† shows the overoxidation peaks of CMIP film in different electrolyte solutions including the perchlorate (pH = 6), phosphate (pH = 7.4) and NaOH (pH = 11). As could be seen from the figure, the overoxidation peak occurs in more positive potentials with decrease of pH values. So the phosphate buffer with pH = 7.4 is used for the rest of overoxidation experiments.
Uptake and release potentials
The optimum potentials for uptake and release of NAP by overoxidized CMIP film were studied by applying different positive and negative potentials respectively to the QCM electrode in uptake and release solutions, and the results are depicted in the Fig. S8.† Fig. S8A† shows the frequency shift of the sensor due to the loading of the drug into the CMIP film at different positive uptake potentials. The positive charge sites of partially oxidized CMIP are responsible for accumulation of NAP anions during the application of positive potentials in the uptake step in which the NAP anions are inserted into CMIP and neutralize its positive charge. As could be seen in the Fig. S8A,† the frequency shift and thus the amount of drug loaded into the sensor, increases with the potential increment up to +0.3 and the amount of drug absorption reaches a plateau. Therefore, the optimal absorption potential of +0.3 V was used in subsequent studies. To determine the optimal potential for release of NAP from CMIP film, the NAP loaded CMIP was exposed to 2 mL of 0.01 mol L−1 NaClO4 solution and negative potentials versus Ag/AgCl applied for 600 s and results are depicted in Fig. S8B.† The amount of IBP release increases, as the employed potential is made more negative up to −0.3 V vs. Ag/AgCl, the amount of released drug reaches a plateau. The release of NAP is accomplished through exchanging with perchlorate ions which are presented in the solution. The optimal uptake and release potentials were found at +0.3 and −0.3 V vs. Ag/AgCl respectively. These results, proves the significant role of potential in controlling the uptake/release of NAP.
Uptake and release times
The uptake and release time is equivalent to the time needed to reach equilibrium at the film/solution interface for a given CMIP film for extraction and desorption steps. The uptake and release time profiles of NAP at OCMIP film were obtained under both open circuit and controlled potential conditions (Fig. 8). From the data depicted in the Fig. 8, the optimal times used for uptake and release were 400 and 300 s, respectively. As shown in this Fig. 8, the uptake and release rates of NAP were increased under controlled potential conditions. The IBP uptake at open circuit was very negligible, even for times longer than 20 min.
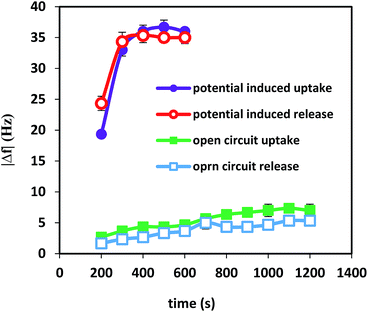 |
| Fig. 8 Effect of uptake ( ) and release ( ) time by applying potential and under open circuit conditions for uptake ( ), and release ( ); uptake solution, 5 μmol L−1 NAP, Euptake = 0.3 V; release solution, 0.01 M ClO4−, Erelease = −0.3 V vs. Ag/AgCl. | |
It is evident from the results that the response time of the proposed potential induced OCMIP/QCM sensor was enhanced in the ordination of 10 folds compared to previously reported on non-conducting MIP based sensors (without applying potential). This achievement if fulfilled by the controlled overoxidation of CMIP with partial maintaining of OCMIP film conductivity which enables application of potential for uptake and release of anionic analyte while achieving a satisfactory level of selectivity and reusability.
Analytical performance of QCM/OCMIP sensor
To obtain the response of the OCMIP/QCM sensor to different concentrations of the NAP, the frequency shift of the sensor plotted versus the concentration of NAP and the calibration curve was established and depicted in Fig. 9. As shown in the figure, it was observed that the frequency shift of the QCM/OCMIP was increased with a linear dependency by the increase of concentration of NAP in the range of 0.1–15 μmol L−1 and a hyperbolic curve was obtained for the rest of studied NAP concentration range 15–50 μmol L−1. The sensitivity was calculated as the slope of linear part of calibration curve and found as 4.9 Hz μmol−1 L. The detection limit, defined as the concentration of analyte giving frequency shift equivalent to three standard deviation of the blank plus the net blank frequency shift, was 0.04 μmol L−1. The experiments were performed in three replicates and the samples were analysed in replicates of three as well. The reproducibility of measurements was tested with five samples and a satisfying result (RSD = 4.9%, n = 5) was obtained.
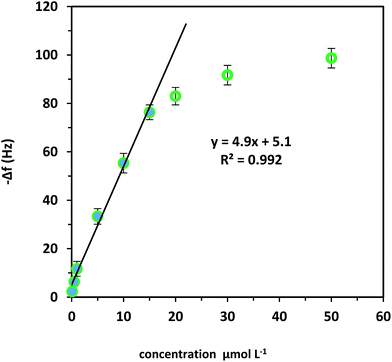 |
| Fig. 9 The response of the OCMIP/QCM sensor to different concentrations of the NAP. | |
To the best of our knowledge from our literature survey, this is the first report of QCM sensor for NAP. However various NAP sensors based on electrochemical and optical transduction mechanism are reported previously. The analytical figures of merit for proposed QCM/OCMIP sensor for NAP was compared with values obtained using different sensors previously reported in the literature (Table 3). Comparing the QCM/OCMIP sensor results for determination of naproxen with literature data using different transduction and recognition mechanisms, shows that the proposed sensor is a simple sensor with 10 fold better detection limit and comparable analysis time and accuracy.
Table 3 Comparison of the limit of detection and the relative standard deviation for analysis of NAP by OCMIP based QCM sensor with different sensors previously reported in the literature
Transduction method |
Recognition element |
LOD (μmol L−1) |
RSD% |
Analysis time (min) |
Matrix |
Ref. |
Amperometry |
Cytochrome p450 |
16 |
6.25 |
— |
Water |
23 |
Cyclic voltammetry |
Cytochrome p450 |
33 |
54 |
— |
Water |
23 |
Fluorescence optode |
C18 silica |
69 |
2 |
7.5 |
Urine, serum |
24 |
Potentiometry |
Graphite/Hg naproxonate |
39 |
— |
0.5 |
Water |
25 |
Cyclic voltammetry |
BSA/reduces graphene oxide |
0.33 |
4.8 |
— |
Water |
26 |
QCM |
OCMIP |
0.04 |
4.9 |
5 |
Water |
This work |
Conclusions
The electrochemical behaviour of electrosynthesized CMIP films before and after electrochemical overoxidation has been studied by EIS and the application of overoxidized CMIP film as recognition element of QCM sensor for analysis of NAP in aqueous solutions was studied. The impedance results analysed in terms of the charge transfer resistance to control the degree of overoxidation which is crucial in achieving the higher selectivity in one hand and maintaining the cohesion of the overoxidized film on sensor electrode and sensor reusability in the other. The results of impedance data were fit to a model circuit with elements and the change in the charge transfer resistance of CMIP film due to overoxidation was in agreement with the ATR FT-IR spectra and FE-SEM graphs. The prepared sensor was successfully used for detection of NAP aqueous solutions in 0.1 μmol L−1 concentration. The selectivity and sensitivity of proposed sensor for NAP was superior to the previously reported sensors.
Acknowledgements
Financial support from the Research Council of Tarbiat Modares University and Iran National Science Foundation (INSF) is gratefully acknowledged.
References
- V. Ratautaite, S. D. Janssens, K. Haenen, M. Nesládek, A. Ramanaviciene, I. Baleviciute and A. Ramanavicius, Electrochim. Acta, 2014, 130, 361–367 CrossRef CAS.
- A. Gültekin, G. Karanfil, M. Kuş, S. Sönmezoğlu and R. Say, Talanta, 2014, 119, 533–537 CrossRef PubMed.
- V. Ratautaite, D. Plausinaitis, I. Baleviciute, L. Mikoliunaite, A. Ramanaviciene and A. Ramanavicius, Sens. Actuators, B, 2015, 212, 63–71 CrossRef CAS.
- J. M. Kim, J. C. Yang and J. Y. Park, Sens. Actuators, B, 2015, 206, 50–55 CrossRef CAS.
- Z. O. Uygun and Y. Dilgin, Sens. Actuators, B, 2013, 188, 78–84 CrossRef CAS.
- M. Babaei and N. Alizadeh, Sens. Actuators, B, 2013, 183, 617–626 CrossRef CAS.
- M. R. Eslami and N. Alizadeh, Sens. Actuators, B, 2015, 220, 880–887 CrossRef CAS.
- S. Tokonami, H. Shiigi and T. Nagaoka, in Molecularly Imprinted Sensors, ed. S. L. Ge and S. A. P. Lunec, Elsevier, Amsterdam, 2012, pp. 73–89, DOI:10.1016/B978-0-444-56331-6.00004-9.
- V. Ratautaite, A. Ramanaviciene, Y. Oztekin, J. Voronovic, Z. Balevicius, L. Mikoliunaite and A. Ramanavicius, Colloids Surf., A, 2013, 418, 16–21 CrossRef CAS.
- Y. Li and R. Qian, Electrochim. Acta, 2000, 45, 1727–1731 CrossRef CAS.
- C. Debiemme-Chouvy and T. T. M. Tran, Electrochem. Commun., 2008, 10, 947–950 CrossRef CAS.
- S. Saha, P. Sarkar and A. P. F. Turner, Electroanalysis, 2015, 26, 2197–2206 CrossRef.
- S. M. Ebrahim, M. M. A.-E. Latif, A. M. Gad and M. M. Soliman, Thin Solid Films, 2010, 518, 4100–4105 CrossRef CAS.
- H. L. K. S. Mosch, S. Hoppener, R. M. Paulus, B. Schroter, U. S. Schubert and A. Ignaszak, Phys. Chem. Chem. Phys., 2015, 17, 13323–13332 RSC.
- S. T. Navale, G. D. Khuspe, M. A. Chougule and V. B. Patil, Org. Electron., 2014, 15, 2159–2167 CrossRef CAS.
- C. Cui, G. Wu, H. Yang, S. She, J. Shen, B. Zhou and Z. Zhang, Solid State Commun., 2010, 150, 1807–1811 CrossRef CAS.
- A. Ramanavicius, A. Finkelsteinas, H. Cesiulis and A. Ramanaviciene, Bioelectrochemistry, 2010, 79, 11–16 CrossRef CAS PubMed.
- I. L. Lehr and S. B. Saidman, React. Funct. Polym., 2008, 68, 1152–1158 CrossRef CAS.
- M. Ahmadi, T. Madrakian and A. Afkhami, Sens. Actuators, B, 2015, 210, 439–445 CrossRef CAS.
- I. Rodríguez, B. R. Scharifker and J. Mostany, J. Electroanal. Chem., 2000, 491, 117–125 CrossRef.
- J. Mostany and B. R. Scharifker, Synth. Met., 1997, 87, 179–185 CrossRef CAS.
- M. Grzeszczuk and R. Ozsakarya, RSC Adv., 2014, 4, 22214–22223 RSC.
- C. Baj-Rossi, T. Rezzonico Jost, A. Cavallini, F. Grassi, G. De Micheli and S. Carrara, Biosens. Bioelectron., 2014, 53, 283–287 CrossRef CAS PubMed.
- J. F. García-Reyes, P. Ortega-Barrales and A. Molina-Díaz, Anal. Sci., 2007, 23, 423–428 CrossRef.
- A. O. Santini, J. E. De Oliveira, H. R. Pezza and L. Pezza, J. Braz. Chem. Soc., 2006, 17, 785–791 CrossRef CAS.
- L. Guo, Y. Huang, Q. Zhang, C. Chen, D. Guo, Y. Chen and Y. Fu, J. Electrochem. Soc., 2014, 161, B70–B74 CrossRef CAS.
Footnote |
† Electronic supplementary information (ESI) available. See DOI: 10.1039/c5ra21489k |
|
This journal is © The Royal Society of Chemistry 2016 |
Click here to see how this site uses Cookies. View our privacy policy here.